- 1Department of Microbiology, University of Uyo, Uyo, Nigeria
- 2Department of Chemistry, University of Uyo, Uyo, Nigeria
- 3School of Water, Energy and Environment, Cranfield University, Cranfield, United Kingdom
Effect of source separated human urine as buffering agent compared to sodium bicarbonate and water in anaerobic co-digestion of lignocellulosic biomass and poultry feces was evaluated in laboratory scale reactor for 180 days at 37 ± 2°C. Mean biogas volume ranged from 37 ± 8 to 101 ± 18 mL gVS−1 in the urine buffered reactors which was 1–5 times higher than the bicarbonate and water buffered reactors and the difference was significant at p = 0. 05. Total volatile fatty acids (VFA) concentration ranged between 396 and 1,400 mg L−1 with a pH of 6.9 ± 0.3 and 7.8 ± 0.1, respectively. In contrast, VFA concentration ranged between 386 and 3,109 mg L−1 (pH 7.6 ± 0.2 and 4.8 ± 0.4) in sodium bicarbonate buffered digestate and control (water) respectively. The result indicates buffering capacity of urine on anaerobic co-digestion with positive effect on biogas production. The Archaeal isoprenoids included markers of aceticlastic and hydrogenotrophic methanogens with a relative abundance that ranged between 0.71–18, 3–55, and 2–59 μg g−1 dry matter in the water (control), bicarbonate and urine buffered digestate, respectively. The Archaeal abundance was 1.12 and 6 times higher in the combined female/male urine than the bicarbonate buffered digestate and the control, and the difference was significant at p = 0.05. Overall, this study demonstrates that human urine with no pharmaceutical loadings as a wetting and buffering agent is a promising option for anaerobic co-digestion with competitive edge over sodium bicarbonate on lignocellulosic biomass saccharification for enhanced biogas production.
HIGHLIGHTS
- Source separated human urine served as buffer in anaerobic co-digestion process.
- Combined female/male urine exerted an additive effect on biogas production.
- Competitive edge offered by the combined female/male urine over sodiumbicarbonate buffer in relation to biogas produced.
- Evidence of synergy for enhanced biogas production fromhigh C/N ratio lignocellulosic biomass combined with low C/N ratio poultry feces.
Introduction
Increased human population is associated with high energy demand from the burning of fossil fuels have been implicated in environmental pollution with global warming impacts. These have heightened the search for alternative energy sources to minimize further damage to the Earth's ecosystem (Karagöz et al., 2012). It is common knowledge that renewable energy production of biofuels such as methane (60–70%) and stabilized digestate by anaerobic digestion (AD) creates economic opportunities and can minimize environmental pollution when the reactor is operated at optimal conditions. The production harnesses the degrading potential of microorganisms in a process that is friendly to the environment, reduce odor and kill pathogenic organisms especially in reactors operated at thermophilic conditions (Appels et al., 2008).
However, anaerobic digestion is a complex synergistic biochemical process that involves sequential hydrolysis as a rate-limiting step, acidogenesis, acetogenesis, and methanogenesis. The process is mediated by a diverse and complex microbial community that requires different optimum conditions to grow, metabolize, and are influenced by factors such as pH, concentration of free ammonia, alkalinity, volatile fatty acid accumulation, heavy metals, sodium, hydrogen, and potassium. Although the AD process has been shown as an efficient technology for biofuel production, the use of different organic feedstock material can influence the process stability (Appels et al., 2008). This is because the organic substrate vary in their physicochemical characteristics especially the carbon-nitrogen ratio. In addition, the process is sensitive to changes in pH, temperature, microbial community composition, presence of inhibitory substances, and micro-pollutants. Thus, an efficient process requires a delicate balance of microbial groups, substrate composition and optimum operating conditions (Kayhanian, 1994; Appels et al., 2008; Wagner et al., 2010).
The use of lignocellulosic biomass in AD provides fermentable sugars from cheap and readily available substrate (Balat, 2011; Paul and Dutta, 2018). For instance, cellulose is the most dominant polysaccharide component in lignocellulosic biomass made up of linear glucose units linked by β-1, 4 glucosidic bonds. However, the complex structural composition and crystalline nature of the biomass requires pre-treatment to increase permeability, cleave the cellulose-hemicellulose-lignin bonds, and release the fermentable cellulose for microbial conversion (Barton, 1988; Jacobsen and Wyman, 2000; Badshah et al., 2012; Gaur et al., 2017). Pre-treatment of lignocellulosic biomass involve the use of methods and substances such as acids, alkali, steam explosion, organic solvent, alkaline-hydrogen peroxide, ammonia, and hot water in addition to enzyme hydrolysis (Sreenath et al., 1999; Torget et al., 2000; Foyle et al., 2007). Most of the method and substances, for example acid, alkaline, and alkaline-peroxide pre-treatments enhanced the release of soluble sugars from lignocellulosic biomass compared to thermal pre-treatment with low sugars. However, these substances generated by-products such as furfurals that inhibit microbial growth and community activity in relation to methane production (Bolado-Rodriquez et al., 2016). Indeed, the high carbon:nitrogen (C/N) ratio of lignocellulosic biomass is a major limiting factor for higher biogas generation during anaerobic digestion. To circumvent this, a low C/N ratio biomass combined with high C/N ratio lignocellulosic biomass can provide the required complement for efficient anaerobic co-digestion and biogas production (Paul and Dutta, 2018).
