- 1Institute for Ecosystems and Sustainability Research, National Autonomous University of Mexico, Morelia, Mexico
- 2Ecohydrology and Landscape Evaluation, Technische Universität Berlin, Berlin, Germany
In the tropics, livestock grazing usually occurs simultaneously with charcoal production, yet empirical understanding of the combined activities remains poor, especially in terms of their effects on hydrological functions. Given predicted growth in both charcoal and beef production in Sub-Sahara Africa, South East Asia, and Central and South America, understanding the potential effects of maintaining this dual production system on local and landscape level hydrological dynamics is paramount for ensuring long-term ecosystem sustainability. Based on a synthesis of existing literature, we propose a theoretical and conceptual framework for analyzing the interlinks between charcoal, livestock, and hydrological processes where they co-exist. As a silo approach, we first analyze the isolated effects of charcoal production and livestock on hydrological processes before exploring their combined effects (systemic approach). Given the scarcity of studies that explicitly address the influence of traditional small-scale charcoal production on hydrological processes, we base our findings on existing knowledge about deforestation, forest fire and grazing impacts on hydrology. We find that exclusion of the effects of companion activities and omission of information on the intensity of biomass harvesting (i.e., pruning branches, selective harvest, clear cutting, uprooting tree stumps) can lead to over-attributing changes in hydrological processes to charcoal, thus exaggerating the effects on ecosystems which might lead to inappropriate interventions. We also find that, in the case of livestock keeping, impacts on hydrological processes are highly dependent on grazing intensity, with low intensity grazing possibly having negligible or even positive effects on forest regrowth and thereby restoration of hydrological processes. Thus, the charcoal-livestock-water nexus may have a wide range of outcomes for hydrological processes from negligible to highly profound effects, depending on key decisions in management and practice. To test these findings, however, field studies are needed that explicitly treat the combined effects of different biomass harvesting practices and grazing intensities on hydrological processes across different scales. Albeit conceptual at this stage, we believe that our approach is a necessary first step in the process of diagnosing potential shortcomings of past approaches for studying charcoal production systems and developing new understanding of this three-way nexus.
Introduction
One third (2.4 billion) of global population depends on traditional woodfuels (charcoal and firewood) for most of their cooking and heating requirements (FAO, 2017). For 29 countries primarily in sub-Saharan Africa (SSA), woodfuel constitutes more than 50% of total national energy supply (FAO, 2014). It is estimated that worldwide, approximately half of the wood extracted from forests is used as woodfuel, 17% of which is converted to charcoal (FAO, 2017). Forests are central for regulating local, regional, and global hydrological cycles (Bradshaw et al., 2007). In woodfuel-dependent nations, over-extraction of woody biomass to supply the energy sector can jeopardize the status of forests and their ability to fulfill their regulatory functions (Bazilian et al., 2011).
The water-energy-food (WEF) nexus is usually presented as the point of interaction of the three resources with a tradeoff often implied between two or all components (Bazilian et al., 2011; Hoff, 2011; Endo et al., 2017). Typically, the energy component depicted in descriptions of this nexus is electrical, sourced either from hydropower, nuclear, petroleum or from biofuels derived from crops. The obvious tradeoff, in these examples, tends to be about securing sufficient water for energy production when it is also needed to produce food. In the case of traditional charcoal production, as is conducted by millions of small-scale producers in SSA, Central and South America, the Caribbean and South East Asia, natural forests and shrublands supply most of the biomass (FAO, 2017). In this context, the water-energy link is ecohydrological with the central question being: Does charcoal production negatively impact local and regional hydrological dynamics?
Most tropical landscapes are managed for multiple co-occurring activities (DeFries and Rosenzweig, 2010) whose combined effects on system-level functioning are insufficiently understood (Uriarte et al., 2011). Charcoal producing landscapes are no exception. Cattle, goats, sheep, donkeys and horses are often released in the forests and woodlands where charcoal is made to graze and forage on grass, shrubs, trees, and coppicing stumps. Livestock grazing in the form of pastoral, agro-pastoral and silvopastoral systems supplies 24% of global meat production; 50% of global beef is produced in developing countries under such grazing systems. Nevertheless, a recent global review of the current state of research of the WEF nexus identified 37 projects that addressed two or more nexus components (Endo et al., 2017). Of these, 23 addressed energy and food; none, however, addressed traditional woodfuel (charcoal and firewood) production systems. In the same review, 25 research projects addressed the combination of food and water (with and without energy), but only one addressed meat and dairy production as the food component, with the focus being on crop production for livestock. Global demand for livestock is expected to increase substantially in the coming decades (Herrero et al., 2009) yet, grazing is virtually absent from nexus research.
Multiple interaction effects invoked by co-occurring activities may be imperceptible if each activity is studies in isolation (Hoff, 2011). We are not aware of published studies that have specifically looked at the ecohydrological effects of charcoal production. Studies do exist, however, on the effects of grazing in forests on hydrological functions. Along with studies on the effects of deforestation, forest degradation, and wildfires on watershed function, they lay the groundwork for developing a conceptual understanding of how charcoal production and livestock might affect site- to watershed-level hydrological processes and functions. To do this, we first describe the charcoal production and the extensive livestock system individually. We follow this with an analysis of how each land use activity—in isolation—influences hydrological processes (the silo approach), before we explore the effects of their co-occurrence (systemic approach). Albeit conceptual at this stage, we believe that our approach is a necessary first step in the process of developing systemic understanding of charcoal production systems that takes into account co-occurring activities. It is also useful in identifying information and knowledge gaps for addressing integrated landscape management challenges in the tropics. By illustrating the added value gained from exploring system-level interaction dynamics, we hope that our paper encourages other researchers and practitioners to conduct similar exercises in their own study areas.
Charcoal Production
Despite transitions to cleaner and more fuels such as gas and electricity, charcoal is still a highly significant source of energy for many urban and peri-urban households in sub-Saharan Africa (SSA), South-east Asia and Latin America (Picos and Valero, 2009; FAO, 2017). Affordability and cultural preference for charcoal, compounded by high rates of population growth and urbanization in these regions suggest that, for the next three to five decades, demand will continue to grow before it begins to drop (FAO, 2017; Santos et al., 2017). Since charcoal is mostly sourced from natural forests (Chidumayo and Gumbo, 2013; FAO, 2017), meeting this growing demand is already posing challenges for the energy, forestry, and environmental sectors in the tropics.
Charcoal production can have severe consequences for ecosystems and ecological processes. Biomass removal and biomass burning can result in habitat depletion and associated biodiversity loss (Ferraro et al., 2011; Fontodji et al., 2011; Sodhi et al., 2011; Specht et al., 2015), contributing to greenhouse gas (GHG) emissions (Pennise et al., 2001; Bailis, 2009; Bailis et al., 2015) and reduction of carbon storage and sequestration capacity of terrestrial forest biomes (Mwampamba, 2007; Chidumayo and Gumbo, 2013). At the kiln site, where the biomass is converted to charcoal, long-term and seemingly non-reversable effects on soil biodiversity and soil physicochemical properties have been reported (Oguntunde et al., 2008; Fontodji et al., 2009; Nigussie and Kissi, 2011). These and earlier studies on charcoal contributed to setting the tone of international policies and interventions for tropical forests, and national forest and energy policies: that charcoal production is environmentally unsustainable; it should be curtailed; cleaner fuels must be sought (Mwampamba et al., 2013; Zulu and Richardson, 2013; Doggart and Meshack, 2017).
In the past decade, increased accessibility and quality of satellite imagery have drastically improved current understanding of charcoal production systems, prompting renewed interest in assessing the broader effects of charcoal production on forests and ecosystems (Ahrends et al., 2010; Ghilardi et al., 2016; Sedano et al., 2016; Bailis et al., 2017). Importantly, newer studies distinguish two dominant pathways through which charcoal in the tropics is produced. In the first pathway, forests are managed specifically to produce charcoal, making it the primary objective of the management system. Under this pathway, felled areas are left to regenerate, and a rotational cycle is maintained, albeit a seemingly arbitrary cycle in cases where informal forest management prevails (FAO, 2017). In the second pathway, trees are harvested for other land use objectives such as to produce timber or to clear new land for agriculture. In this pathway, charcoal making is a by-product that makes use of woody residues unsuitable for timber or fallen and uprooted trees that hinder agricultural activities. While forest degradation (in terms of species richness, genetic and structural diversity, and biomass density) tends to be the most likely outcome in the first pathway, complete forest loss (deforestation) is more probable in the second.
Seldom do studies on charcoal production specify which of these two pathways prevails in their study areas, making it difficult to discern which process is more prominent globally and regionally. Nevertheless, attributing forest degradation rather than deforestation to charcoal production has probably contributed to fundamental shifts in attitudes toward charcoal (and woodfuels) by policymakers and the donor community. This is especially that case in SSA, where there has been a visible upsurge in recent years in government and donor funded interventions aimed at producing charcoal more sustainably, a complete turnaround from previous prohibition and elimination interventions. Examples include the Swiss government support for a charcoal project in Tanzania (2014), German government support to wood charcoal industry in Namibia (2017), FAO's publication of how to green the charcoal value chain (FAO, 2017), and The Nature Conservancy's request for a study on the economics, policy and investment opportunities for sustainable charcoal in East Africa (2017). However, due to shortcomings in existing research, these new projects—which will apply state of the art knowledge of charcoal production systems—could encounter critical implementation challenges.
As research approaches have become more systemic and interdisciplinary, it is becoming increasingly obvious that charcoal production studies have made two important omissions about production systems in the tropics. First, that charcoal is rarely the only activity occurring on the land on which it is produced. Anecdotal evidence from researchers and practitioners working in charcoal production systems often cite timber harvesting, grazing, hunting, and collection of medicinal plants, wild foods and firewood as typical activities co-occurring in the same physical space and at the same time as charcoal is being produced (Eckholm et al., 1984; Maass et al., 2005; Mwampamba, 2009; Randriamalala et al., 2016; Woollen et al., 2016; Castillo-Hernández, 2017). Few of these studies, however, explicitly quantify the effects of these co-occurring activities on ecosystems. This makes the specific impact of charcoal making on forests and forest processes a challenge to single out and easy to over or underestimate. Secondly, the forests and woodlands from which charcoal is derived have a regulating role in local and regional hydrological dynamics in addition to the carbon cycle regulation that is more often emphasized.
