- 1Leibniz-Institute of Freshwater Ecology and Inland Fisheries, Berlin, Germany
- 2Department of Biology, Chemistry and Pharmacy, Freie Universität Berlin, Berlin, Germany
- 3School of Geography, Queen Mary University of London, London, United Kingdom
- 4German Centre for Integrative Biodiversity Research, Leipzig, Germany
- 5Department of Civil, Environmental and Mechanical Engineering, Università degli Studi di Trento, Trento, Italy
Artificial light at night (ALAN) is a widespread alteration of the natural environment that can affect the functioning of ecosystems. ALAN can change the movement patterns of freshwater animals that move into the adjacent riparian and terrestrial ecosystems, but the implications for local riparian consumers that rely on these subsidies are still unexplored. We conducted a 2-year field experiment to quantify changes of freshwater-terrestrial linkages by installing streetlights in a previously light-naïve riparian area adjacent to an agricultural drainage ditch. We compared the abundance and community composition of emerging aquatic insects, flying insects, and ground-dwelling arthropods with an unlit control site. Comparisons were made within and between years using two-way generalized least squares (GLS) model and a BACI design (Before-After Control-Impact). Aquatic insect emergence, the proportion of flying insects that were aquatic in origin, and the total abundance of flying insects all increased in the ALAN-illuminated area. The abundance of several night-active ground-dwelling predators (Pachygnatha clercki, Trochosa sp., Opiliones) increased under ALAN and their activity was extended into the day. Conversely, the abundance of nocturnal ground beetles (Carabidae) decreased under ALAN. The changes in composition of riparian predator and scavenger communities suggest that the increase in aquatic-to-terrestrial subsidy flux may cascade through the riparian food web. The work is among the first studies to experimentally manipulate ALAN using a large-scale field experiment, and provides evidence that ALAN can affect processes that link adjacent ecosystems. Given the large number of streetlights that are installed along shorelines of freshwater bodies throughout the globe, the effects could be widespread and represent an underestimated source of impairment for both aquatic and riparian systems.
Introduction
The continuing global increase of artificial light at night (ALAN) and its effects on organisms and ecosystems have received considerable attention in recent years (Hölker et al., 2010). It has become clear that ALAN can affect the movement, reproduction, physiology and behaviour of animals (Longcore and Rich, 2004; Navara and Nelson, 2007; Perkin et al., 2011; Kurvers and Hölker, 2015; Honnen et al., 2016). Changes to animal movement have been reported in freshwater ecosystems, where ALAN can disrupt the diel vertical migration of zooplankton, arthropod drift (Bishop, 1969; Moore et al., 2001; Perkin et al., 2014b), and fish predation (Tabor et al., 2004). The attraction of terrestrial insects to ALAN light sources is well-documented (Eisenbeis et al., 2006) and it can have negative consequences (Horváth et al., 2009; Perkin et al., 2014a; Degen et al., 2016). Most research has focused on individual species, although there have been a few studies examining communities (e.g., Davies et al., 2012; Hölker et al., 2015; Holzhauer et al., 2015; Spoelstra et al., 2015; Grubisic et al., 2017), ecosystem functions (Knop et al., 2017) and ecosystems (Gaston et al., 2015).
Human populations are concentrated near water bodies (Kummu et al., 2011) and thus the impact of ALAN on ecosystems and organisms is of particular importance for freshwaters and the adjacent riparian ecosystems (Perkin et al., 2011). Aquatic and riparian ecosystems are connected by fluxes of energy and matter. For example, terrestrially derived carbon and nutrients support aquatic metabolism (Wallace et al., 1999; Mehner et al., 2005) while emerging aquatic insects and amphibians provide subsidies for a wide range of terrestrial consumers (Marczak and Richardson, 2007; Bartels et al., 2012). Aquatic-terrestrial subsidy fluxes can be reciprocal, benefitting consumers in both habitats (Polis et al., 1997; Nakano and Murakami, 2001; Richardson et al., 2010) while also contributing to food-web and ecosystem stability (Takimoto et al., 2002). By affecting the organisms involved, ALAN could alter the natural dynamics of these subsidy fluxes, with potentially widespread ecological effects. The few studies to investigate this possibility have suggested that riparian consumers may respond to the increased availability of prey when aquatic insects are attracted to light sources (Perkin et al., 2011; Meyer and Sullivan, 2013). A difficulty with previous studies has been the inability to disentangle the effects of ALAN from the many associated anthropogenic stressors such as urbanisation, sealing (paving) of the ground, increased noise, and chemical pollution (Perkin et al., 2011).
Experimental approaches can help to exclude one or more confounding factors, but they remain rare considering the costs associated with setting up realistic ecosystem-scale manipulations. Here, we report results from a large-scale field experiment in which we introduced commercial streetlights to a previously ALAN-naïve area to study the impact of ALAN on the aquatic-terrestrial linkage. Streetlights were installed along an agricultural drainage ditch and in the adjacent riparian areas at two sites: one site was illuminated at night during part of the experiment, while the other remained dark to serve as a control. This experimental setup controls for most confounding factors associated with urbanisation; moreover, installation of identical streetlights at both sites controls for the effects associated with the physical structure of the light poles. Pre- and post-illumination assessment (using a BACI design; see Methods) allowed us to more confidently ascribe observed ecological changes to the effects of ALAN. We quantified changes in (i) aquatic insect emergence, considered as a primary source of aquatic subsidies to the terrestrial system; (ii) the abundance of flying aquatic and terrestrial insects in the riparian environment; and (iii) the abundance and composition of day and night-active riparian ground-dwelling predator and scavenger communities.