In most cases, a stable AD process is maintained by the use of buffers such as sodium bicarbonate, potassium bicarbonate, potassium carbonate, sodium nitrate, and anhydrous ammonia (Lin et al., 2013) to keep the pH between 6.8 and 7.2. On the other hand, anaerobic co-digestion of organic waste has gained acceptance because the process can remain stable and efficient without the use of chemical buffers, and with a minimal or no post AD processing of digestate for disposal. In spite of this, establishing the right feedstock combination for anaerobic co-digestion is a challenge and can significantly influence biogas production (Gaur and Suthar, 2017). A different perspective on this will involve natural substances and/or waste material with relevant or similar composition as the chemical buffers to offer an alternative option, augment nutrient, and moisture for stable AD process. For example, the human urine is a body fluid and waste expected to be disposed of without any major application. In terms of composition in the domestic sewage, urine supplies approximately 70–80% of the nitrogen, 50–70% of the phosphorus, and 60–70% of the pharmaceutical loadings (Jimenez et al., 2015). In recent years, however, there is increased understanding that the composition of source separated urine suggests it can serve as nutrient and growth inducer for plant and microorganisms (Larsen et al., 2004; Akpan-Idiok et al., 2012; Andreev et al., 2017). Further to this, urine source separation commonly called NoMix-technology in urban wastewater management is accepted as innovative approach to increase water quality, reduce pharmaceutical loadings, and enhance nutrient recovery (Lienert and Larsen, 2010; Ekama et al., 2011; Jimenez et al., 2015; Landry and Boyer, 2016).
To assess the effect of urine on the anaerobic co-digestion of lignocellulosic biomass (sawdust) and poultry feces performance, the biomethane potential assay (BMP) was used. In addition, microorganisms in the inoculum (cow dung) were exposed to unspiked sawdust and poultry manure (control) for comparison. The effect was evaluated using microbial community abundance, biogas production and volatile fatty acids (VFA). The significance and implications of source separated human urine with no pharmaceutical loadings on the key steps of anaerobic digestion performance are also highlighted.
Materials and Methods
Substrate, Buffer and Wetting Agent
Lignocellulosic biomass (sawdust) and poultry feces were used in this study as substrate for the AD process. Water, sodium bicarbonate anhydrous (Na2CO3, 99.5% pure) and fresh human urine (male and female separately and in combination) were used as wetting and buffering agents in the reactors. The source separated urine samples were provided anonymously from the male and female toilets of the University of Uyo. To ensure that the research study conforms with appropriate ethical principles and standards, the research was submitted and approved by the Uyo University's Research Ethics Committee. Accordingly donors were informed of the purpose, method and intended use of the research and written informed consent was obtained from all participants. Early morning urine samples from the human subjects not on any drug therapy for at least 7 days prior to the sampling period were collected in clean sterile containers. We adopted this approach rather than random sample collection to minimize the influence of pharmaceutical loadings in the urine which inhibited the microorganisms and contributed to the low biogas produced in our previous experimental setup. In addition, the choice of the substrates was based on the carbon-nitrogen (C/N) ratios. For instance, the poultry feces is relatively a lower C/N value material (C/N ratio: 5–15) whereas the sawdust is a higher C/N value material (C/N ratio: 200–500) (Hagos et al., 2017). The sawdust from untreated wood/timber and poultry feces were collected from the Ultra-modern Timber Market, Uyo and the University of Uyo Farms respectively. The samples in polyethylene bags were transported to the Microbiological laboratory, University of Uyo for the biomethane potential assay.
Experimental Design
The biomethane potential (BMP) assay was used as an index of the anaerobic bioconversion to measure the volume of biogas produced per gram of volatile solid in the substrate. We measured the substrate digested and biogas produced by a modified approach as described by Owen et al. (1979) and Angelidaki et al., (2003, 2009) by subjecting the lignocellulosic biomass to solid state fermentation with a near absence of free moisture from water or urine. Briefly, 200 g each of sawdust and poultry feces as the co-substrate were separately moistened with either 10 mL of distilled water or source separated fresh urine samples, mixed in a wooden trough and allowed to stand for 14 days at room temperature (28 ± 2°C) as a form of pre-treatment. The biogas assay was set up in a batch mode and involved the use of 100 mL amber serum bottles (Gerresheimer 61020G, USA) and 20 mm aluminum cap with central molded septum combination seal (FB 67567, Fisher Scientific, UK) as reactor. 10 g subsample of the pre-treated substrate was inoculated with 5 g VS−1 aged-cow dung and fed into the 100 mL amber serum bottles. Cow dung was used as inoculum because of the associated methanogenic microorganisms with the potential for anaerobic co-digestion and biogas production (Gaur and Suthar, 2017). Prior to inoculation of the pre-treated substrate, the seed inoculum (cow dung) was incubated at 37.0 ± 0.2°C for 25 days until no biogas was detected from the reactor. The wetting/buffering agent used in the BMP assay was 45 mL of distilled water, male urine, female urine, and combined male/female urine respectively were added to separate pre-treated substrate and resulted in a total reaction volume of 60 mL. The combined female/male urine was 22.5 mL each. In another set up used as positive control, pH of the blended substrate was adjusted with sodium bicarbonate anhydrous (Na2CO3, 99.5% pure) to 7.2 ± 0.2 and were determined using standard methods (APHA, 2005). The content of the reactors were allowed to equilibrate for 1 h at 45°C and sealed using standard hand operated crimper, 20 mm cap size (JG Finneran 9300-20, USA). Triplicate reactors for each treatment were incubated in a thermostatically regulated water bath maintained at 37 ± 0.2°C with 15 min discontinuous manual agitation every 12 h for 180 days. At 3 days interval, gas volume was determined by volumetric method (Valcke and Verstraete, 1983) by connecting each reactor to a graduated reverse cylinder device containing water as a barrier solution and the liquid displacement measured and converted to biogas volume using the formula:
where r = internal radius of the column (cm); h = production of biogas as water level decreased in the column (cm); H = working length of gas collection column (cm); k = standard atmospheric pressure (1,033 cm water gauge).