Where Charcoal Meets Livestock
Among companion activities, livestock keeping is probably the most common, occurring virtually everywhere that charcoal is produced, from goat grazing in Madagascar (Randriamalala et al., 2016) to cattle in Kenya (Owen, 2013), Ethiopia (Gezahegn, 2018), Mexico (Castillo-Hernández, 2017), and Argentina (Abril and Bucher, 1999; Clark et al., 2010). In fact, some landowners in the tropics consider grazing an ideal complementary activity for charcoal production and vice versa. They actively prune (Owen, 2013) or selectively cut (Castillo-Hernández, 2017) trees to maintain desirable levels of pasture while converting the resultant biomass into charcoal. Alternatively, they incorporate livestock into forests and woodlands to keep grasses low to prevent the spread of wildfires (Castillo-Hernández, 2017). Due to complex process interactions it is likely that charcoal and livestock production co-occurring in an area will affect hydrological dynamics distinctly different than if each activity were occurring separately. At the very least, current understanding of charcoal production effects on vegetation and soils in the tropics would need to be reassessed in light of the possible effects that livestock may also be having on the system. Similarly, studies of livestock impact on vegetation that omitted the co-occurrence of charcoal in management systems would probably have misinterpreted the effects. Failure to address some of these inherent complexities of charcoal production systems may partially explain a history of frustrations with policy interventions in the sector, particularly in SSA (Mwampamba et al., 2013; Zulu and Richardson, 2013; Doggart and Meshack, 2017).
Studies that explicitly recognize land use activities that co-occur with charcoal making or which document, measure, and explore their singular and combined effects are few and only just emerging (e.g., Randriamalala et al., 2016; Woollen et al., 2016; Castillo-Hernández, 2017). Where companion activities exist, pinpointing the singular effects of charcoal is complicated by the fact that other activities exert their own effects on ecosystems by interacting directly with the vegetation and with the substrate on which vegetation depends. Livestock, for example, could affect woody biomass supply for charcoal by enhancing or inhibiting biomass recovery processes, such as natural regeneration. A silo approach to understanding the effects of livestock or charcoal ignores the multiple interactions taking place between vegetation and livestock, between livestock and charcoal production, and between charcoal production and vegetation. It also excludes the effects of these interactions on other higher-level ecological processes such as regulation of the carbon and hydrological cycles.
Incorporating companion activities to the charcoal production system drastically changes how charcoal effects are understood and how charcoal as a “problem” can be addressed through policy and practice. Indeed, doing so changes the charcoal issue from a seemingly tame or relatively simple problem to an “extraordinarily complex” or “wicked” one (Lach et al., 2005). Or, one that has “multiple and conflicting criteria for defining solutions,” whose “solutions create problems for others,” and where “no rules exist for determining when problems can be said to be solved” (Rittel and Webber, 1973). Given the rise in recent years of meat production in the tropics to meet global shifts toward higher meat consumption (McAlpine et al., 2009; Henchion et al., 2014; Lobato et al., 2014), where livestock keeping coincides with charcoal, an integrated understanding of their combined effects would ensure that adequate policies and practices are developed.
The Water Dimension
Known feedbacks exist between vegetation and hydrological processes (Ludwig et al., 2005; Dekker et al., 2007; Asbjornsen et al., 2011) that are affected by biomass removal, a fundamental feature in charcoal production. The absence of hydrological studies in charcoal production systems is particularly relevant and oddly surprising given the tendency for charcoal making to occur in water-limited ecosystems. Tropical dry forests and woodlands (e.g., Miombo and Mopane woodlands in SSA and the sahel woodlands of W Africa), tropical savannas (e.g., the cerrado ecosystems of Brazil), and tropical shrublands (e.g., chacos in Argentina, matorral in Mexico) provide most of the biomass used to produce charcoal (FAO, 2017). If charcoal production affects hydrological processes, compounded effects would be expected in these water-stressed ecosystems where changes in hydrological processes could have cascading and disproportional effects on overall system resilience.
The Charcoal-Livestock—Water-Nexus
Omitting these two attributes of typical tropical charcoal production, i.e., the co-occurrence with companion activities and the feedbacks with hydrology, systems puts “sustainable” charcoal projects at risk of being fundamentally flawed in terms of their ecological understanding of system-wide responses to charcoal production. Additionally, implementation barriers may arise due to conflicts between land users who have not been adequately acknowledged.
Thus, the confluence of charcoal, livestock keeping, and hydrological processes presents an intriguing and unexplored nexus that urgently needs unraveling. Here, we provide a theoretical and conceptual framework for analyzing the interlinks between charcoal, livestock, and hydrological processes where they co-occur.
Theoretical Underpinning of Interactions Between Vegetation and Hydrological Processes
There is long-term recognition by both hydrologists and ecologists of the importance of vegetation (and forests in particular) in modulating key hydrological processes (Asbjornsen et al., 2011; Henchion et al., 2014). From reducing the direct impact of precipitation on bare surfaces to determining infiltration into soils, vegetation (along with climatic, geographical and geological factors) determines the amount of water available in the landscape to replenish surface and underground reservoirs (Ludwig et al., 2005). Nevertheless, studies that explore interlinks between vegetation and hydrology in charcoal production systems are virtually nonexistent.
Four stages in the charcoal production cycle are likely to be the most relevant for hydrological processes (Figure 1). These stages represent key changes in vegetation and soil properties that, given what is understood of vegetation-water dynamics, should influence hydrological processes. The mature forest phase consisting of trees forming a closed or partially closed canopy represents the natural forest type in the area and its main characteristics in terms of tree size and shape, tree density, and tree species composition. Depending on forest type, a mix of sapling trees, shrubs, herbs, and grass would be expected in the understory vegetation.
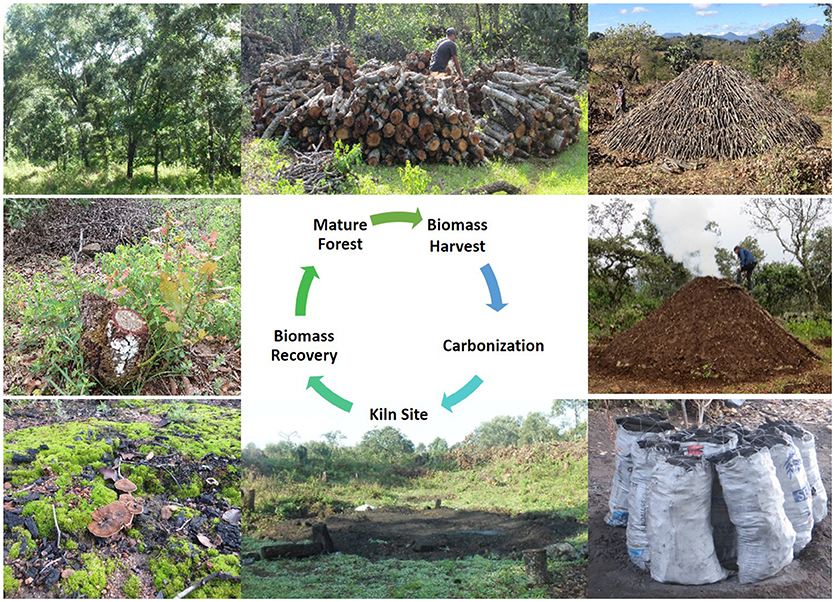
Figure 1. Key stages in a typical traditional system in which forests or woodland are managed for (among other things) charcoal production. Clockwise from upper left corner: Wood is harvested from mature forests, different sized logs are stacked above or below-ground to form a rectangular or conical kiln that is insulated with locally available material such as, grass and earth. Once lit, the process of carbonization converts the wood to charcoal which is subsequently packed into sacks that are sold to intermediaries. With time (>20 years), the kiln site recovers some vegetation. In felled areas, tree stumps coppice in 2–4 weeks to initiate the biomass recovery period which lasts from 8 to 30 years before the cycle is repeated. Livestock are typically an integral part of this system, present in all or only some of the stages. Photos courtesy of THM.
The biomass harvesting phase captures key elements about how the vegetation is removed, specifically, whether biomass is obtained from felling entire trees, pruning branches, or from thinning sprouts and suckers. It also outlines whether clear felling (i.e., all trees are felled regardless of size and/or species) or selective harvesting (only specific species of specific size are felled) is practiced. In most forests or woodlands managed for charcoal production, 50–90% of standing trees are felled (Chidumayo, 1991; Chidumayo and Gumbo, 2013). Removing >10% of forest cover is technically deforestation (FAO, 2010). The biomass harvesting stage also clarifies whether uprooting is involved. As outlined earlier, uprooting would indicate that other land use motives are at play (e.g., agricultural expansion) in which charcoal making would be a by-product (pathway one).
The biomass recovery phase is a period lasting 9–30 years (FAO, 2017) and is generally characterized by resprouting (or coppicing) of tree stumps and gradual recovery of aboveground vegetation toward mature forest status. Coppicing is a widely recognized functional trait of tropical forest tree species and the primary mechanism through which they regenerate (Murphy and Lugo, 1986; McLaren and McDonald, 2003). It is also a key shared worldwide characteristic of trees preferred for charcoal making (Chidumayo and Gumbo, 2013; FAO, 2017).
Finally, a parallel recovery process takes place on abandoned kiln sites. Kiln sites are areas where biomass was stacked, insulated, and ignited to convert it to charcoal. The site represents an area that has experienced extreme heat (>400°C) for 5–21 days and sometimes more (FAO, 2017). It can take on a rectangular or circular shape typically occupying a surface area of 20–100 m2. In traditional charcoal production systems, the kiln site moves in response to biomass availability (FAO, 2017). Depending on numerous factors, among which are tree density of desired species, ability, and willingness to move logs to kiln site, and ease of finding ideal conditions for locating kilns, several (dozens, scores) kiln sites might be concentrated in a given area or dispersed across a larger surface. Generally, the total kiln site surface area tends to be about 5% of the total harvested area, but this varies considerably across forest types and other factors (Chidumayo and Gumbo, 2013). Some kiln sites are reused in subsequent harvest cycles (Voorhoeve, 2017), but more often than not, new sites are developed with each harvesting cycle.
For the purpose of our analysis, we grouped hydrological processes into four main groups based on the impact of processes on different components of the water balance (Figure 2): (i) aboveground rainfall distribution (interception, stemflow, and throughfall); (ii) distribution of infiltration and water storage in the soil (i.e., the water that remains in the local environment and is available for vegetation recovery); (iii) evapotranspiration (i.e., the sum of water that is released from the soil surface and vegetation into the atmosphere), and; (iv) runoff and percolation to groundwater (i.e., water which is lost from the topsoil and is no longer available for evapotranspiration). Granted, this is a gross simplification of the systems, since all these processes are highly interlinked and dependent on one another.