Methods
Study Area
The field experiment was carried out using a large-scale experimental infrastructure fully described by Holzhauer et al. (2015). It is located in the Westhavelland Nature Park and within a 750-km2 International Dark-Sky Reserve that is one of the least illuminated areas in Germany (International Dark Sky Association (IDA), 2015). The area is characterized by an extensive system of agricultural drainage ditches (Figures 1A,B). In April 2012, two identically managed grassland areas with no prior exposure to ALAN were selected for a multiple-year experiment to study ecological impacts of ALAN. The two sites are separated by a Euclidean distance of ~600 m (800 m along the drainage ditch) and a row of trees. Both sites were equipped with three parallel rows (3, 23, and 43 m away from the water) of four conventional 4.75 m high streetlights located 20 m apart (Figure 1C) and with one 70-W high-pressure sodium lamp each (OSRAM VIALOX NAV-T Super 4Y). Maximum illuminance was ~50 lux, minimum illuminance between two rows of street lamps was around 1 lux and minimum illuminance between two adjacent street lamps of the same row was around 10 lux (see Holzhauer et al., 2015 for further details about light distribution and spectral composition). Ecological monitoring started at the beginning of May 2012, prior to any illumination. From July 25 onward, one site (the Treatment) was illuminated at night, i.e., one set of streetlights was switched on between civil twilight at dusk and dawn. The Control site remained dark, yet provided identical physical structure (see Holzhauer et al., 2015 for further details).
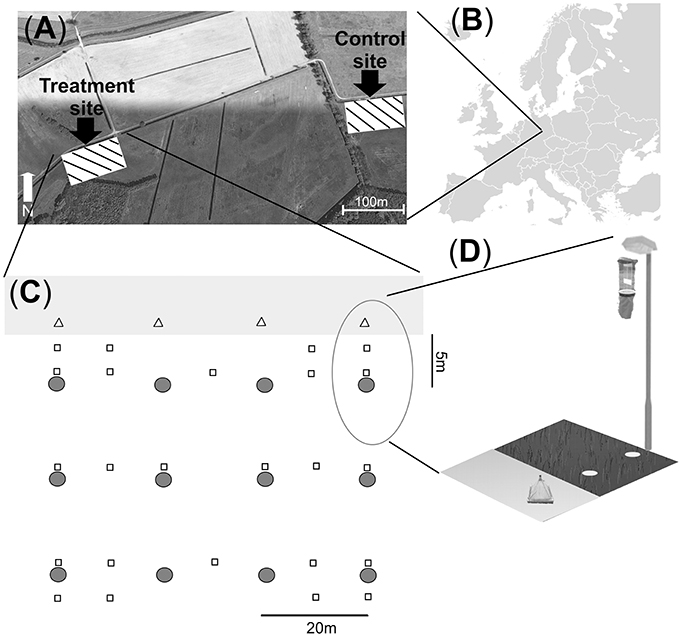
Figure 1. Study area in the Westhavelland (Brandenburg; 52° 41′ 29.81″ N, 12° 27′ 37.54″ E) depicting Treatment and Control sites (each 60 × 40 m) located along an agricultural drainage ditch (A,B). The lower panels (C,D) depict the Treatment site with streetlamps and sampling traps. Floating pyramidal emergence traps (triangles, n = 4) were placed adjacent to a lamp on the water surface of the drainage ditch. Air eclector traps were mounted below each lamp (grey circles, n = 12). Pitfall traps (quadrats, n = 24) were placed on the ground in multiple locations. The structural design of the Control site was identical to the Treatment site, yet streetlights were not switched on. Map data by Google Earth Pro (2011)1 (A) and Wiki-vr (https://en.wikipedia.org/wiki/File:Europe_blank_map.png) (B).
Environmental Conditions
Data collection started in June 2012. Weather stations at both sites continuously recorded air temperature and humidity every 15 min. Underwater probes continuously recorded water temperature and dissolved oxygen in the drainage ditches. These data were used to ascertain continuous chemico-physical similarity between the two sites and obtain reference (baseline) values in the absence of illumination at the Treatment site (Holzhauer et al., 2015).
Arthropod Collection and Identification
Insects were collected from both sites using identical procedures. Emerging aquatic insects were sampled using four floating pyramidal emergence traps (0.85 × 0.85 m, 300-μm mesh) at each site. These were placed in the drainage ditch adjacent to the bank and one trap was placed directly in front of each streetlamp (Figures 1C,D). Sampling was continuous for 128–192 h but frequency varied according to emergence patterns. In 2012, sampling occurred weekly from May to August and monthly in September and October. In 2013, sampling occurred monthly in May and June and weekly in July. Aquatic and terrestrial flying insects were collected using 12 air eclector traps at each site consisting of two perpendicular acrylic panels (each 204 × 500 × 3 mm) mounted above a collecting funnel and placed 0.5 m below each lamp (Figures 1C,D). Ground-dwelling arthropods were collected using 24 pitfall traps at each site, each consisting of a container (15-cm diameter) inserted in the ground with its rim at the soil surface. A transparent acrylic sheet was placed above each trap to prevent entry of precipitation and debris. Pitfall traps were positioned under and between streetlights at varying distances from the drainage ditch (Figure 1C). Air eclector and pitfall traps were active for one 24-h sampling period every 2 weeks from May to October 2012 and May to July 2013. Sampling always occurred on rainless nights within one night of each half-moon phase (first and third quarter). In 2012, sampling was carried out at night and lasted from astronomical sunset to sunrise (8–14 h depending on the season). In 2013, pitfall trap sampling was also conducted during the day (10–16 day-time hours) following the night sampling. All traps were fitted with collecting containers with 70% ethanol for preservation (see Holzhauer et al., 2015 for further details). In 2012 sheet weaver spiders, harvestmen and only the most common wolf spiders and long-jawed spiders (e.g., Pardosa prativaga, Pachygnatha clerki) were identified at the species or genus level (see taxa list in Appendix S3). Other taxonomic groups were identified at family or order level (see Appendix S3). In 2013 species level was reached for all wolf spiders and ground beetles (see taxa list Appendix S3). Identification was conducted under a binocular microscope and using taxonomical literature (Roberts, 1996; Schaefer, 2010; Stresemann, 2011). Species that have a larval phase in the water were considered as aquatic. Larval individuals were excluded from counts.