Measurement of Volatile Fatty Acids (VFAs)
The VFA composition was measured using the approach described in Eduok et al. (2017) in which 10 mL duplicate digestate samples were centrifuged at 5,000 rpm for 20 min and filtered through 0.45 μm and then 0.2 μm syringe filter. 9 mL of filtered sample was inactivated and stabilized by adding 10 μL of sulphuric acid and frozen until needed for analysis. VFA concentrations were determined by high performance liquid chromatography (HPLC) on Kontron 535 detector (Bio-Tek, Vermont, USA) with a Bio-Rad (California, USA) HPLC column for fermentation monitoring. The column temperature was maintained at 60°C with an eluent of 1 mM H2SO4 with a flow rate of 0.8 mL min−1. Detection of VFA concentrations was done with ultraviolet light at 208 nm.
PLEL Markers
We measured the phospholipid etherlipid (PLEL) of the microbial biomass in the digestate by the approach as described by Gattinger et al. (2003). Briefly, Citrate buffer-chloroform-methanol of 0.8:1:2 (v/v/v) was used to extract 5 g of lyophilized digestate, subjected to solid-phase fractionation followed by transesterification through mild alkaline methanolysis. Aliquots of the phospholipid fraction equivalent to ~12.5 g dry matter were subjected to PLEL analysis (Gattinger et al., 2003). Acid hydrolysis and methylated cleavage of the polar head group was performed using 2 mL of methanol: chloroform: 37% hydrochloric acid (10:1:1, v/v/v). The dried ether-linked isoprenoids were then reconstituted in 0.2 mL of hexane and analyzed by gas chromatography coupled to mass spectrometry (GC-MS Agilent Technologies 6890N) according to the operating conditions (Gattinger et al., 2003). 200 μL nonadecanoic acid methyl ester (Sigma, UK) was added as an internal standard to each sample after solid phase extraction. In accordance with other studies, Archaeal biomass was estimated using a conversion factor of 5.9 × 1012 cells per 2.5 μmol PLEL (Bai et al., 2000).
Statistical Analysis
Kruskal-Wallis test was performed using Statistica software® version 11 (Statsoft, Tulsa, OK, USA) and values are presented as mean ± standard deviation with levels of significance maintained at 95% for each test. PLEL profiles were log-transformed to minimize skewed distribution and subjected to group-averaged hierarchical cluster analysis based on Bray-Curtis similarity measure using PRIMER version 6 (Clarke and Warwick, 2001).
Results and Discussion
Effect of Urine Buffer on Biogas Production and pH
The BMP assay at 180 days recorded a mean biogas volume ranging between 19 ± 5 and 102 ± 17 mL gVS d−1 in the control (water) and female/male urine buffered digester, respectively (Figure 1). The daily biogas production showed rapid biogas production for the first 40 days, followed by a decrease in biogas production between days 40 and 180 (Figure 2). The high initial biogas production was due to the preferential digestion of readily biodegradable organic materials. The dissipation of the readily degradable materials may have then caused biogas production decrease between days 40 and 180 (Figure 1). The sodium bicarbonate buffered reactor produced 1.1 times higher cumulative biogas than the female/male urine buffered reactor in the first 45 days but the trend was reversed after 55 days up to 180 days (Figure 2). The result indicates a lag period for a stable performance in relation to biogas produced from the female/male urine buffered reactor compared with sodium bicarbonate buffered reactor (Figure 2). In addition, the urine exerted a positive effect on the different groups of microorganisms associated with the saccharification of the lignocellulosic biomass and methanation than water. Further to this, the breakdown of lignocellulosic biomass into fermentable sugars by urine hydrolysis was more pronounced in the source separated urine buffered digestate. For instance, biogas volume from the male, female and male/female urine buffered digestate was 2, 3, and 5 times higher than in the control (water), respectively. However, in the combined female/male urine treatments, biogas production was 1.04, and 1.5 and 3 times higher than in the bicarbonate, female and male urine buffered digestate, respectively. Whereas, the biogas produced in the female urine buffered digestate was 2 times higher than in the male urine treatment and suggests a competitive advantage over the male urine as a buffer. Overall, the response of the methanogens from the female/male buffered digester in relation to biogas volume produced ranged from 1.04 to 5 times higher than all other treatments and the difference was significant at p = 0.05. The result has evidenced the synergy for enhanced biogas production from high C/N ratio lignocellulosic biomass combined with low C/N ratio poultry feces.
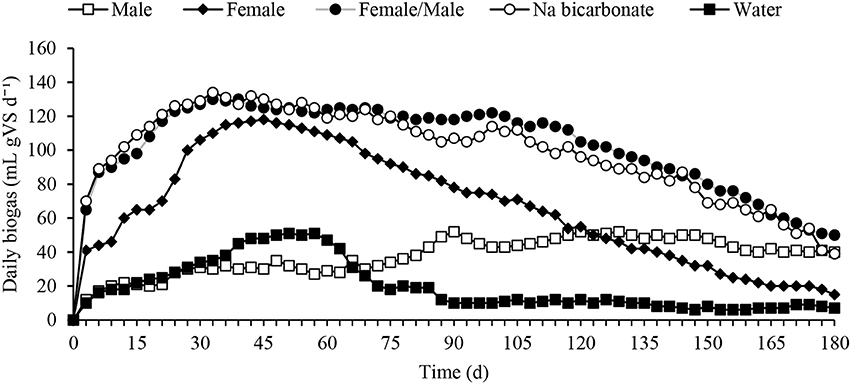
Figure 1. Daily biogas volume produced from anaerobic co-digestion of lignocellulosic biomass pre-treated with human urine, water and sodium bicarbonate. The combined female/male urine buffer in relation to other treatments enhanced biogas production. The biogas volume in a set of triplicate reactors were not significantly different (p = 0.05).