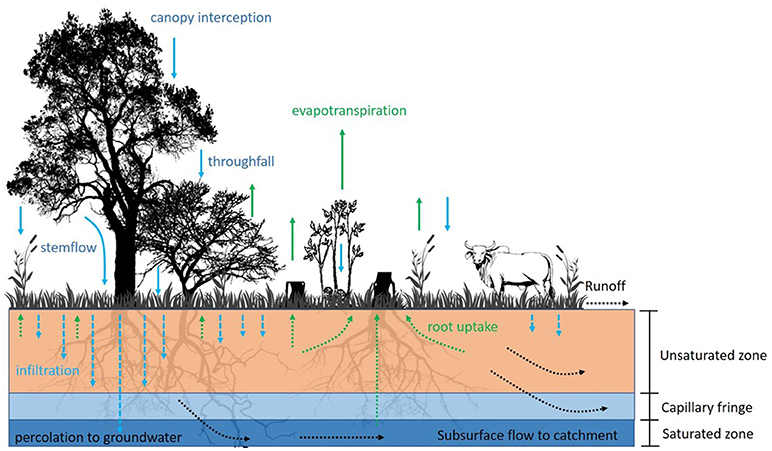
Figure 2. Hydrological processes most relevant for exploring the effects of charcoal production and livestock on hydrological functioning. Illustration by THM.
Silo Approach I: The Potential Effects of Charcoal Production on Hydrological Processes
Review of Hydrological Processes in a Mature Forest
In mature forests, trees affect how water (i.e., rainfall) is distributed to the forest floor, how it infiltrates into the soil, and how it moves as surface runoff or subsurface flow to catchment-scale discharge. Worldwide, the influence of forests on hydrological processes is variable because they are contingent on geological properties and river network topography on the one hand, and on forest type, forest age, and climatic conditions on the other (Zhang et al., 2001; Bonell and Bruijnzeel, 2005; Levia et al., 2011). In mature forests, the “double funneling effect” of trees plays a fundamental role for the distribution of rainfall to evaporation, infiltration, and surface runoff (Johnson and Lehmann, 2006). The first funneling effect refers to aboveground distribution of rainfall by tree canopies. Forest canopies can intercept a relatively large proportion of precipitation, 10–50% of season-long or annual rainfall (Carlyle-Moses and Gash, 2011). The remaining rainfall makes its way to the forest floor, either as throughfall or along stems and branches (Llorens and Domingo, 2007). The degree of funneling by trees depends strongly on the tree species (Llorens and Domingo, 2007; Návar, 2011), canopy architecture (Bialkowski and Buttle, 2015), stand density and forest age and can range from negligible funneling ratios for some species to extreme funneling ratios for others (Levia et al., 2010; Zou et al., 2015).
The second funneling effect refers to the impact of tree root systems on belowground distribution of water. In the vicinity of tree roots, enhanced soil fauna abundance and activity in the root system increases soil macroporosity (Lozano-Parra et al., 2016), which can lead to very high infiltration capacity near tree stems. Indeed, due to this second funneling effect, infiltration rates are observed to decrease exponentially with increasing distance from tree stems (Pressland, 1976). The high overall infiltration capacity of mature forests ensures that most of the rain that reaches the forest floor percolates into the soil, thus contributing to soil moisture and groundwater recharge (Návar, 2011) and making surface runoff or erosion highly unlikely (Bonell and Bruijnzeel, 2005; Farrick and Branfireun, 2014).
In forests, the water which is intercepted by the vegetation as well as a large part of the infiltrated water is used for evapotranspiration. Generally, evapotranspiration from forests is higher than from other vegetation types (Bosch and Hewlett, 1982) and this difference increases with increasing annual precipitation (Zhang et al., 2001). In dryland forests, such as the Miombo and sahel woodlands from which most charcoal in SSA originates, the absolute difference between evapotranspiration in forests and other vegetation types is not that high, however, in such water-limited ecosystems even small differences in the water balance are important (Zhang et al., 2017). Forests can also affect the temporal dynamics of evapotranspiration. Forest evapotranspiration can remain higher than that of shrubs or grasses during warm and dry spells because the forest root systems can obtain water from deeper soil layers (Lozano-Parra et al., 2016). Through this mechanism, forests can have a positive effect on the feedback between soil moisture and precipitation, even during warm and dry spells (Bonan, 2008).
Catchment-scale discharge from mature forests is usually produced mainly by subsurface runoff to streams (rather than surface runoff, see Farrick and Branfireun, 2014), either through rapid subsurface stormflow or slower groundwater flow (Dias et al., 2015). At the watershed scale, this ensures slower and more continuous discharge from forested areas, a stark contrast to the fast discharge with larger amplitudes associated with croplands (Dias et al., 2015). It is through this ability to regulate flow that forests can provide important flood protection during heavy local rainfall events in summertime (Bradshaw et al., 2007).
Effects of Biomass Harvest on Hydrological Processes
In the following paragraphs we summarize the effects of deforestation on hydrological dynamics. As mentioned in the introduction this is one prevalent pathway for producing charcoal in parts of the tropics, including in Brazil (Swami et al., 2009) and parts of Tanzania (Beukering et al., 2007), where the primary motivation however is agricultural expansion. Deforestation studies can be misleading if applied to the first pathway of charcoal production in which other biomass harvesting methods are used and tree stumps are left intact to regenerate. In the absence of research that has investigated the hydrological effects of biomass harvesting methods in forests or woodland maintained for charcoal making (by pruning branches, selective felling or clear cutting), we can only hypothesize that significant discrepancies can be expected in the influence of the different harvesting methods on the hydrological processes. Thus, we provide a description of how hydrological processes might vary theoretically if charcoal were bring produced under the different pathways.
Whether clearcut or selective harvest is undertaken, the biggest impact that tree removal has is to alter rainfall distribution to the different water balance components through the removal of the double funneling effect of trees. Due to vegetation removal, rainfall is distributed homogeneously on the soil surface and lands on the soil surface with more force (Levia and Frost, 2003), which may increase the susceptibility of soil to erosion. Additionally, vegetation removal changes the infiltration capacity of soils, which in turn influences water distribution to infiltration or runoff processes (Johnson and Lehmann, 2006). When biomass removal occurs, and especially when it includes uprooting of trees, the soil structure—including its macroporosity—is destroyed, resulting in increased surface runoff. Destruction of the macroporosity effect, in fact, explains the oft-reported erosion effects of deforestation (Bosch and Hewlett, 1982; Bruijnzeel, 2004). Under a scenario of pathway one charcoal production, the first funneling effect of vegetation removal could be minimal (in low intensity pruning) or very similar to that of deforestation (in clear cut). But because roots are maintained in pathway one production, the soil structure per se remains undisturbed even if the direct impact of raindrops intensifies. The second funneling effect, therefore, might be minimally affected (and could even stay intact, in some cases) compared to the deforestation scenario, thus only minimally influencing the mean infiltration.
Apart from changes in mean annual runoff sums, runoff dynamics also change to much faster flow rates during and shortly after rainfall events after vegetation removal (Ward et al., 2007). This is most evident in clearcutting for agriculture. Conversion of forest to soy bean agriculture, for example, resulted in a strong increase in both the total discharge and the amplitude in discharge (Dias et al., 2015), meaning more extreme high and low flows. Similarly, a review on the effects of deforestation on catchment scale discharge in East Africa found that total discharge increased as did the peaks of discharge; however decrease in low flows was only significant in 31–35% of the studied catchments (Guzha et al., 2018). Under pathway one production, changes to runoff dynamics would be substantially lower not only due to maintenance of intact root systems, but also because the litter layer and undergrowth vegetation generally remains intact (albeit trampled) and would buffer soils from the intensified power of raindrops arriving on the soil surface. The exceptions could be on steep slopes or with recent fire events that removed the buffer effect of undergrowth vegetation and litter.
In addition to infiltration, evapotranspiration volumes and dynamics can also be (temporarily) affected by tree removal for charcoal production. Trees draw water from deeper layers than shrubs and grasses do, which generally contain more shallow roots and have limited access to deeper sources of soil moisture in dry months (Lozano-Parra et al., 2016). Consequently, tree transpiration can continue for a longer period than transpiration of undergrowth vegetation. In addition to more homogeneous rainfall distribution due to vegetation removal, the absence of branches and leaves to intercept the rain removes shade. Its removal would enhance undergrowth, intensify undergrowth evapotranspiration of the undergrowth and quicken the drying of the top soil layer. Thus, biomass harvesting not only results in an absolute change in the water balance components, it mainly also affects the temporal dynamics of hydrological processes. Depending on the scale of land use change in the area, this local vegetation-soil moisture interaction may lead to a macro scale feedback (Dekker et al., 2007; Seneviratne and Stöckli, 2008), whereby large-scale deforestation influences temperature and precipitation regimes of the region (Chambers and Artaxo, 2017), small scale changes however are too small to influence rainfall patterns (D'Almeida et al., 2007). Due to the complex nature of forest-atmosphere feedbacks and the influence of patch size, a general conclusion on the influence of forest removal on local climate conditions cannot be made (Bonan, 2008; Chambers and Artaxo, 2017).
Thinning and selective logging of up to 70% has been shown to have a modest effect on rainfall partitioning and an even smaller effect on soil water and streamflow (probably due to the increased vigor of remaining vegetation) (Bruijnzeel, 2006). Hence, as long as the biomass harvesting method ensures that the soil structure remains intact for the most part, the direct influence of biomass harvesting for charcoal on infiltration is expected to be negligible. This example highlights the importance of studying the specific effects of different biomass harvesting procedures on hydrological processes. In a deforestation scenario, we can deduce from the literature, that infiltration and evapotranspiration strongly decrease, and surface runoff increases after deforestation (Bosch and Hewlett, 1982), especially if there is a complete change of land use to agriculture (crop) or pasture (Alegre and Cassel, 1996).
Hydrological Processes During Biomass Recovery
In a scenario in which forests are cleared for agriculture at large scales, the resulting changes in temporal dynamics of evapotranspiration and runoff as well as influence on the climate would remain. Under pathway one production, which is generally small scale and temporary, with rapid regrowth of the coppices, the influence of harvesting on the yearly sums as well as temporal dynamics of the different water balance components might be minimal and rapidly restore to pre-harvesting values.
Biomass recovery begins immediately after biomass harvest and lasts 9–30 years (FAO, 2017). During this period, infiltration, runoff and evapotranspiration can recover to their initial states (Hassler et al., 2011), although the time to recovery may vary depending on the severity of deforestation, the type of alternative land use (Colón and Lugo, 2006), soil properties (D'Almeida et al., 2007; Hassler et al., 2011), and local climatic conditions (Aide et al., 1996; Chazdon, 2003). After a clear-cut, fine scale biological responses in roots are key to determining vegetation recovery. The fine-root biomass of recovering trunks, for example, undergo three key phases during recovery: a rapid increase up to a maximum of fine-root biomass; a decrease during maturation of the stand; and a steady-state in mature stands (Claus and George, 2005). The hydrological implications of this is that water use by vegetation changes dramatically with stand age (Vertessy et al., 2001). Resprouting tree trunks demand the most water in the early stages of vegetation recovery and during forest maturation, but this demand decreases and then stays relatively steady in mature stands (Figure 3). Importantly, water that is taken up undergoes evapotranspiration and is unavailable for discharge from the catchment.