Experimental Approach
The experiment was set up as a BACI design (Before-After, Control-Impact) (Stewart-Oaten et al., 1986), testing for differences in arthropod abundance between the dark Control site and the Treatment site, before and after the exposure to artificial light (BACI phases). An un-replicated BACI design such as this assesses how a system reacts to perturbation in comparison to a simultaneously studied unperturbed control system (Stewart-Oaten et al., 1992). In our case, the problem of un-replicated perturbation was addressed in four ways: (i) an effect of ALAN is indicated by a significant interaction of the factor “site” (i.e., Control/Treatment) with “phase” (i.e., before/after perturbation) rather than by a direct difference (i.e., main effect) between the two sites; (ii) mean sample values at each site at any point in time are reliably estimated by extensive replication using multiple traps; (iii) multiple sampling occasions in the before and after phases provide reliable means for both phases and the necessary degrees of freedom for the statistical tests; and (iv) potential confounding effects are excluded by striving for similarity in environmental conditions (other than those directly related to ALAN) across sites and assessing stability of this similarity (or persistence of any dissimilarity) between phases. In other words, for the identification of an ALAN effect, there must be a persistent difference between phases at the Treatment site that is larger than any measured changes at the Control site, while other environmental variables should show minimal variation i.e. sites must remain environmentally similar (or similarly different) at any point in time.
Between May and July 2012 both sites were kept dark, representing the period before ALAN treatment. Illumination then began at the Treatment site in July 2012 and continued until the end of the study in July 2013. We made two statistical comparisons: The first one compared the unlit and lit periods in 2012, i.e. May-July 2012 with August-October 2012. Since this comparison mainly tests for changes within the same year, we refer to it as BACI-acute. As this comparison could be confounded by site-specific changes in phenology, we ran a second comparison that considered data from both years during the same season and compared the unlit period from May-July 2012 with the lit period from May-July 2013. Since this comparison tests for changes from one year to the next, we refer to it as BACI-chronic. Statistical aspects of both comparisons are described in detail below.
In addition to the BACI design we conducted a circadian analysis during the After 2013 phase (May to July 2013) when day-time data were collected. This allowed us to assess differences in abundance of ground-dwelling secondary consumers between diurnal and nocturnal communities. This was done to assess changes in the timing of activity due to ALAN, such as increased nocturnal activity of predominantly diurnal species and vice versa.
Statistical Analysis—Environmental Conditions
In accordance with the experimental BACI design (see above), differences among Control/Treatment sites and Before/After phases were assessed for each environmental parameter using two-way Generalized Least Squares (GLS) models with factors “site” (Control, Treatment), “phase” (Before 2012 and After 2012 for BACI-acute; Before 2012 and After 2013 for BACI-chronic) and their interaction. GLS were ran using the nlme package (Pinheiro et al., 2015) for R (version 3.3.1; R Core Team, 2015). The analysis included an autoregressive correlation structure (corARMA) to account for serial correlation of time series data. The data autocorrelation was tested for each environmental parameter using Durbin-Watson statistics in the car package (Fox et al., 2016) for R. The correlation structure suitability for each model was tested using a likelihood-ratio test (see Holzhauer et al., 2015 for a similar approach).
For each GLS with a significant interaction, we performed contrast analysis as pairwise comparison using least-squares means (LSM) with the lsmeans package (Lenth, 2016) for R. Pairwise comparison were performed between Control and Treatment site within the same phase, and between two different phases within the same site.
Statistical Analysis—Arthropods
For all analyses, arthropod abundance was standardised to the number of individuals caught per hour of trap operation (CPUE; catch per unit effort). High levels of precipitation and flooding caused malfunction of some pitfall traps in 2013 and we therefore only used data from positions where traps had remained functional at both sites. Only night-time samples were analysed for the comparisons of ground-dwelling secondary consumers, because daytime data were not collected in 2012. Because traps were spatially dependent, we calculated the mean CPUE of all traps at each site for each sampling occasion. Replication for the statistical tests was provided by multiple observations at each site and phase.