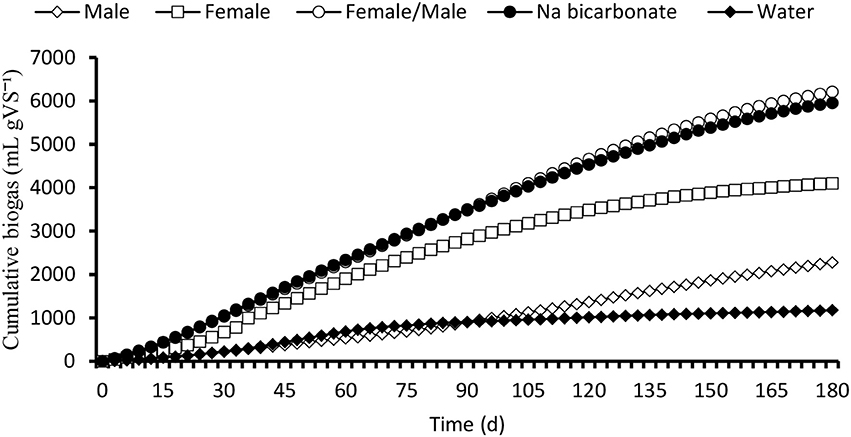
Figure 2. Cumulative biogas volume produced from the anaerobic co-digestion of the lignocellulosic biomass. Female/male urine had a competitive edge over the other treatments.
Similar to the commonly known ammonia fiber explosion (AFEX) approach used in the hydrolysis of lignocellulosic biomass (Bals et al., 2010), the results suggest that the fresh human urine and urea from the poultry feces with an initial pH that ranged between 5.3 ± 0.3 and 6.2 ± 0.2 in the water and female/male urine mixture, respectively served as a mild acid and contributed to the cellulose-hemicellulose-lignin bond cleavage which subsequently contributed to the release of the sugars for microbial metabolism. However, as the sugars were metabolized, the pH was expected to decrease due to an accumulation of VFA into the system. Notwithstanding this, the pH in the female/male urine buffered digestate was stable at 7.8 ± 0.1 (Figure 3) which indicated there was no imbalance as a result of accumulated VFAs. As a result of this, no significant negative effect was observed on the growth of the methanogens and biogas produced in the reactors. In relation to the control digestate, the pH reduced to 4.8 ± 0.4 which resulted in the minimal growth and metabolic activities of the methanogens and hence biogas production. In addition, the difference in the pH of the digestate ranged between 2.2 (male urine) and 3.1 (female/male urine) and was significant at p = 0.05.
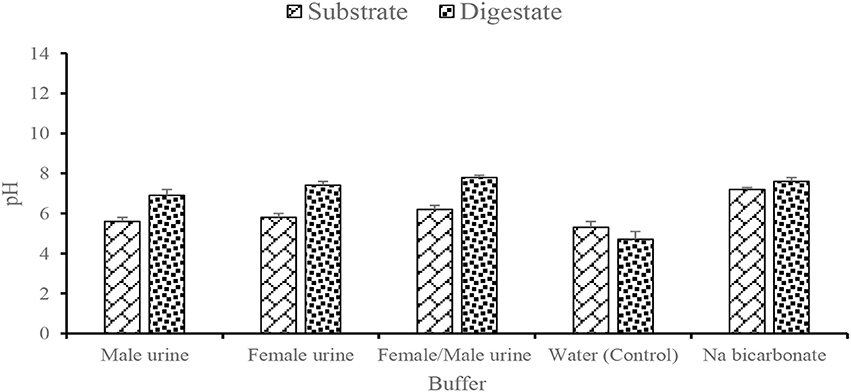
Figure 3. Effect of different buffering agents on the pH of substrate used and digestate produced from the BMP assay.
The plausible reason we deduced for the observed effect was that the hydrolysed urine contributed to the stable reactor performance as a buffering agent. Indeed, the hydrolysed urine has a high buffering capacity (Andreev et al., 2017) and therefore minimized the inhibitory effect of accumulated VFAs to the methanogens (Nicol et al., 2004; Franke-Whittle et al., 2014; Eduok et al., 2017). Although acid pre-treatment is a low cost and efficient process in hemicellulose hydrolysis to release monomeric sugars, corrosive and toxic compounds are produced (Talebnia et al., 2010; Ferreira et al., 2013), whereas alkaline pre-treatment of lignocellulosic biomass with hydroxides of ammonia, potassium, calcium and sodium modifies the structure and solubilize lignin with minimal inhibition of biogas production (Ferreira et al., 2013; Krishania et al., 2013). In contrast, the use of urine as a buffer can be compared to a combined alkaline treatment and ammonia fiber explosion (AFEX) with positive effect on the AD performance and biogas production.
At the moment, the mechanistic structure of the bond cleavage and continuous release of the fermentable sugars (cellulose) for microbial use and subsequent biogas production is unclear. From the result of the biogas generation, it appears that the biological and chemical components of the female urine in relation to that of the poultry feces, and probably the physicochemical constituent of the lignocellulosic biomass exerted either an additive, synergistic and/or potentiation effects or a combination of any two or all effects on the cellulose-hemicellulose-lignin bond cleavage to release the cellulose. For example, apparently healthy female genital microbiota is lactobacilli-dominated involved in regulatory and immune functions (Witkin et al., 2007; O'Hanlon et al., 2013) and poultry feces includes the lactic acid bacteria (Nazef et al., 2008) which are implicated in lignocellulosic biomass breakdown (Boguta et al., 2014). Specifically, Lactobacillus and Pediococcus strains from female genital microbiota were implicated in the bioconversion of lignocellulosic biomass. These strains were also able to resist common inhibitory substances such as the phenolic compounds, acetate, and furfurals from pre-treated lignocellulosic biomass (Boguta et al., 2014). It is possible that the presence of these organisms in the female urine enhanced the conversion of the substrate to simple sugars for biogas production. In addition, component of the hydrolysed female urine probably stimulated growth of the microorganisms associated with catalysis of the rate-limiting step of anaerobic digestion (hydrolysis) compared to the male urine and water. The result does not rule out the presence or influence of different components in the sawdust, poultry feces, and urine that played a role in the digestion and biogas production. Other confounding factors that could have exerted synergistic effect on the microbial activity and reactor performance include yeast in female urine and probably the hormones in male and female urine. The result from the female/male buffered reactor suggests a complimentary interaction that resulted in a synergistic or additive effect on the methanogenic activity and biogas production. What is not established is whether other components of the urine apart from the microorganisms played any role but it does suggest that our perception of urine that is free from pharmaceutical loading as waste maybe compositionally flawed and its potential effect on microorganisms and in particular, the methanogens has been so far underestimated.