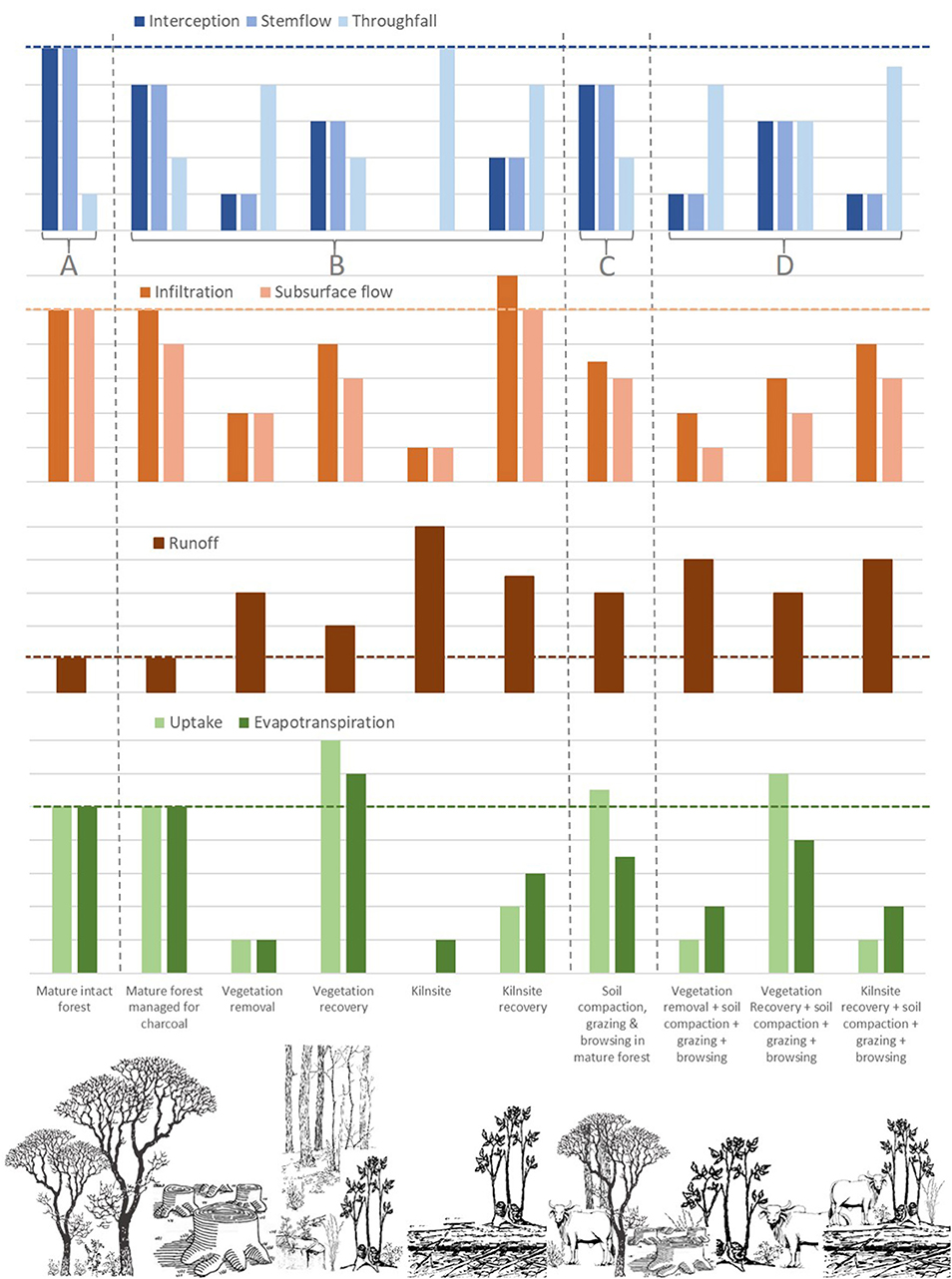
Figure 3. Hypothetical comparison of how key hydrological processes would change relative to (A) mature intact forests (far left bars), (B) during each stage of the production cycle in forests managed solely for charcoal (next five bars), (C) in forests managed only for livestock, and (D) in forests managed for both charcoal and livestock. Changes in hydrological processes are assessed relative to levels in mature forests (horizontal dotted line) and not relative to each other. Grazing intensity is assumed to be medium to high.
As the vegetation changes from stumps to bushy shrubs to high canopy trees, raindrop impact on soil surfaces becomes less forceful with increasing vegetation cover and rainfall distribution to surface layers diversifies to once again include stemflow and throughfall (Figure 2). A decrease in surface temperature and increase in humidity under the canopy due to increased shade from regrowth reduces direct soil water evaporation (Negrete-Yankelevich et al., 2007). Consequently, soil moisture patterns feedback to affect vegetation dynamics through their effects on plant establishment and growth (Breshears and Barnes, 1999), competitive interactions and successional processes (Booth et al., 2003; Asbjornsen et al., 2004).
Hydrological Dynamics at Kiln Sites and Surrounding Area
Studies of the influence of fire on hydrological processes may help deduce what occurs at kiln sites. After fires, runoff and erosion increase strongly, but then slowly decrease in the following years if revegetation of sites occurs (Cerdá and Doerr, 2005). The increase in runoff and erosion is not only due to vegetation removal, but also often attributed to a hydrophobicity of the top soil caused by the fire (Doerr et al., 2000). Additionally, under severe fires (such as can be expected at kiln sites) the soil structure (macroporosity) is destroyed as is the soil fauna, which is responsible for building a large part of soil structure (Certini, 2005). Whether or not soil infiltration recovers and runoff and soil erosion decrease to pre-fire states depends mainly on whether revegetation happens at kiln sites (Cerdá and Doerr, 2005). Revegetation of kiln sites, however, is an understudied phenomenon, with contradicting findings. While some studies report little to no vegetation recovery of abandoned kiln sites (Boutette and Karch, 1984; Chidumayo and Gumbo, 2013), others show that revegetation occurs, although sites have distinct characteristics (soil properties, species composition) from surrounding areas, even hundreds of years after abandonment), at least in temperate regions (Nelle, 2003; Carrari et al., 2016).
After vegetation regrowth, infiltration capacity may recover rapidly and surpass that of pre-burn levels. Studies conducted specifically on the impact of charcoal kilns on soil properties often report soils under kilns to have lower bulk density, higher porosity and higher saturated conductivity (Oguntunde et al., 2008; Nigussie and Kissi, 2011; Wahabu et al., 2015). This higher conductivity contradicts the decreased infiltration (and therefore increased runoff) mentioned earlier and reported by studies on natural forest fires. It is possible that the effects on hydrology, such as water repellency, which is detected in post-fire landscapes diminishes over time. As soil structure is reconstructed and even improved, compared to pre-fire conditions, this can be followed by soil development in the opposite direction (high porosity). These mechanisms could help explain the apparent contradiction between the effects of fire and what has been recorded for kiln sites. Improved soil conditions at kiln sites is an oft-reported phenomenon associated with increased biodiversity (Carrari et al., 2016) and more lush vegetation on long abandoned kiln sites (Glaser et al., 2002; Oguntunde et al., 2004).
Kiln sites are often discussed as the area where biomass was burned, but they do, in fact, represent a larger impacted surface area. Removal of trees, undergrowth and grass to develop the kiln site exposes soils. In sloped areas, sometimes the ground is leveled by cutting into the slope. Additionally, the earth used to insulate the kiln is obtained from the periphery of the cleared site or at close proximity (< 15 m). This generates ditches and piles of lose soil. These practices imply that the most notable impact of kilns on hydrological processes in probably through the redistribution of rainfall to the soil surface. The bare ground becomes susceptible to the direct impact of raindrops, which increases runoff and exposes soils to erosion effects (Chidumayo and Gumbo, 2013). Runoff at kiln sites is exacerbated by the added fact that, in the area surrounding the kilns, the cleared woodland also modifies rainfall partitioning as described above (Levia and Frost, 2003) affecting water infiltration into the soil (Hamilton and King, 1983) and thereby, modifying evapotranspiration and runoff more generally (Chidumayo and Gumbo, 2013).
The ditches and gaping holes from which soil was extracted to build the kiln are a kiln-related feature that is virtually, understudied. These holes are, in fact, shallow troughs in which water can accumulate after a rainfall event to percolate into surface layers and affect vegetation dynamics downslope. Whether effective or not, ditches (200 cm long × 50 cm wide × 50 cm deep) are often intentionally dug out in forest restoration sites in Mexico with the belief that they conserve soils and improve soil moisture (SAGARPA, 2009; Perevochtchikova et al., 2012). Research is needed to understand the significance of kiln-derived ditches to ecohydrological processes in charcoal production systems.
Silo Approach II: The Effects of Livestock on Hydrological Processes
Cattle can be important agents of geomorphological change (Trimble and Mendel, 1995). Livestock grazing impacts forests in two principal ways: by altering vegetation cover and through the mechanical action of their hooves which is compounded by the weight of the animals (Mwendera and Mohamed Saleem, 1997; Blanco-Sepúlveda and Nieuwenhuyse, 2011). Studies linking the impact of grazing on hydrological processes have focused primarily on the latter, and specifically on the soil compaction effects on infiltration rates and surface runoff. Research has shown that, generally, soil compaction increases soil bulk density and decreases porosity which, in turn, decreases water infiltration rates and, subsequently, increases surface runoff (Rauzi and Hanson, 1966; Hanson et al., 1970; Gifford and Hawkins, 1978; Mwendera and Mohamed Saleem, 1997). Although these processes can affect site-level nutrient cycles, soil moisture patterns, erosion and sediment yields, downstream water quality, and on-site productivity (Gifford and Hawkins, 1978), for the most part, they have been assumed rather than measured directly.
The impact of herbivory and its subsequent effects on other hydrological processes beyond those of infiltration and erosion is understudied. We know even less about the effects of browsing (foraging on branches and understory shrubs) than those caused by grazing (consumption of grasses and herbaceous plants). We can deduce that removal or reduction of herbaceous vegetation would expose forest soils to the impact of rain events, increase erosion, reduce the horizon layer and if considered in light of compaction effects, would make it more difficult for seeds and saplings to establish. Low-intensity grazing and browsing, however, avoid many of these impacts (Randriamalala et al., 2016) and rather, maintain vegetation at a constant state of recuperation, which on the other hand, may increase water uptake.