For the BACI comparisons (acute and chronic), differences in arthropod abundance (as CPUE) for each trap type (emergence, air eclector, pitfall) were examined using GLS with an autoregressive correlation structure (corARMA) and with factors “site” and “phase” and their interaction (see above). This was similar to the analysis of the environmental conditions (see above). For the analysis of air eclector traps, where both terrestrial and aquatic insects were caught, the same model was also run to assess difference in proportion of aquatic insects (on the total). For the analysis of the pitfall traps, taxa were grouped into 13 larger taxonomical units (see Table 2). This was done in order to compare communities from 2012 to 2013 samplings in which different levels of identification were reached (see above). Subsequently, the same GLS (see above) was run for each of these taxa (see Table 2). This was done in order to observe taxa-specific effects of ALAN.
The effect of ALAN was also assessed on the circadian activity of ground-dwelling secondary consumers in the After 2013 phase (May–Jul 2013), where both day-time and night-time samples were collected. Two-way GLS were used with an autoregressive correlation structure (corARMA) and with factors “site” and “time of the day” (day, night) and their interaction. These GLS models were performed in parallel on the same taxa analysed with the BACI design (see above) in order to identify taxa-dependent effects of ALAN (see Table 3).
As for the analysis of the environmental conditions, LSM was used to perform pairwise contrast analysis for each GLS with a significant interaction. To control for inflated false discovery rates, we used Benjamini-Hochberg corrected p-values for the pairwise contrast analyses (Waite and Campbell, 2006). The distribution of residuals was assessed using Wilk-Shapiro tests (Shapiro and Wilk, 1965) and quantile-plots (Wilk and Gnanadesikan, 1968). Arthropod CPUE was log(X + 1)-transformed to meet the assumption of normality.
Results
Environmental Conditions
Throughout the experiment, air temperature and humidity did not differ among sites and phases (Appendix S1, S2) while mean daily water temperature was slightly higher at the Control than at the Treatment site by 1.8°C in the period prior to illumination (Before 2012), 0.8°C during illumination in 2012 (After 2012) and 1.3°C in 2013 (After 2013). Dissolved oxygen was 0.7 mg l−1 and 1.32 mg l−1 higher at the Treatment site prior to illumination in 2012 and during illumination in 2012, respectively. However, it was 1.5 mg l−1 lower at the Treatment site in 2013 (Appendix S1, S2).
Aquatic Insect Emergence
We collected 25 families of insects in emergence traps. Most individuals belonged to the Ephemeroptera (Baetidae, Cloeon dipterum), Trichoptera (5 families) or Diptera (17 families) (Taxon list in Appendix S3). The model of comparison BACI-acute found no significant difference in emergence among phases and sites, i.e. before and after ALAN treatment in 2012 (Table 1, Figure 2). The model of comparison BACI-chronic indicated constantly higher insect abundance (as CPUE) at the Treatment site when this was illuminated (Table 1, Figure 2). Insect CPUE at the Treatment site during illumination in 2013 was 2-fold higher than at the Control site and 3-fold higher compared to itself prior to illumination in 2012 (Table 1, Figure 2). No difference in CPUE were found at the Control site throughout the experiment. (Table 1, Figure 2).
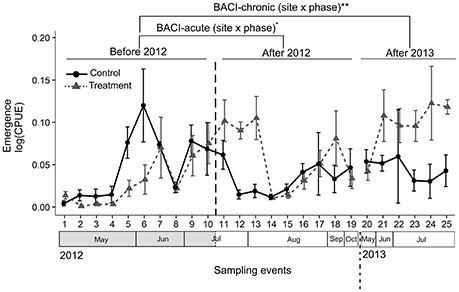
Figure 2. Abundance of emerging aquatic insects (CPUE) was compared across sampling events within and among years using a BACI design. Control and Treatment sites were first compared prior to and during experimental illumination in 2012 (BACI-acute; Before 2012 and After 2012). Sites were then compared prior to illumination in 2012 with the same period of the year in 2013, after experimental illumination (BACI-chronic; Before 2012, After 2013). Significant site × phase interactions (GLS) are shown as **p < 0.01; ·0.05 < p < 0.07. Means across all traps with standard errors are shown for the two sites at each sampling event.
Flying Insects
We collected a total of 189 aquatic and terrestrial taxa in the air eclector traps. The majority of aquatic insects were Ephemeroptera and Diptera and the majority of terrestrial insects were Lepidoptera and Coleoptera (see Appendix S3 for a taxon list). Both GLS models (comparisons BACI-acute, -chronic) indicated higher abundance of aquatic insects at the Treatment site when this was illuminated (Table 1, Figure 3A). In 2012 (comparison BACI-acute) the lit Treatment site had 76-fold higher CPUE than the Control site, and 70-fold higher CPUE compared to itself prior to illumination (Table 1, Figure 3A). There was no change in aquatic insect abundance at the Control site throughout the experiment and no differences between the Control and the Treatment site were found prior to illumination (Figure 3A). In Comparison BACI-chronic the lit Treatment site in 2013 had 267-fold higher CPUE than the Control site and 918-fold higher CPUE compared to itself prior to illumination (Table 1, Figure 3A). There was no change in aquatic insect abundance at the Control site throughout the experiment and no differences between the Control and the Treatment site were found prior to illumination. (Figure 3A). For flying terrestrial insects, only the model of comparison BACI-acute in 2012 indicated differences among sites and phases (Table 1, Figure 3B). Terrestrial flying insect CPUE at the lit Treatment site was 34-fold higher than the Control site, and 81-fold higher CPUE compared to the same site prior to illumination (Table 1, Figure 3B). There was no difference between sites prior to illumination or within the Control site before and after illumination in 2012 (Figure 3B).