Effect on Volatile Fatty Acids (VFAs) Accumulation Over 180 Days
The total VFAs concentration in the digestate was 1,400 mg L−1 (male), 1,098 mg L−1 (female), 396 mg L−1 (Female + Male), 386 mg L−1 (Na bicarbonate), and 3,109 mg L−1 (water) with a difference ranging between 2 and 8 times higher in control (water) than the urine buffered digestate (Figure 4). This difference was significant at p = 0.05. However, the ΣVFAs in the female/male urine buffered digestate was 1.02 times higher than in the sodium bicarbonate buffered digester. The main VFAs in the digestate were acetic and propionic acids (24 and 32%, respectively of the maximum total VFAs at 180 day), whereas the other VFA concentration ranged between 9% (n-valeric acid) and 13% (n-butyric acid). In the female/male urine buffered digestate, the VFA concentrations were below 100 mg L−1 similar to the concentration in the sodium bicarbonate buffered digestate and indicates enhanced microbial conversion of the metabolites to biomass and biogas.
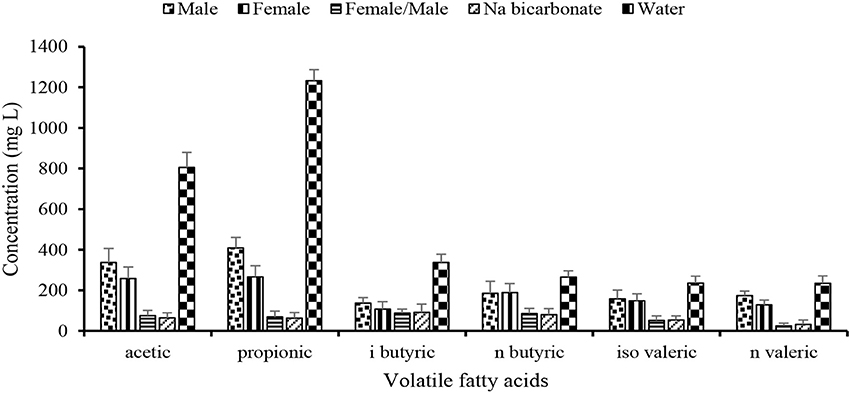
Figure 4. Effect of human urine on the volatile fatty acid accumulated during lignocellulosic biomass and poultry manure anaerobic co-digestion. Reduced concentration of the VFAs in the urine buffered digestate in relation to the control indicates stable reactor performance and conversion of substrate by the methanogens. Error bars denote standard deviation.
The lower concentration of acetic acid compared to propionic acid suggests that the archaeal community was dominated by aceticlastic than hydrogenotrophic methanogens. Further to this, the result indicates that propionic acid accumulated and its oxidation was reduced in the control because of low abundance of hydrogenotrophic methanogens, whereas acetic acid was readily converted by aceticlastic methanogens (Figure 4).
The propionic:acetic acid ratio with the value of 1.4 is required to create unstable condition during AD process (Hill et al., 1987) and was not exceeded in the urine buffered digesters. For instance, the ratio of propionic:acetic acid in the urine buffered digestate was 1.2 (male), 1.03 (female), 0.92 (Female/male) compared to 0.97 (sodium bicarbonate) and 1.5 in the negative control (water). The result indicates that VFAs accumulated in the control digestate and resulted in the reduced pH with a negative influence on the AD process performance, whereas the urine similar to the sodium bicarbonate served as a buffer and mitigated the inhibitory effect exerted by the VFAs in the digesters. The result corroborates the findings from other studies (Nicol et al., 2004; Franke-Whittle et al., 2014; Eduok et al., 2017) in which high concentration of VFAs was unable to cause AD process imbalance in a buffered system. Thus, the use of source separated human urine with no pharmaceutical loadings in anaerobic co-digestion is a promising option for a balanced reactor performance to enhance biogas production.
Effect on Methanogenic Microbial Diversity and Abundance
The PLEL markers associated with the archaeal community in the digestate included the saturated (i15:0, i20:0, i40:0, and i40:0-1cy) and unsaturated (i20:1) isoprenoids (Figure 5). The total PLEL concentration in the urine buffered reactors ranged between 55 and 155 μg g−1 compared to 26 μg g−1 in the control reactor. The result indicates that the PLEL concentration was 2–6 times higher in the urine buffered digestate than that in the control and these differences were significant at p = 0.05.