Agro-silvo-pastoral systems can be harmonious and sustainable if the pressure of the different components are kept in a good balance, as is the case for the Meditarranean “Dehesa” landscapes (Schnabel et al., 2009). Surpassing sustainable grazing intensity levels decreases the recovery rate of trees and leads to landscape degradation (Schnabel et al., 2009). Intensive grazing and overgrazing have also been shown to cause irreversible conversion of forests to shrubland (Murphy and Lugo, 1986; Eliason and Allen, 1997; Trejo and Dirzo, 2000) and desertification (Geist and Lambin, 2004). Shrublands imply a drastic change in plant morphology from that of forests, which also leads to a change in volume and fluctuations in evapotranspiration (Sun et al., 2016). How this goes on to affect other hydrological processes remains unclear.
The Systemic Approach: A Three-Way Nexus Between Charcoal, Livestock, and Water
The systemic approach requires considering the effects on hydrological processes when charcoal production co-occurs with livestock keeping. To do so consists of a three-step process: understanding charcoal-livestock dynamics and its effects on soils and vegetation; overlaying those effects on the hydrological system, and finally, considering how hydrological changes influence charcoal and livestock processes. Charcoal-livestock dynamics would exacerbate some hydrological processes or inhibit them from occurring.
A woodland exposed to charcoal and livestock simultaneously undergoes removal of forest canopy for charcoal, soil compaction from the weight of cattle, destruction of the soil structure from the direct impact of hooves, and vegetation suppression or over-compensation from browsing and grazing by cattle. These processes have the largest effect on infiltration rates, which decrease with biomass removal for charcoal production, but this drop is exacerbated if soils (in the mature forest) have experienced the treading stress of livestock or if livestock are introduced immediately after biomass harvest (Arevalo et al., 1998; Godsey and Elsenbeer, 2002). Consequently, the co-occurrence of charcoal production and grazing increases surface runoff, and thereby, erosion. The repercussion of this is that areas where both activities occur could have less water available for vegetation regrowth such that the growth spurts observed in the early phases of recovery in charcoal-only systems are suppressed or severely hampered. Compared to livestock-only systems in which trees are maintained, most of the rainfall distribution attributes of mature forest would be conserved making the effects of compaction less severe.
The direct effects of grazing and browsing on resprouting tree stumps probably affects vegetation recovery rates and intensity, and through this, evapotranspiration, subsurface runoff, and catchment discharge. Browsing of coppicing tree trunks may keep sprouts from growing beyond a certain size while over-browsing could altogether stunt the recovery process (Plieninger et al., 2011). Alternatively, herbivory may cause vegetation to compensate with intense growth which would increase water demand. The consistent regrowth of biomass would result in high evapotranspiration rates and the subsequent decrease and tapering off of evapotranspiration expected with forest maturity might not occur. The compacted soils in harvested areas and kiln sites might also make it difficult for seeds to establish (Pedraza and Williams-Linera, 2003) as soil erosion would have swept off the seedbank and the litter and the first horizon layers necessary for healthy seedling establishment and growth.
Discussion
Despite intuitive interlinks between forests and water, wood-based energy such as charcoal and firewood are underrepresented in discussions and research on the food-water-energy nexus. Similarly, livestock keeping—and in particular grazing in natural forests—is rarely depicted in the food component of the nexus. In tropical regions such as SSA, however, and in countries where large volumes of charcoal are produced, extensive livestock grazing tends to coincide spatiotemporally with charcoal production bringing novel dimensions to the nexus, primarily ecological in nature. The step by step hypothetical exploration we have conducted in this review of how different hydrological processes would play out in charcoal-livestock systems provides a preliminary analysis of the nexus. It facilitates identification of potential tradeoffs, synergies, and critical knowledge gaps.
We have brought together current understanding of the ecohydrological effects of deforestation, forest degradation and livestock to develop a preliminary cognitive model of the nexus highlighting its components, their interactions, and how these might vary under different management regimes. This conceptualization exercise serves several purposes. First it is used to identify and compare the influence of the two different pathways of charcoal production on hydrological processes: aboveground rainfall distribution, infiltration and soil water storage, evapotranspiration and, runoff and groundwater. It ecomes very clear here that the influence of the charcoal production where true deforestation takes place and land use changes to agriculture has a very large and permanent influence on the different hydrological processes, however in case of relatively small scale charcoal production with removal or thinning of coppices without uprooting trees, the influence on the hydrological processes is likely much smaller and with proper consideration of rapid regrowth potential, might be reduced to a negligible and only short lived local influence.
The second purpose that our conceptualization serves is to highlight the stages of the charcoal-livestock system that are key to understanding the nexus in terms of its hydrological effects. A typical charcoal value chain of the first pathway outlines seven to eight stages between the forest where biomass is sourced and the final charcoal consumer (FAO, 2017). In such a life cycle approach to understanding charcoal, the production stages are depicted as two main processes: sourcing of the biomass and carbonization. If the central question, however, is “How do charcoal and livestock, together, affect local and regional hydrology?” more detailed understanding of the production phase is required, necessitating finer subdivisions of the production process into four central processes: the forest before it is cut, the biomass harvesting stage, and the process of recovery of the stand where biomass was harvested, and of the kiln site where carbonization took place. This framing downplays carbonization and focuses instead on the effect it leaves behind at the kiln site. Consequently, we emphasize the need for explicit research programs which study the influence of the different production processes to appreciate their possible effects on ecohydrological dynamics. By doing so, we provide a simple and effective starting point for understanding this nexus and the interactions therein. Such understanding, albeit preliminary and conceptual at this stage, is necessary for flagging key issues of the charcoal-livestock system that could lead to undesired consequences for system-level dynamics. It also pinpoints knowledge gaps that are worthy of further research. These are discussed in more detail in the following paragraphs.
At first glance—and with only lay understanding of ecohydrology—it would seem evident that charcoal production and livestock keeping, whether occurring independently or conjointly should impact local hydrological processes negatively through their effect on vegetation and soil substrate. Our exercise, however, revealed that a range of impacts are possible, from negligible to highly profound, depending on several management characteristics of both land use activities. In the case of charcoal, the intensity of biomass harvesting is key: pruning of branches might have imperceptible effects to the hydrological system while selective harvesting and clear cutting could alter the system significantly. Our review suggests that as long as pruning and selective harvesting maintain 30% canopy cover or more, the effects on rainfall distribution and soil erosion can be minimal. For this to apply, however, livestock keeping would need to be maintained at low grazing intensities so that soils are unexposed. Indeed, the key management criteria for livestock keeping is grazing intensity. Studies repeatedly show that, whether it is goats or cattle, low grazing intensities avoid a cascade of effects on vegetation, hydrological processes and soil substrates.
The review process highlighted shortcomings in the charcoal research and literature that require immediate attention. Despite recognition of the two production pathways of charcoal, published studies on charcoal rarely describe with sufficient detail which of these pathways is prevalent in their study sites. This makes it a challenge to determine which biomass harvest strategy is practiced (deforestation with uprooting vs. pruning, selective harvest, and clearcutting with stem maintenance) and to deduce from existing studies how hydrological dynamics are affected by charcoal. Assigning a production pathway in the field or from remote sensing is challenging, however. A clear-cut forest in which all trees are used to produce charcoal would, in the short term, display most of the characteristics of deforestation for agriculture or other land use. To determine the motivations for forest clearing would require waiting 6 months to one year to see if tree stumps ultimately regenerate. Even so, observing regeneration after one year does not “proove” the original motivations for clear cutting since those could have changed with time. Furthermore, since livestock can be a temporary or permanent element of the system, it is possible to completely miss or overlook their role or to over-attribute their importance, respectively. Long-term field studies as outlined by Maass (2017) in this special issue, frequent field visits combined with time-series analysis of satellite imagery are needed to pinpoint production pathways and to outline the management practices at the level required for evaluation of hydrological implications.
Throughout our undertaking of this analysis we have mainly focused on local influences of charcoal production and livestock, trying to understand how processes occurring at the level of individual trees and surrounding soils scale up to affect site-level biophysicochemical interactions, and the implications of all this on catchment discharge. In case we assume a large homogeneous landscape in which all the land is dedicated to charcoal production, continuous biomass harvesting for charcoal would maintain a constant fraction of the patches with trees in a state of regrowth, consuming large volumes of water and never reaching the tapering off of evapotranspiration associated with mature forests (Vertessy et al., 2001). High demand for water by recovering vegetation might occur at the expense of catchment recharge. In a scenario whereby charcoal production co-occurs with high intensity grazing, grazing would exacerbate the effect on hydrological processes, through higher runoff (due to soil compaction by animal hooves), low soil water storage and low evapotranspiration implying a loss in the cooling effect of forests. It follows from this that, even if trees stumps are maintained and soil structure remains relatively undisturbed, a catchment used for charcoal production in co-occurrence with grazing could alter catchment level hydrological dynamics. The effects would be substantially more negative if livestock were incorporated at high intensity grazing.
Projected growth in demand for charcoal and meat indicate expansion or intensification of existing production systems in the tropics, including silvopastoral systems such as those analyzed in this review (Herrero et al., 2009; McAlpine et al., 2009; Henchion et al., 2014; Lobato et al., 2014). Specifically, for this system, we can expect biomass harvesting and grazing to intensify. In the case of charcoal, clear cutting becomes the dominant form of biomass harvesting as has already been observed when charcoal demand increases (e.g., Ahrends et al., 2010; Chidumayo and Gumbo, 2013). Rotational cycles might also be shortened implying that mature forest status is never attained. In terms of livestock, more cattle per unit area is expected and year-round stocking might become commonplace. This combination of factors might acerbate hydrological dynamics and push previously sustainable systems into unsustainable pathways. Addressing the research gaps that we have highlighted throughout this paper would help identify how to avoid costly tradeoffs.
Conclusions
Charcoal production and livestock keeping in the tropics have been demonized by poor understanding of how, precisely, they affect ecosystems and perhaps even wrongly attributing their effects on hydrological processes. There are several pressing environmental challenges facing society today where ecohydrology can contribute to scientific understanding of the complex interactions between multiple resources for developing sound management and policy solutions. By applying ecohydrological science to this three-way nexus, we have contributed to improving current understanding of how charcoal and livestock might—in isolation and conjointly—affect hydrological dynamics from local to catchment scale. We see that the degree to which charcoal production influences hydrological processes depends strongly on what kind of biomass harvesting method is used and on the scale of harvesting. We recognize that there are usually many other potential drivers and stressors that may also affect the charcoal-livestock-water dynamics.