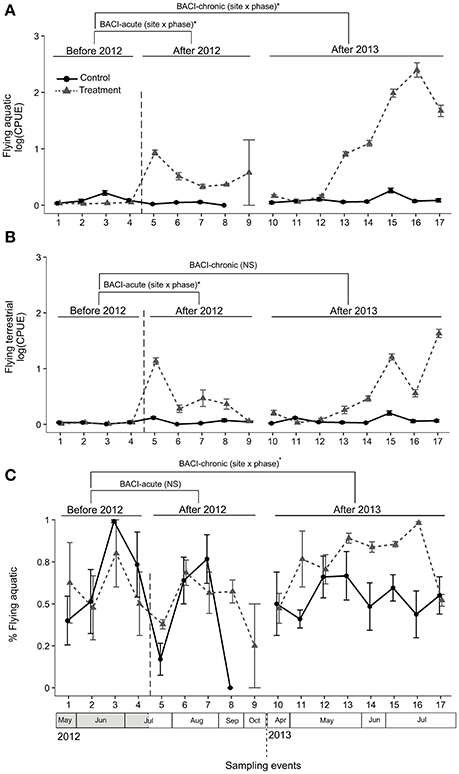
Figure 3. Abundance (CPUE) of adult flying aquatic (A) and terrestrial (B) insects and proportion (%) of aquatic insect on the total (C) were compared across sampling events within and among years using a BACI design. Control and Treatment sites were first compared prior to and during experimental illumination in 2012 (BACI-acute; Before 2012 and After 2012). Sites were then compared prior to illumination in 2012 with the same period of the year in 2013, after experimental illumination (BACI-chronic; Before 2012, After 2013). Significant site × phase interactions (GLS) are shown as *p < 0.05; ·0.05 < p < 0.07; NSp > 0.07. Means across all traps with standard errors are shown for the two sites at each sampling event.
The proportion of arthropods that were aquatic in origin did not differ among sites and phases in 2012 (comparison BACI-acute) (Table 1, Figure 3C), but differed in the comparison between years (comparison BACI-chronic) (Table 1, Figure 3C). In 2013, aquatic insects at the Treatment site comprised 75% of the total catch, compared to 54% at the Control site in the same year (i.e., ~1.5-fold greater) and 58% at the Treatment site prior to illumination (i.e., ~1.4-fold greater) (Table 1, Figure 3C). There was no difference in the proportion of aquatic insects between Control and Treatment sites in 2012 prior to illumination, nor between years at the Control site (Figure 3C).
Ground-Dwelling Arthropods
We collected a total of 135 taxa of ground-dwelling arthropods in the pitfall traps (see Appendix S3 for a taxon list) that were grouped into 13 taxa for the analyses (see Table 2). For primary consumers, there was no significant variation in CPUE among sites and phases in either comparisons BACI-acute or BACI-chronic (Table 1, Figure 4A). For secondary consumers, there was no difference in CPUE among sites and phases in the comparison between phases within 2012 (comparison BACI-acute). In the comparison BACI-chronic between 2012 and 2013 (Table 1, Figure 4B) 1.3-fold lower CPUE was found at the lit Treatment site than at the Control site in 2013, while the lit Treatment site had 6.5-fold higher CPUE than the Treatment site prior to illumination (Table 1, Figure 4B).
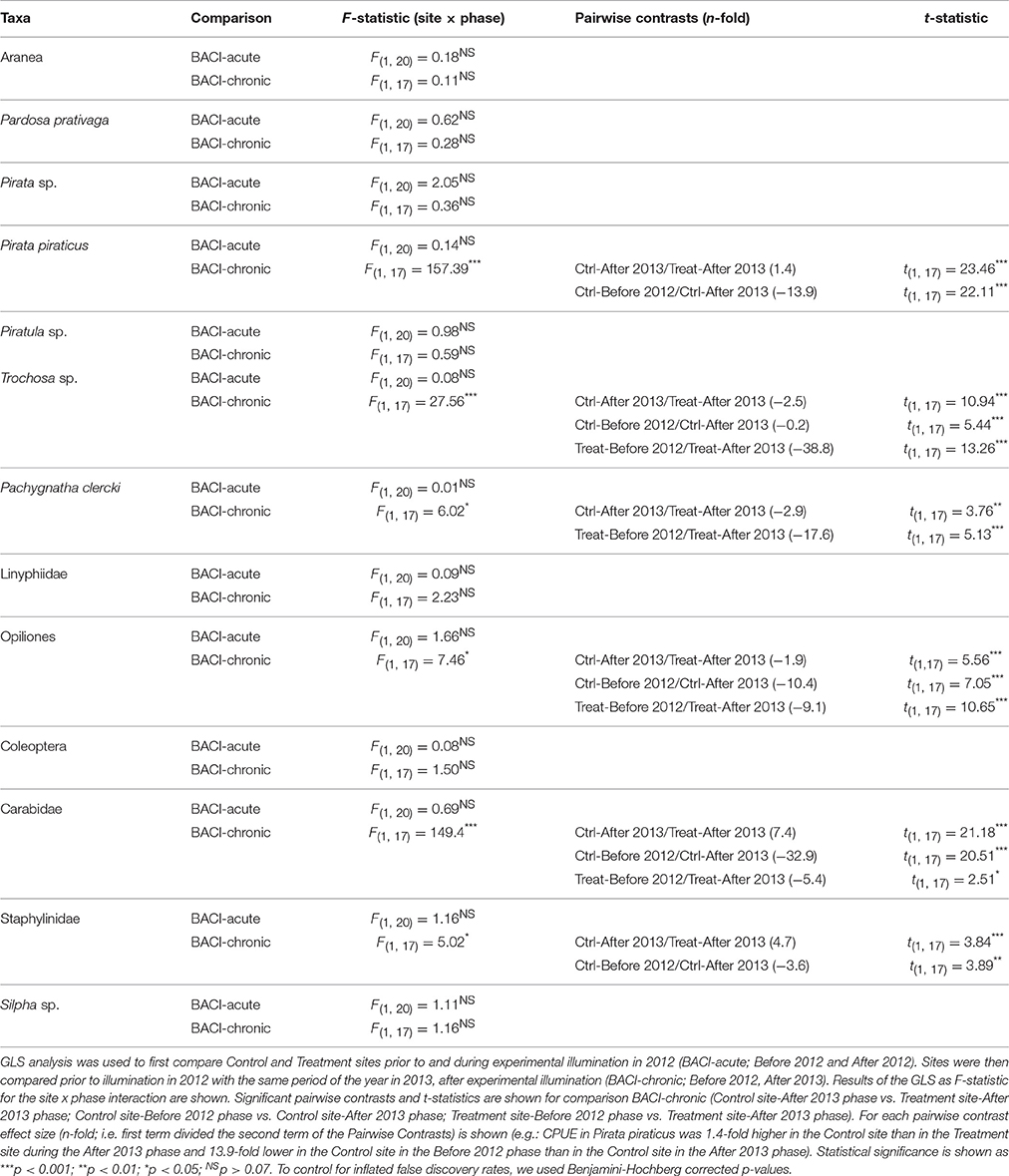
Table 2. Arthropod abundance (CPUE) analysed using a BACI design for 13 taxa of ground-dwelling secondary consumers (from pitfall traps) taxonomically identified in both 2012 and 2013 samplings (see Appendix S3).
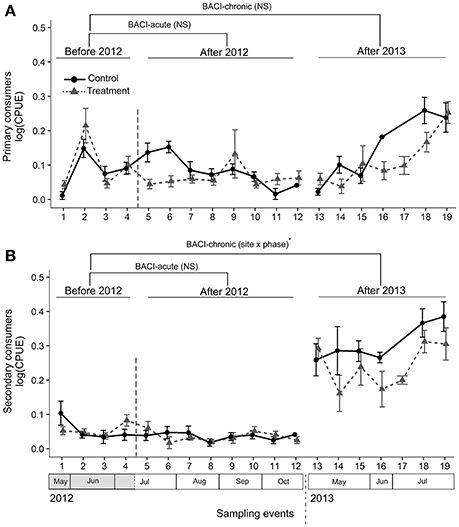