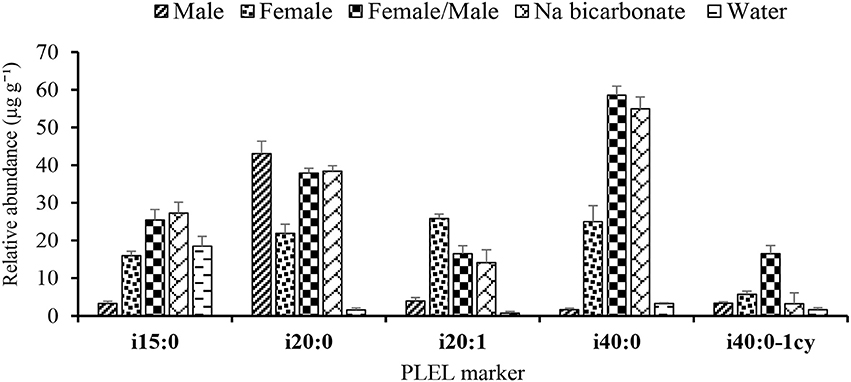
Figure 5. Phospholipid etherlipid (PLEL) markers of methanogenic Archaea in the digestate. Varying concentrations of saturated (i15:0, i20:0, i40:0, and i40:0-1cy) and unsaturated (i20:1) isoprenoids indicate the archaeal community composition and diversity in the digestate. Error bars denote standard deviation of FAME concentration.
A higher abundance of Archaea PLEL markers identified as aceticlastic methanogens (i15:0, i20:0, i40:0, and i40:0-1cy) and hydrogenotrophic methanogens (i40:0) was recovered in the urine buffered digestate in relation to the low abundance observed in the control reactor. Further to this, biogas production which directly relates to the methanogenic activity and hence PLEL concentrations in the digesters evidenced a strong positive correlation (r = 0.85) with the pH. For instance, the control (water) digestate with the lower pH (4.8 ± 0.4) and total VFAs of 3,109 mg L−1 had ΣPLEL concentration of 26 μg g−1. In contrast, the near alkaline pH of 7.6 ± 0.2 with total VFAs of 396 mg L−1 and ΣPLEL of 155 μg g−1 in the female/male urine spiked digestate indicates the buffering effect on the methanogens and AD process performance. In terms of PLEL abundance, 58% was similar in all the treatments and reflected the common source of the methanogens (Figure 6).
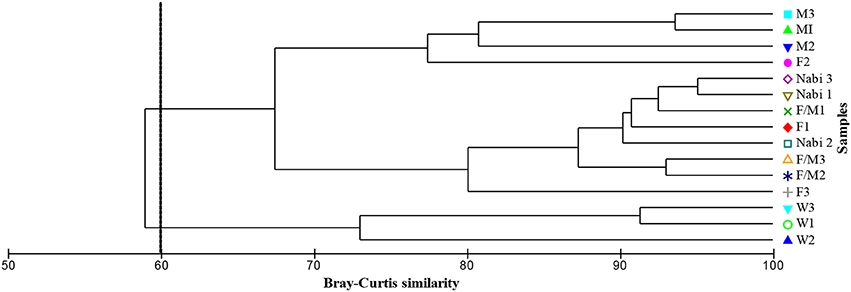
Figure 6. Dendrogram of the Archaeal PLEL markers influenced by different buffers in the digestate based on group-averaged clustering from Bray-Curtis similarities. The relative abundance of PLEL content was 94.67% similar in the sodium bicarbonate and female/male urine buffered digestate, and 58.10% in the water (W), male (M), female (F), female/male (F/M), sodium bicarbonate (Nabi) buffered digestate (1, 2, and 3 denote sample number).
On the other hand, the relative abundance of PLEL markers in the female/male buffered digestate had 95% resemblance to that in the sodium bicarbonate buffered digestate and indicates that the treatments influenced the growth and proliferation of methanogens and provided a stable, non-toxic condition for microbial metabolism in the reactor. Indeed, the combined female/male urine exerted a buffering effect similar to the sodium bicarbonate and in addition, increased the growth of methanogenic microorganisms. Overall, the PLEL marker in the urine buffered digestate was higher than in the control digestate and directly correlates with the increased volume of biogas produced.
Conclusion
The chemical composition of source separated human urine served as a wetting and buffering agent for enhanced biogas production during anaerobic co-digestion of lignocellulosic biomass and poultry feces. The empirical evidence indicates that the poultry feces in addition to the cow dung provided a mixture of microorganisms that were able to use most of the metabolic intermediates of the AD process. In addition, the source separated urine hydrolysed during the anaerobic process provided a stable reactor performance, near alkaline pH in the digestate, induced growth and proliferation of methanogens that resulted in low VFAs content and high biogas production. In terms of biogas production, the outcome in the reactor with the mixed female/male urine buffer was additive. Thus, the combined female/male urine as wetting/buffering agent offered a competitive edge over the other treatments and is as a promising option for enhanced biogas production from lignocellulosic biomass.
Author Contributions
SE, OJ, BI, and EI have contributed equally to the experimental work. SE was also involved in the experimental design of the work. FC was involved in the experimental setup, the critical review, and editing stage of the manuscript.
Conflict of Interest Statement
The authors declare that the research was conducted in the absence of any commercial or financial relationships that could be construed as a potential conflict of interest.
The reviewer BV and handling editor declared their shared affiliation.
Acknowledgments
The authors are grateful to Marvel MPCS for financial support through grant MMPCS/2017/A0133.
References
Akpan-Idiok, A. U., Udo, I. A., and Braide, E. I. (2012). The use of human urine as an organic fertilizer in the production of okra (Abelmoschus esculentus) in South Eastern Nigeria, Resources. Conserv. Recycl. 62, 14–20. doi: 10.1016/j.resconrec.2012.02.003
Andreev, N., Ronteltap, M., Boincean, B., Wernli, M., Zubcov, E., Bagrin, N., et al. (2017). Lactic acid fermentation of human urine too improve its fertilizing value and reduce odour emissions. J. Environ. Manage. 198, 63–69. doi: 10.1016/j.jenvman.2017.04.059
Angelidaki, I., Alves, M., Bolzonella, D., Borzacconi, I., Campos, J. I., Guwy, A. J., et al. (2009). Defining the biomethane potential (BMP) of solid organic wastes and energy crops: a proposed protocol for batch assays. Wat. Sci. Technol. 59, 927–934. doi: 10.2166/wst.2009.040
APHA. (2005). Standard Methods for Examination of Waste and Wastewater. Washington, DC: American Public Health Association.