Author Contributions
TM was involved with the development of the manuscript topic, literature review, writing and the illustrations. NvS contributed to development of the manuscript topic, especially of the hydrological processes, literature review and writing. LC conducted literature review.
Funding
This work has been possible due to funding from the National Autonomous University of Mexico's Research and Technology Innovation Support Program (PAPIIT), Projects No. IA201514 (2014–2015) and IA202216 (2016–2017).
Conflict of Interest Statement
The authors declare that the research was conducted in the absence of any commercial or financial relationships that could be construed as a potential conflict of interest.
References
Abril, A., and Bucher, E. H. (1999). The effects of overgrazing on soil microbial community and fertility in the Chaco dry savannas of Argentina. Appl. Soil Ecol. 12, 159–167. doi: 10.1016/S0929-1393(98)00162-0
Ahrends, A., Burgess, N. D., Milledge, S. A., Bulling, M. T., Fisher, B., Smart, J. C., et al. (2010). Predictable waves of sequential forest degradation and biodiversity loss spreading from an African city. Proc. Natl. Acad. Sci. U.S.A. 107, 14556–14561. doi: 10.1073/pnas.0914471107
Aide, T. M., Zimmerman, J. K., Rosario, M., and Marcano, H. (1996). Forest recovery in abandoned cattle pastures along an elevational gradient in Northeastern Puerto Rico. Biotropica 28, 537–548. doi: 10.2307/2389095
Alegre, J. C., and Cassel, D. K. (1996). Dynamics of soil physical properties under alternative systems to slash-and-burn. Agric. Ecosyst. Environ. 58, 39–48. doi: 10.1016/0167-8809(95)00654-0
Arevalo, L. A., Alegre, J. C., Bandy, D. E., and Szott, L. T. (1998). The effect of cattle grazing on soil physical and chemical properties in a silvopastoral system in the Peruvian Amazon. Agroforest Syst. 40, 109–124. doi: 10.1023/A:1006075114659
Asbjornsen, H., Goldsmith, G. R., Alvarado-Barrientos, M. S., Rebel, K., Van Osch, F. P., Rietkerk, M., et al. (2011). Ecohydrological advances and applications in plant-water relations research: A review. J. Plant Ecol. 4, 3–22. doi: 10.1093/jpe/rtr005
Asbjornsen, H., Vogt, K. A., and Ashton, M. S. (2004). Synergistic responses of oak, pine and shrub seedlings to edge environments and drought in a fragmented tropical highland oak forest, Oaxaca, Mexico. For. Ecol. Manage. 192, 313–334. doi: 10.1016/j.foreco.2004.01.035
Bailis, R. (2009). Modeling climate change mitigation from alternative methods of charcoal production in Kenya. Biomass Bioenergy 33, 1491–1502. doi: 10.1016/j.biombioe.2009.07.001
Bailis, R., Drigo, R., Ghilardi, A., and Masera, O. (2015). The carbon footprint of traditional woodfuels. Nat. Clim. Change 5, 266–272. doi: 10.1038/nclimate2491
Bailis, R., Wang, Y., Drigo, R., Ghilardi, A., and Masera, O. (2017). Getting the numbers right: revisiting woodfuel sustainability in the developing world. Environ. Res. Lett. 12:115002. doi: 10.1088/1748-9326/aa83ed
Bazilian, M., Rogner, H., Howells, M., Hermann, S., Arent, D., Gielen, D., et al. (2011). Considering the energy, water and food nexus: towards an integrated modelling approach. Energy Policy 39, 7896–7906. doi: 10.1016/j.enpol.2011.09.039
Beukering, P. J. H., Kahyararab, G., Masseya, E., di Primaa, S., Hessa, S., Makundi, V., et al. (2007). Optimization of the Charcoal Chain in Tanzania. PREM [Poverty Reduction and Environmental Management Working Paper] 07/03. Amsterdam: Institute for Environmental Studies.
Bialkowski, R., and Buttle, J. M. (2015). Stemflow and throughfall contributions to soil water recharge under trees with differing branch architectures. Hydrol. Process. 29, 4068–4082. doi: 10.1002/hyp.10463
Blanco-Sepúlveda, R., and Nieuwenhuyse, A. (2011). Influence of topographic and edaphic factors on vulnerability to soil degradation due to cattle grazing in humid tropical mountains in northern Honduras. Catena 86, 130–137. doi: 10.1016/j.catena.2011.03.007
Bonan, G. B. (2008). Forests and climate change: forcings, feedbacks, and the climate benefits of forests. Science 320, 1444–1449. doi: 10.1126/science.1155121
Bonell, M., and Bruijnzeel, L. A. (2005). Forests, Water and People in the Humid Tropics: Past, Present and Future Hydrological Research for Integrated Land and Water Management. International Hydrology Series. Cambridge: Cambridge University Press.
Booth, M. S., Caldwell, M. M., and Stark, J. M. (2003). Overlapping resource use in three Great Basin species: implications for community invasibility and vegetation dynamics. J. Ecol. 91, 36–48. doi: 10.1046/j.1365-2745.2003.00739.x
Bosch, J. M., and Hewlett, J. D. (1982). A review of catchment experiments to determine the effect of vegetation changes on water yield and evapotranspiration. J. Hydrol. 55, 3–23. doi: 10.1016/0022-1694(82)90117-2
Boutette, F. I., and Karch, E. G. (1984). Charcoal: Small Scale Production and Use. Eschborn: Deutsche Gesellschatf fü Technische Zusammenarbeit (GTZ)
Bradshaw, C., Sodhi, N., Peh, K., and Brook, B. (2007). Global evidence that deforestation amplifies flood risk and severity in the developing world. Glob. Change Biol. 13, 2379–2395. doi: 10.1111/j.1365-2486.2007.01446.x
Breshears, D. D., and Barnes, F. J. (1999). Interrelationships between plant functional types and soil moisture heterogeneity for semiarid landscapes within the grassland/forest continuum: A unified conceptual model. Landsc. Ecol. 14:465. doi: 10.1023/A:1008040327508
Bruijnzeel, L. A. (2004). Hydrological functions of tropical forests: not seeing the soil for the trees? Agric. Ecosyst. Environ. 104, 185–228. doi: 10.1016/j.agee.2004.01.015
Bruijnzeel, L. A. (2006). Hydrological Impacts of Converting Tropical Montane Cloud Forest to Pasture with Initial Reference to Northern Costa Rica. Final Technical Report DFID-FRP Project No. R7991. Amsterdam; Aylesford: VU University Amsterdam; Forestry Research Programme of the UK Department for International Development.
Carlyle-Moses, D. E., and Gash, J. H. C. (2011). “Rainfall interception loss by forest canopies,” in Forest Hydrology and Biogeochemistry: Synthesis of Past Research and Future Directions. eds D. F. Levia, D. Carlyle-Moses, and T. Tanaka (Dordrecht: Springer Netherlands), 407–423. doi: 10.1007/978-94-007-1363-5_20
Carrari, E., Ampoorter, E., Verheyen, K., Coppi, A., and Selvi, F. (2016). Former charcoal kiln platforms as microhabitats affecting understorey vegetation in Mediterranean forests. Appl. Veg. Sci. 19, 486–497. doi: 10.1111/avsc.12238
Castillo-Hernández, L. A. (2017). Sinergias y Compensaciones Entre Servicios Ecosistémicos Múltiples: el Caso de la Ganadería y las Plantas útiles en Bosques de Encino Manejados Para Producir Carbón Vegetal. Master's thesis, Universidad Nacional Autónoma de México, Morelia, MX.
Cerdá, A., and Doerr, S. H. (2005). Influence of vegetation recovery on soil hydrology and erodibility following fire: an 11-year investigation. Int. J. Wildland Fire. 14, 423–437. doi: 10.1071/WF05044
Certini, G. (2005). Effects of fire on properties of forest soils: A review. Oecologia 143, 1–10. doi: 10.1007/s00442-004-1788-8
Chambers, J. Q., and Artaxo, P. (2017). Deforestation size influences rainfall. Nat. Clim. Chang. 7:175. doi: 10.1038/nclimate3238
Chazdon, R. L. (2003). Tropical forest recovery: legacies of human impact and natural disturbances. Perspect. Plant Ecol. Evol. Syst. 6, 51–71. doi: 10.1078/1433-8319-00042
Chidumayo, E. N. (1991). Woody biomass structure and utilisation for charcoal production in a Zambian miombo woodland. Bioresour. Technol. 37, 43–52. doi: 10.1016/0960-8524(91)90110-6
Chidumayo, E. N., and Gumbo, D. J. (2013). The environmental impacts of charcoal production in tropical ecosystems of the world: A synthesis. Energy Sustain. Dev. 17, 86–94. doi: 10.1016/j.esd.2012.07.004
Clark, M. L., Aide, T. M., Grau, H. R., and Riner, G. (2010). A scalable approach to mapping annual land cover at 250 m using MODIS time series data: A case study in the Dry Chaco ecoregion of South America. Remote Sens. Environ. 114, 2816–2832. doi: 10.1016/j.rse.2010.07.001
Claus, A., and George, E. (2005). Effect of stand age on fine-root biomass and biomass distribution in three European forest chronosequences. Can. J. For. Res. 35, 1617–1625. doi: 10.1139/x05-079
Colón, S. M., and Lugo, A. E. (2006). Recovery of a subtropical dry forest after abandonment of different land uses. Biotropica 38, 354–364. doi: 10.1111/j.1744-7429.2006.00159.x
D'Almeida, C., Vörösmarty, C. J., Hurtt, G. C., Marengo, J. A., Dingman, S. L., and Keim, B. D. (2007). The effects of deforestation on the hydrological cycle in Amazonia: a review on scale and resolution. Int. J. Climatol. 27, 633–647. doi: 10.1002/joc.1475
DeFries, R., and Rosenzweig, C. (2010). Toward a whole-landscape approach for sustainable land use in the tropics. Proc. Natl. Acad. Sci. U.S.A. 107, 19627–19632. doi: 10.1073/pnas.1011163107.