Figure 4. Abundance (CPUE) of ground-dwelling primary (A) and secondary (B) consumers was compared across sampling events within and among years using a BACI design. Control and Treatment sites were first compared prior to and during experimental illumination in 2012 (BACI-acute; Before 2012 and After 2012). Sites were then compared prior to illumination in 2012 with the same period of the year in 2013, after experimental illumination (BACI-chronic; Before 2012, After 2013). Significant site × phase interactions (GLS) are shown as ·0.05 < p < 0.07; NSp > 0.07. Means across all traps with standard errors are shown for the two sites at each sampling event.
GLS performed on each of the 13 taxa comprising the community of ground-dwelling secondary consumers showed taxa-specific difference in CPUE among sites and phases between 2012 and 2013 (comparison BACI-chronic). In 2013, Trochosa sp. (a wolf spider), Pachygnatha clercki (a long-jawed spider) and Opiliones, were more abundant at the lit Treatment site than at the Control site (Table 2; Appendix S4A–C). Conversely, Pirata piraticus (a wolf spider), Carabidae (ground beetles) and Staphylinidae (rove beetles), were more abundant at the Control site (see Table 2, Appendix S4D–F). Taxa that differed between Treatment and Control in 2013, also differed between post- and pre-illumination periods in the Treatment site (P. clerki), in the Control site (P. piraticus, Staphylinidae) and in both Treatment and Control sites (Trochosa sp., Opiliones, Carabidae) (see Table 2, Appendix S4).
The analysis of the circadian activity shows taxa-specific differences in CPUE between Control and Treatment site and day-/night-time samplings in 2013 (May-Jul, After 2013 phase). CPUE in Trochosa sp. was 7-fold higher at night than at day at the Treatment compared to the Control site where it was 4-fold-higher (Table 3, Figure 5A). Pachygnatha clercki, Opiliones (harvestmen), and Silpha sp. (a carrion beetle) were more abundant in the Treatment site than in the Control site (significant “site” effect). This was true for both day and night sampling (Table 3, Figures 5B–D). In contrast, CPUE in Carabidae at the Treatment site was 5-fold lower at night than at day (significant “site × “time” interaction), while CPUE at the Control site at day and night was similar. CPUE in P. piraticus was 12-fold higher at night than at day at the Control site (significant “site × “time” interaction), while no difference in CPUE between day and night sampling was found at the Treatment site (Table 3, Figure 5E).