Appels, L., Baeyens, J., Degreve, J., and Dewil, R. (2008). Principles and potential of the anaerobic digestion of waste-activated sludge. Prog, Energy Combust. Sci. 34, 755–781. doi: 10.1016/j.pecs.2008.06.002
Badshah, M., Lam, D. M., Liu, J., and Mattiasson, B. (2012). Use of an automatic methane potential test system for evaluating the biomethane potential of sugarcane bagasse after different treatments. Biores Technol. 114, 262–268. doi: 10.1016/j.biortech.2012.02.022
Bai, Q., Gattinger, A., and Zelles, L. (2000). Characterization of microbial consortia in paddy rice soil by phospholipid analysis. Microbial Ecol. 39, 273–281. doi: 10.1007/s002480000020
Balat, M. (2011). Production of bioethanol from lignocellulosic materials via the biochemical pathway. Energy Convers. Manage. 52, 858–885. doi: 10.1016/j.enconman.2010.08.013
Bals, B., Rogers, C., Jin, M., Belan, V., and Dale, B. (2010). Evaluation of ammonia fibre expansion (AFEX) pretreatment for enzymatic hydrolysis of switchgrass harvested in different seasons and locations. Biotechnol. Biofuels 3:1 doi: 10.1186/1754-6834-3-1
Barton, F. E. (1988). Chemistry of lignocellulosic: methods of analysis and consequence of structure. Animal Feed Sci. Technol. 21, 279–286. doi: 10.1016/0377-8401(88)90107-1
Boguta, A. M., Bingal, F., Martinussen, J., and Jensen, P. (2014). Screening of lactic acid bacteria for their potential as microbial cell factors for bioconversion of lignocellulosic feedstocks. Microbial Cell Factories 13:97. doi: 10.1186/s12934-014-0097-0
Bolado-Rodriquez, S., Toquero, C., Martin-Juarez, J., Travaini, R., and Garcia-Encina, P. A. (2016). Effect of thermal, acid, alkaline and alkaline-peroxide pretreatment on the biochemical methane potential and kinetics of the anaerobic digestion of wheat straw and sugar cane bagasse. Biores Technol. 201, 182–190. doi: 10.1016/j.biortech.2015.11.047
Clarke, K. R., and Warwick, R. M. (2001). Changes in Marine Communities: An Approach to Statistical Analysis and Interpretation. Plymouth: PRIMER-E.
Eduok, S., Ferguson, R., Jefferson, B., Villa, R., and Coulon, F. (2017). Aged-engineered nanoparticles effect on sludge anaerobic digestion performance and associated microbial communities. Sci. Tot Environ. 606, 232–241. doi: 10.1016/j.scitotenv.2017.07.178
Ekama, G. A., Wilsenach, J. A., and Chen, G. H. (2011). Saline sewage treatment and source separation of urine for more sustainable urban water management. Water Sci. Technol. 64, 1307–1316. doi: 10.2166/wst.2011.403
Ferreira, L. C., Donoso-Bravo, A., Nilsen, P. J., Fdz-Polanco, F., and Perez-Elvira, S. I. (2013). Influence of thermal pretreatment on the biochemical methane potential of wheat straw. Biores. Technol. 143, 251–257. doi: 10.1016/j.biortech.2013.05.065
Foyle, T., Jennings, L., and Mulcahy, P. (2007). Compositional analysis of lignocellulosic materials: evaluation of methods used for sugar analysis of waste paper and straw. Biores. Technol. 98, 3026–3036. doi: 10.1016/j.biortech.2006.10.013
Franke-Whittle, I. H., Walter, A., Ebner, C., and Insam, H. (2014). Investigation into the effect of high concentrations of volatile fatty acids in anaerobic on methanogenic communities. Waste Manage 34, 2080–2089. doi: 10.1016/j.wasman.2014.07.020
Gattinger, A., Günthner, A., Schloter, M., and Munch, J. C. (2003). Characterisation of Archaea in soils by polar lipid analysis. Acta Biotechnol. 23, 21–28. doi: 10.1002/abio.200390003
Gaur, R. Z., Khan, A. A., and Suthar, S. (2017). Effect of thermal pre-treatment on co-digestion of duckweed (Lemna gibba) and waste activated sludge on biogas production. Chemosphere 174, 754–763. doi: 10.1016/j.chemosphere.2017.01.133
Gaur, R. Z., and Suthar, S. (2017). Anaerobic digestion of activated sludge, anaerobic granular sludge and cow dung with food waste for enhanced methane production. J. Clean. Product. 164, 557–566. doi: 10.1016/j.jclepro.2017.06.201
Hagos, K., Zong, J., Li, D., Liu, C., and Lu, X. (2017). Anaerobic co-digestion process for biogas production: progress, challenges and perspectives. Renew. Sustain. Energy Rev. 76, 1485–1496. doi: 10.1016/j.rser.2016.11.184
Hill, D. T., Cobb, S. A., and Bolte, J. P. (1987). Using volatile fatty acid relationships to predict anaerobic digester failure. Trans. ASAE. 30, 496–501. doi: 10.13031/2013.31977
Jacobsen, S. E., and Wyman, C. E. (2000). Cellulose and hemicellulose hydrolysis models for application to current and novel pretreatment processes. Appl. Biochem. Biotechnol 84–86, 81–96. doi: 10.1385/ABAB:84-86:1-9:81
Jimenez, J., Bott, C., Love, N., and Bratby, J. (2015). Source separation of urine as an alternative solution to nutrient management in biological nutrient removal treatment plants. Water Environ Res. 87, 2120–2129. doi: 10.2175/106143015X14212658613884