Dekker, S. C., Rietkerk, M., and Bierkens, M. F. P. (2007). Coupling microscale vegetation-soil water and macroscale vegetation-precipitation feedbacks in semiarid ecosystems. Glob. Chang. Biol. 13, 671–678. doi: 10.1111/j.1365-2486.2007.01327.x
Dias, L. C. P., Macedo, M. N., Costa, M. H., Coe, M. T., and Neill, C. (2015). Effects of land cover change on evapotranspiration and streamflow of small catchments in the Upper Xingu River Basin, Central Brazil. J. Hydrol. Reg. Stud. 4, 108–122. doi: 10.1016/j.ejrh.2015.05.010
Doerr, S. H., Shakesby, R. A., and Walsh, R. P. D. (2000). Soil water repellency: its causes, characteristics and hydro-geomorphological significance. Earth Sci. Rev. 51, 33–65. doi: 10.1016/S0012-8252(00)00011-8
Doggart, N., and Meshack, C. (2017). The marginalization and sustainable charcoal production in the policies of the modernizing African Nation. Front. Environ. Sci. 5:27. doi: 10.3389/fenvs.2017.00027
Eckholm, E., Foley, G., Barnard, G., and Timberlake, L. (1984). Fuel Fuelwood: The Energy Crisis That Won't Go Away. London: Earthscan.
Eliason, S. A., and Allen, E. B. (1997). Exotic grass competition in suppressing native shrubland re-establishment. Restorat. Ecol. 5, 245–255. doi: 10.1046/j.1526-100X.1997.09729.x
Endo, A., Tsurita, I., Burnett, K., and Orencio, P. M. (2017). A review of the current state of research on the water, energy, and food nexus. J. Hydrol. 11, 20–30. doi: 10.1016/j.ejrh.2015.11.010
FAO (2010). Global Forest Resources Assessment 2010, Terms and Definitions. Forestry Department, Food and Agriculture Organization of the United Nations, Rome.
FAO (2017). The Charcoal Transition: Greening The Charcoal Value Chain To Mitigate Climate Change And Improve Local Livelihoods. Food and Agriculture Organization of the United Nations, Rome.
Farrick, K., and Branfireun, B. (2014). Infiltration and soil water dynamics in a tropical dry forest: it may be dry but definitely not arid. Hydrol. Process. 28, 4377–4387. doi: 10.1002/hyp.10177
Ferraro, P. J., Hanauer, M. M., and Sims, K. R. (2011). Conditions associated with protected area success in conservation and poverty reduction. Proc. Natl. Acad. Sci. U.S.A. 108, 13913–13918. doi: 10.1073/pnas.1011529108
Fontodji, J., Mawussi, G., Nuto, Y., and Kokou, K. (2009). Effects of charcoal production on soil biodiversity and soil physical and chemical properties in Togo, West Africa. Int. J. Biol. Chem. Sci. 3:1051. doi: 10.4314/ijbcs.v3i5.51051
Fontodji, J. K., Atsri, H., Adjonou, K., Radji, A. R., Kokutse, A. D., Nuto, Y., et al. (2011). “Impact of charcoal production on biodiversity in Togo (West Africa),” in The Importance of Biological Interactions in the Study of Biodiversity, ed J. L. Pujol (London: InTech). doi: 10.5772/22969
Geist, H. J., and Lambin, E. F. (2004). Dynamic causal patterns of desertification. AIBS Bull. 54, 817–829. doi: 10.1641/0006-3568(2004)0540817:DCPOD2.0.CO;2
Gezahegn, A. K. (2018). Characterization of Rangeland Resources and Dynamics of the Pastoral Production Systems in the Somali Region of Eastern Ethiopia. Doctoral dissertation, University of the Free State.
Ghilardi, A., Bailis, R., Mas, J. F., Skutsch, M., Elvir, J. A., Quevedo, A., et al. (2016). Spatiotemporal modeling of fuelwood environmental impacts: towards improved accounting for non-renewable biomass. Environ. Model. Softw. 82, 241–254. doi: 10.1016/j.envsoft.2016.04.023
Gifford, G. F., and Hawkins, R. H. (1978). Hydrologic impact of grazing on infriltration: a critical review. Water Resour. Res. 14, 305–312. doi: 10.1029/WR014i002p00305
Glaser, B., Lehmann, J., and Zech, W. (2002). Ameliorating physical and chemical properties of highly weathered soils in the tropics with charcoal–a review. Biol. Fertil. Soils 35, 219–230. doi: 10.1007/s00374-002-0466-4
Godsey, S., and Elsenbeer, H. (2002). The soil hydrologic response to forest regrowth: a case study from southwestern Amazonia. Hydrol. Process. 16, 1519–1522. doi: 10.1002/hyp.605
Guzha, A. C., Rufino, M. C., Okoth, S., Jacobs, S., and Nóbrega, R. L. B. (2018). Impacts of land use and land cover change on surface runoff, discharge and low flows: evidence from East Africa. J. Hydrol. Reg. Stud. 15, 49–67. doi: 10.1016/j.ejrh.2017.11.005
Hamilton, L. S., and King, P. N. (1983). Tropical Forested Watersheds: Hydrologic and Soils Response to Major Uses or Conversions. Boulder, CO: Westview Press.
Hanson, C. L., Kuhlman, A. R., Erickson, C. J., and Lewis, J. K. (1970). Grazing effects on runoff and vegetation on western south dakota rangeland. J. Range Manage. 23, 418–420. doi: 10.2307/3896312
Hassler, S. K., Zimmermann, B., Breugel, M., Hall, J. S., and Elsenbeer, H. (2011). Recovery of saturated hydraulic conductivity under secondary succession on former pasture in the humid tropics. For. Ecol. Manage. 261, 1634–1642. doi: 10.1016/j.foreco.2010.06.031
Henchion, M., McCarthy, M., Resconi, V. C., and Troy, D. (2014). Meat consumption: trends and quality matters. Meat Sci. 98, 561–568. doi: 10.1016/j.meatsci.2014.06.007
Herrero, M., Thornton, P. K., Gerber, P., and Reid, R. S. (2009). Livestock, livelihoods and the environment: understanding the trade-offs. Curr. Opin. Environ. Sustain. 1, 111–120. doi: 10.1016/j.cosust.2009.10.003
Hoff, H. (2011). Understanding the Nexus. Background Paper for the Bonn 2011 Conference: The Water, Energy and Food Security Nexus. Stockholm: Stockholm Environment Institute.
Johnson, M. S., and Lehmann, J. (2006). Double-funneling of trees: stemflow and root-induced preferential flow. Ecoscience 13, 324–333. doi: 10.2980/i1195-6860-13-3-324.1
Lach, D., Rayner, S., and Ingram, H. (2005). Taming the Waters: strategies to domesticate the wicked problems of water resource management. Int. J. Water 3, 1–17. doi: 10.1504/IJW.2005.007156
Levia, D. F., Carlyle-Moses, D., and Tanaka, T. (2011). Forest Hydrology and Biogeochemistry: Synthesis of Past Research and Future Directions. New York, NY: Ecological Studies; Springer. doi: 10.1007/978-94-007-1363-5
Levia, D. F., and Frost, E. E. (2003). A review and evaluation of stemflow literature in the hydrologic and biogeochemical cycles of forested and agricultural ecosystems. J. Hydrol. 274, 1–29. doi: 10.1016/S0022-1694(02)00399-2
Levia, D. F., Van Stan, J. T., Mage, S. M., and Kelley-Hauske, P. W. (2010). Temporal variability of stemflow volume in a beech-yellow poplar forest in relation to tree species and size. J. Hydrol. 380, 112–120. doi: 10.1016/j.jhydrol.2009.10.028
Llorens, P., and Domingo, F. (2007). Rainfall partitioning by vegetation under Mediterranean conditions. A review of studies in Europe. J. Hydrol. 335, 37–54. doi: 10.1016/j.jhydrol.2006.10.032
Lobato, J. F., Freitas, A. K., Devincenzi, T., Cardoso, L. L., Tarouco, J. U., Vieira, R. M., et al. (2014). Brazilian beef produced on pastures: sustainable and healthy. Meat Sci. 98. 336–345. doi: 10.1016/j.meatsci.2014.06.022
Lozano-Parra, J., van Schaik, N. L. M. B., Schnabel, S., and Gómez-Gutiérrez, Á. (2016). Soil moisture dynamics at high temporal resolution in a semiarid Mediterranean watershed with scattered tree cover. Hydrol. Process. 30, 1155–1170. doi: 10.1002/hyp.10694
Ludwig, J. A., Wilcox, B. P., Breshears, D. D., Tongway, D. J., and Imeson, A. C. (2005). Vegetation patches and runoff-erosion as interacting ecohydrological processes in semiarid landscapes. Ecology 86, 288–297. doi: 10.1890/03-0569
Maass, J. M., Balvanera, P., Castillo, A., Daily, G. C., Mooney, H. A., Ehrlich, P., et al. (2005). Ecosystem services of tropical dry forests: insights from longterm ecological and social research on the Pacific Coast of Mexico. Ecol. Soc. 10, 1–23. doi: 10.5751/ES-01219-100117
Maass, M. (2017). Integrating food-water-energy research through a socio-ecosystem approach. Front. Environ. Sci. 5:48. doi: 10.3389/fenvs.2017.00048
McAlpine, C. A., Etter, A., Fearnside, P. M., Seabrook, L., and Laurance, W. F. (2009). Increasing world consumption of beef as a driver of regional and global change: a call for policy action based on evidence from Queensland (Australia), Colombia and Brazil. Global Environ. Change 19, 21–33. doi: 10.1016/j.gloenvcha.2008.10.008
McLaren, K. P., and McDonald, M. A. (2003). Coppice regrowth in a disturbed tropical dry limestone forest in Jamaica. For. Ecol. Manage. 180, 99–111. doi: 10.1016/S0378-1127(02)00606-0
Murphy, P. G., and Lugo, A. E. (1986). Ecology of tropical dry forest. Annu. Rev. Ecol. Syst. 17, 67–88. doi: 10.1146/annurev.es.17.110186.000435
Mwampamba, T. (2007). Has the woodfuel crisis returned? Urban charcoal consumption in Tanzania and its implications to present and future forest availability. Energy Policy 35, 4221–4234. doi: 10.1016/j.enpol.2007.02.010
Mwampamba, T. (2009). Forest Recovery and Carbon Sequestration Under Shifting Cultivation in the Eastern Arc Mountains, Tanzania: Landscape and Landuse Effects. Dissertation, University of California, Davis, CA.