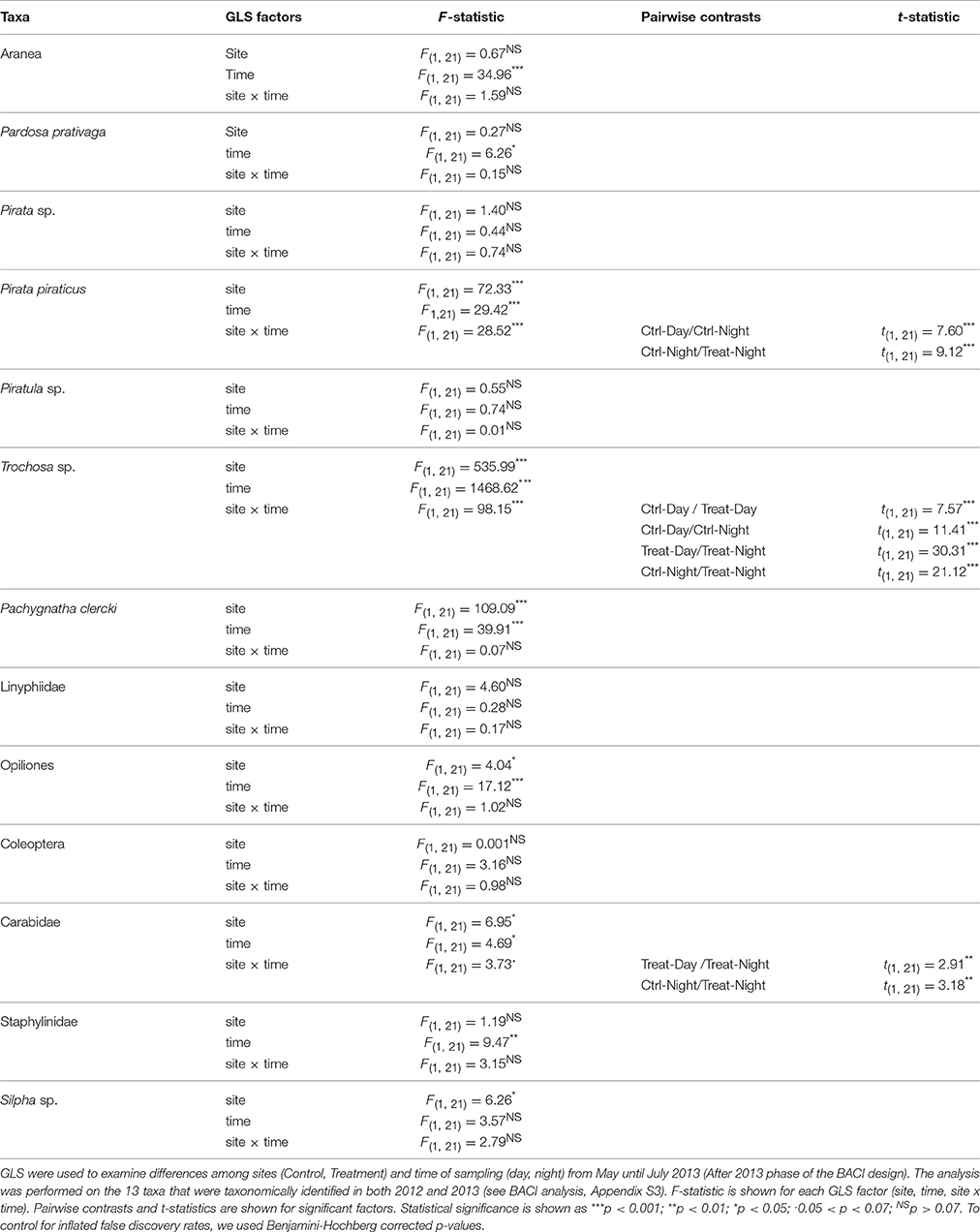
Table 3. Arthropod abundance (CPUE) was compared to assess circadian activity of ground-dwelling secondary consumers.
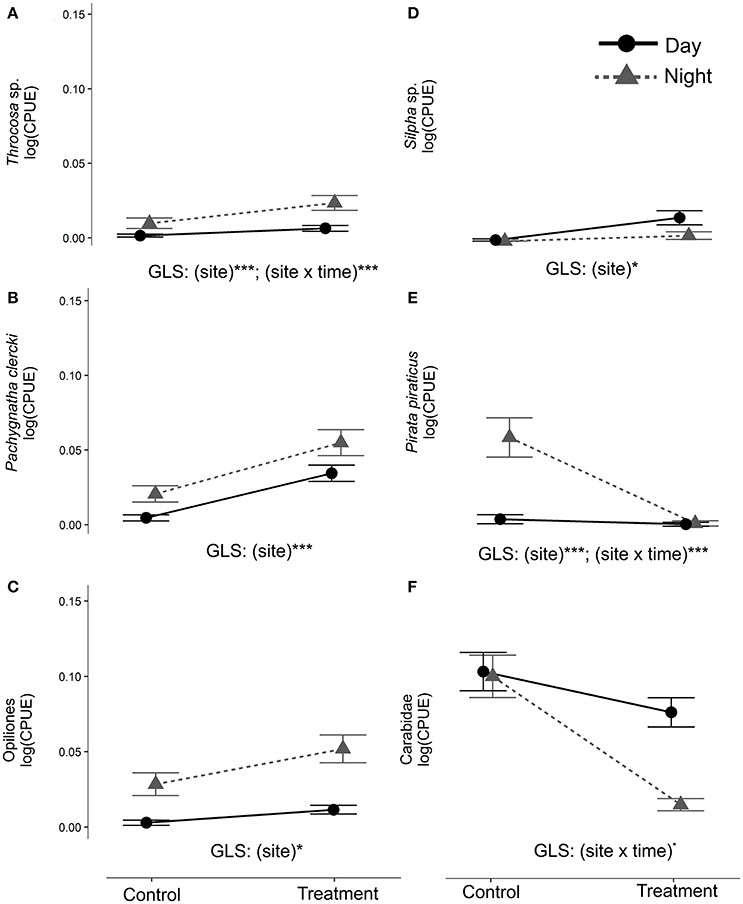
Figure 5. Arthropod abundance (CPUE) was compared to assess circadian activity of ground-dwelling secondary consumers. GLS were used to examine differences among sites (Control, Treatment), time of sampling (day, night) and their interaction for the period from May until July 2013 (“After 2013” phase). The analysis was performed on the 13 taxa that were taxonomically identified in both 2012 and 2013 (see BACI analysis). In the figure only taxa with significant GLS terms of relevance (site, site × time) are shown (panels from A–F) (***p < 0.001; *p < 0.05; ·0.05 < p < 0.07).