Karagöz, P., Rocha, I. V., Ozkan, M., and Angelidaki, I. (2012). Alkaline peroxide pretreatment of rapeseed straw for enhanced bioethanol production by same vessel saccharification and co-fermentation. Biores Technol. 104, 341–357. doi: 10.1016/j.biortech.2011.10.075
Kayhanian, M. (1994). Performance of a high solid anaerobic digestion process under various ammonia concentrations. J. Chem. Technol. Biotechnol. 59, 349–352. doi: 10.1002/jctb.280590406
Krishania, M., Vijay, V. K., and Chandra, R. (2013). Methane fermentation and kinetics of wheat straw pretreated substrates co-digested with cattle manure in batch assay. Energy 57, 359–367. doi: 10.1016/j.energy.2013.05.028
Landry, K. A., and Boyer, T. H. (2016). Life cycle assessment and costing of urine source separation: focus on nonsteriodal anti-inflammatory drug removal. Water Res. 105, 487–495. doi: 10.1016/j.watres.2016.09.024
Larsen, T. A., Lienert, J., Joss, A., and Siegrist, H. (2004). How to avoid pharmaceuticals in the aquatic environment. J. Biotechnol. 113, 295–304 doi: 10.1016/j.jbiotec.2004.03.033
Lienert, J., and Larsen, T. A. (2010). High acceptance of urine source separation in seven European Countries: a review. Environ. Sci. Technol. 44, 556–566. doi: 10.1021/es9028765
Lin, Y., Lu, F., Shao, L., and He, P. (2013). Influence of bicarbonate buffer on the methanogenic pathway during thermophilic anaerobic digestion. Biores. Technol. 137, 245–253 doi: 10.1016/j.biortech.2013.03.093
Nazef, L., Belguesmia, Y., Tani, A., Prevost, H., and Drider, D. (2008). Identification of lactic acid bacteria from poultry feces: evidence on anti-Campylobacter and anti-Listeria activities. Poultry Sci. 87, 329–334. doi: 10.3382/ps.2007-00282
Nicol, G. W., Webster, G., Glover, L. A., and Prosser, J. I. (2004). Differential response of archaeal and bacterial communities to nitrogen inputs and pH changes in upland pasture rhizosphere soil. Environ. Microbiol. 6, 861–867. doi: 10.1111/j.1462-2920.2004.00627.x
O'Hanlon, D. E., Moench, T. R., and Cone, R. A. (2013). Vaginal pH and Microbicidal lactic acid when lactobacilli dominate the microbiota. PLoS ONE 8:e80074. doi: 10.1371/journal.pone.0080074
Owen, W. F., Stuckey, D. C., Healy, J. B. Jr., Young, L. Y., and McCarty, P. L. (1979). Bioassay for monitoring biochemical methane potential and anaerobic toxicity. Water Res. 13, 485–492. doi: 10.1016/0043-1354(79)90043-5
Paul, S., and Dutta, A. (2018). Challenges and opportunities of lignocellulosic biomass for anaerobic digestion. Res. Conserv. Recycl. 130, 164–174. doi: 10.1016/j.resconrec.2017.12.005
Sreenath, H. K., Koegel, R. G., Moldes, A. B., Jeffries, T. W., and Straub, R. J. (1999). Enzymic saccharification of alfalfa fibre liquid hot water pretreatment. Process Biochem. 35, 34–41. doi: 10.1016/S0032-9592(99)00029-1
Talebnia, F., Karakashew, D., and Angelidaki, I. (2010). Production of bioethanol from wheat straw: an overview on pretreatment hydrolysis and fermentation. Bioresourc. Technol. 101, 4744–4753. doi: 10.1016/j.biortech.2009.11.080
Torget, R. W., Kim, J. S., and Lee, Y. Y. (2000). Fundamental aspects of dilute acid hydrolysis of hardword carbohydrates. Cellulose hydrolysis. Ind. Eng. Chem. Res. 39: 2817–2825. doi: 10.1021/ie990915q
Valcke, D., and Verstraete, W. (1983). A practical method to estimate the acetolastic methanogenic biomass in anaerobic reactors. J. WPCF 55:1191.
Wagner, A. O., Gstrauntaler, G., and Illmer, P. (2010). Utilization of single added fatty acids by consortia of digester sludge in batch culture. Waste Manag. 30, 1822–1827. doi: 10.1016/j.wasman.2010.05.013
Keywords: lignocellulosic biomass, urine, anaerobic co-digestion, buffer, biogas
Citation: Eduok S, John O, Ita B, Inyang E and Coulon F (2018) Enhanced Biogas Production From Anaerobic Co-digestion of Lignocellulosic Biomass and Poultry Feces Using Source Separated Human Urine as Buffering Agent. Front. Environ. Sci. 6:67. doi: 10.3389/fenvs.2018.00067
Received: 09 February 2018; Accepted: 12 June 2018;
Published: 02 July 2018.
Edited by:
Rajeev Pratap Singh, Banaras Hindu University, IndiaReviewed by:
Abid Ali Khan, Jamia Millia Islamia, IndiaBarkha Vaish, Banaras Hindu University, India
Copyright © 2018 Eduok, John, Ita, Inyang and Coulon. This is an open-access article distributed under the terms of the Creative Commons Attribution License (CC BY). The use, distribution or reproduction in other forums is permitted, provided the original author(s) and the copyright owner(s) are credited and that the original publication in this journal is cited, in accordance with accepted academic practice. No use, distribution or reproduction is permitted which does not comply with these terms.
*Correspondence: Frédéric Coulon, Zi5jb3Vsb25AY3JhbmZpZWxkLmFjLnVr