Mwampamba, T. H., Ghilardi, A., Sander, K., and Chaix, K. J. (2013). Dispelling common misconceptions to improve attitudes and policy outlook in developing countries. Energy Sustain. Dev. 17, 75–85. doi: 10.1016/j.esd.2013.01.001
Mwendera, E. J., and Mohamed Saleem, M. A. (1997). Hydrologic response to cattle in the Ethiopian highlands. Agric. Ecosyst. Environ. 64, 33–41. doi: 10.1016/S0167-8809(96)01127-9
Návar, J. (2011). Stemflow variation in Mexico's northeastern forest communities: its contribution to soil moisture content and aquifer recharge. J. Hydrol. 408, 35–42. doi: 10.1016/j.jhydrol.2011.07.006
Negrete-Yankelevich, S., Fragoso, C., Newton, A. C., and Heal, O. W. (2007). Successional changes in soil, litter and macroinvertebrate parameters following selective logging in a Mexican Cloud Forest. Appl. Soil Ecol. 35, 340–355. doi: 10.1016/j.apsoil.2006.07.006
Nelle, O. (2003). Woodland history of the last 500 years revealed by anthracological studies of charcoal kiln sites in the Bavarian Forest, Germany. Phytocoenologia 33, 667–682. doi: 10.1127/0340-269X/2003/0033-0667
Nigussie, A., and Kissi, E. (2011). Effect of charcoal production on soil properties in Southwestern Ethiopia. Middle East J. Sci. Res. 9, 807–813.
Oguntunde, P. G., Abiodun, B. J., Ajayi, A. E., and Giesen, N. (2008). Effects of charcoal production on soil physical properties in Ghana. J. Plant Nutr. Soil Sci. 171, 591–596. doi: 10.1002/jpln.200625185
Oguntunde, P. G., Fosu, M., Ajayi, A. E., and Van De Giesen, N. (2004). Effects of charcoal production on maize yield, chemical properties and texture of soil. Biol. Fertil. Soils 39, 295–299. doi: 10.1007/s00374-003-0707-1
Owen, M. (2013). The Legally Compliant Charcoal Industry and its Barriers to Growth in Kenya. Final Report in Support to Forest Sector Reform in Kenya. NIRAS Finland, 42.
Pedraza, R., and Williams-Linera, G. (2003). Evaluation of native tree species for the rehabilitation of deforested areas in a Mexican cloud forest. N. Forests 26:83. doi: 10.1023/A:1024423511760
Pennise, D. M., Smith, K. R., Kithinji, J. P., Rezende, M. E., Raad, T. J., Zhang, J., et al. (2001). Emissions of greenhouse gases and other airborne pollutants from charcoal making in Kenya and Brazil. J. Geophys. Res. Atmos. 106, 24143–24155. doi: 10.1029/2000JD000041
Perevochtchikova, M., Tamayo, O., and Milena, A. (2012). Avances y limitantes del programa de pago de servicios ambientales hidrológicos en México, 2003-2009. Revista Mexicana de Ciencias Forestales 3, 89–112. Available online at: http://www.scielo.org.mx/scielo.php?pid=S2007-11322012000200008&script=sci_arttext
Picos, J., and Valero, E. (2009). “Forest land resources,” in Forests and Forest Plants-Volume I, eds Owens and Lund (Paris: UNESCO-EOLSS), 66.
Plieninger, T., Schaich, H., and Kizos, T. (2011). Land-use legacies in the forest structure of silvopastoral oak woodlands in the Eastern Mediterranean. Reg. Environ. Change. 11:603. doi: 10.1007/s10113-010-0192-7
Pressland, A. J. (1976). Possible effects of removal of mulga on rangeland stability in south western Queensland. Aust. Rangeland J. 1, 24–30. doi: 10.1071/RJ9760024
Randriamalala, J. R., Radosy, H. O., Razanaka, S., Randriambanona, H., and Hervé, D. (2016). Effects of goat grazing and woody charcoal production on xerophytic thickets of southwestern Madagascar. J. Arid Environ. 128, 65–72. doi: 10.1016/j.jaridenv.2016.01.002
Rauzi, F., and Hanson, D. L. (1966). Water intake and runoff as affected by intensity of grazing. J. Range Manage. 19, 351–356. doi: 10.2307/3895570.
Rittel, H. W. J., and Webber, M. M. (1973). Dilemmas in a general theory of planning. Policy Sci. 4:155. doi: 10.1007/BF01405730
SAGARPA (2009). Tinas Ciegas. Especialidad de Hidrociencias del Colegio de Postgraduados, Montecillos, Estado de México.
Santos, M. J., Dekker, S. C., Daioglou, V., Braakhekke, M. C., and van Vuuren, D. P. (2017). Modeling the effects of future growing demand for charcoal in the tropics. Front. Environ. Sci. 5:28. doi: 10.3389/fenvs.2017.00028
Schnabel, S., Gutiérrez, A., and Contador, J. F. (2009). “Grazing and soil erosion in dehesas of SW Spain,” in Avances en Estudios Sobre Desertificación. Contributions to the International Conference in memory of Prof. Johm B. Thornes, eds F. Romero-Díaz, F. Belmonte Serrato, S. Alonso, and F. López Bermúdez (Murcia: Editum Universidad de Murcia), 725–728.
Sedano, F., Silva, J. A., Machoco, R., Meque, C. H., Sitoe, A., Ribeiro, N., et al. (2016). The impact of charcoal production on forest degradation: a case study in Tete, Mozambique. Environ. Res. Lett. 11:094020. doi: 10.1088/1748-9326/11/9/094020
Seneviratne, S., and Stöckli, R. (2008). “The Role of Land-Atmosphere Interactions for Climate Variability in Europe,” in Climate Variability and Extremes during the Past 100 Years. Advances in Global Change Research, eds S. Brönnimann, J. Luterbacher, T. Ewen, H. Diaz, R. Stolarski, and U. Neu (Dordrecht: Springer), 179–193.
Sodhi, N. S., Butler, R., Laurance, W. F., and Gibson, L. (2011). Conservation successes at micro-, meso-and macroscales. Trends Ecol. Evol. 26, 585–594. doi: 10.1016/j.tree.2011.07.002
Specht, M. J., Pinto, S. R. R., Albuquerque, U. P., Tabarelli, M., and Melo, F. P. (2015). Burning biodiversity: fuelwood harvesting causes forest degradation in human-dominated tropical landscapes. Global Ecol. Conservat. 3, 200–209. doi: 10.1016/j.gecco.2014.12.002
Sun, G., Domec, J. C., and Amatya, D. M. (2016). “Forest evapotranspiration: measurements and modeling at multiple scales,” in Forest Hydrology: Processes, Management and Assessment, eds D. Amatya, T. Williams, L. Bren, and C. de Jong (Boston, MA: CABI Publishers), 19, 32–50.
Swami, S. N., Steiner, C., Teixeira, W. G., and Lehmann, J. (2009). “Charcoal making in the Brazilian Amazon: economic aspects of production and carbon conversion efficiencies of kilns,” in Amazonian Dark Earths: Wim Sombroek's Vision, eds W. I. Woods, J. Lehmann, L. Rebellato, C. Steiner, W. G. Teixeira, and A. WinklerPrins (Berlin: Springer), 411–422.
Trejo, I., and Dirzo, R. (2000). Deforestation of seasonally dry tropical forest: a national and local analysis in Mexico. Biol. Conserv. 94, 133–142. doi: 10.1016/S0006-3207(99)00188-3
Trimble, S. W., and Mendel, A. C. (1995). The cow as a geomorphic agent—a critical review. Geomorphology 13, 233–253. doi: 10.1016/0169-555X(95)00028-4
Uriarte, M., Yackulic, C. B., Lim, Y., and Arce-Nazario, J. A. (2011). Influence of land use on water quality in a tropical landscape: a multi-scale analysis. Landscape Ecol. 26:1151. doi: 10.1007/s10980-011-9642-y
Vertessy, R. A., Watson, F. G. R., and O'Sullivan, S. K. (2001). Factors determining relations between stand age and catchment water balance in mountain ash forests. For. Ecol. Manage. 143, 13–26. doi: 10.1016/S0378-1127(00)00501-6
Voorhoeve, J. (2017). Modelling effects of charcoal production on the carbon Budget in managed oak forest of Central Mexico. Master's thesis. Utrecht University, Utrecht.
Wahabu, S., Fosu-Mensah, B. Y., and Nyame, F. K. (2015). Impact of charcoal production on physical and chemical properties of soil in the central gonja district of the Northern region, Ghana. Environ. Nat. Resour. Res. 5, 11–18. doi: 10.5539/enrr.v5n3p11
Ward, S. E., Bardgett, R. D., McNamara, N. P., Adamson, J. K., and Ostle, N. J. (2007). Long-term consequences of grazing and burning on northern peatland carbon dynamics. Ecosystems 10, 1069–1083. doi: 10.1007/s10021-007-9080-5
Woollen, E., Ryan, C. M., Baumert, S., Vollmer, F., Grundy, I., Fisher, J., et al. (2016). Charcoal production in the mopane woodlands of mozambique: what are the trade-offs with other ecosystem services? Philos. Trans. R. Soc. Lond. B Biol. Sci. 371, 653–660. doi: 10.1098/rstb.2015.0315
Zhang, L., Dawes, W., and Walker, G. (2001). Response of mean annual evapotranspiration to vegetation changes at catchment scale. Water Resour. Res. 37, 701–708. doi: 10.1029/2000WR900325
Zhang, M., Liu, N., Harperc, R., Li, Q., Liu, K., Wei, X., et al. (2017). A global review on hydrological responses to forest change across multiple spatial scales: importance of scale, climate, forest type and hydrological regime. J. Hydrol. 546, 44–59. doi: 10.1016/j.jhydrol.2016.12.040
Zou, C. B., Caterina, G. L., Will, R. E., Stebler, E., and Turton, D. (2015). Canopy interception for a tallgrass prairie under juniper encroachment. PLoS ONE 10:e0141422. doi: 10.1371/journal.pone.0141422
Keywords: biomass energy, charcoal, ecohydrology, grazing, nexus
Citation: Mwampamba TH, van Schaik NLMB and Castillo Hernandez LA (2018) Incorporating Ecohydrological Processes Into an Analysis of Charcoal-Livestock Production Systems in the Tropics: An Alternative Interpretation of the Water-Energy-Food Nexus. Front. Environ. Sci. 6:99. doi: 10.3389/fenvs.2018.00099
Received: 26 February 2018; Accepted: 20 August 2018;
Published: 11 September 2018.
Edited by:
Luuk Fleskens, Wageningen University & Research, NetherlandsReviewed by:
Subhrajit Saha, Georgia Southern University, United StatesKegan Farrick, University of the West Indies, Monaco
Copyright © 2018 Mwampamba, van Schaik and Castillo Hernandez. This is an open-access article distributed under the terms of the Creative Commons Attribution License (CC BY). The use, distribution or reproduction in other forums is permitted, provided the original author(s) and the copyright owner(s) are credited and that the original publication in this journal is cited, in accordance with accepted academic practice. No use, distribution or reproduction is permitted which does not comply with these terms.
*Correspondence: Tuyeni H. Mwampamba, dHV5ZW5pQGlpZXMudW5hbS5teA==