Discussion
This is one of the first studies to experimentally manipulate ALAN in field conditions at the ecosystem scale to test the hypothesis that ALAN can change the subsidies of aquatic insects and that this can influence the composition and abundance of local riparian consumers. Obtained results provide strong support for this hypothesis, and identify several species-specific responses.
Aquatic Insect Emergence
We found a higher flux of aquatic insects into the illuminated riparian area through an increase in emergence directly under lamps. Emergence increased by a factor 2 in the artificially lit waterbody from 2012 to 2013, with no change in the Control site. The increase was constant from May to July 2013 implying that ALAN affected overall abundances rather than emergence timing. Our results suggest that the Treatment site produced more aquatic insect biomass or that the rate of larval survival was higher. Increased illumination is generally associated with increased predation risk (Cerri and Fraser, 1983), as diurnally active piscivorous fish can extend their hunting activity at night, increasing predation pressure on smaller, invertivorous fish (Becker et al., 2013). Lee et al. (2013) attributed reduced emergence in Cloeon dipterum (the most abundant species in our emergence traps) to increased fish predation in a wetland. Abundant fish species in the ditches of the study area were the invertivorous Gasterosteus aculeatus (three-spined stickleback), Rhodeus amarus (European bitterling) and young Perca fluviatilis (European perch), as well as piscivorous visual predators such as Esox lucius (Northern pike) and adults of P. fluviatilis (A. Manfrin, pers. obs.). The invertivores have to balance predator-avoidance and feeding efficiency (Fraser and Metcalfe, 1997; Nightingale et al., 2006) and this may have reduced predation on aquatic invertebrates, leading to increased abundance and emergence (Figure 6). ALAN becomes polarised when reflected off the water surface and this can act as a cue to swarming mayflies and other insects, potentially increasing the number of eggs deposited in illuminated areas (Horváth et al., 2009). ALAN may have led to aggregation of the immature insects (still in the water) near the lights and thus the emergence traps, which were located directly under lights. We did not measure benthic densities of immature aquatic stages in the two ditches, so we cannot assess whether ALAN increased the overall density in the water. Photoperiod, water temperature and oxygen concentration are all important cues for aquatic insect emergence. Typically, warmer water causes faster growth and earlier adult emergence, thus influencing the timing of emergence (Ward and Stanford, 1982; Harper and Peckarsky, 2006). However, in our experiment we observed no differences between Control and Treatment sites in 2013 compared to 2012 in daily water temperature or in the timing of insect emergence. Oxygen concentrations can also influence insect emergence. Connolly et al. (2004) found that mayfly emergence was reduced by 60% in hypoxic (25–35% saturation) compared to normoxic (95–100% saturation) water. The mayfly C. dipterum was the most abundant taxon in our emergence traps. Compared to other mayfly species it is tolerant of low-oxygen conditions (Sartori and Landolt, 1999), therefore slight differences in oxygen concentration between Control and Treatment sites in 2013 was unlikely the cause of the increased emergence observed at the lit site during that period. Food availability (e.g., periphyton for primary consumers) is another factor affecting insect emergence. Péry et al. (2002) observed that food limitation (0.10–0.15 mg/larva/day) reduced Chironomus riparius emergence by 15% compared to individuals fed ad libitum. However, a study conducted in 2014 found no difference in periphyton biomass between Treatment and Control sites (unpublished data, M. Grubisic).
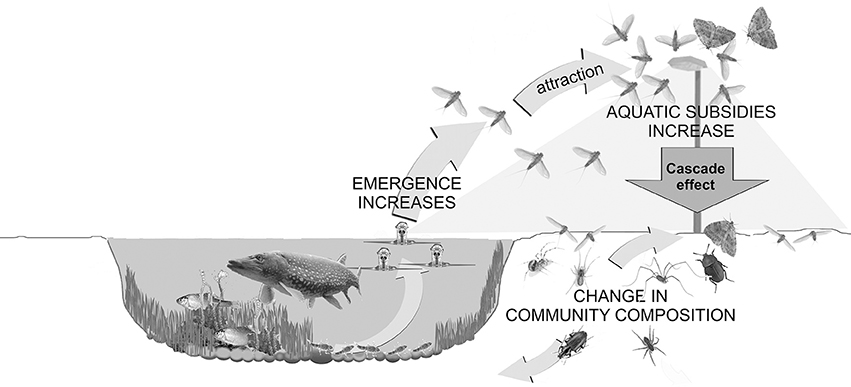
Figure 6. Conceptual figure depicting how artificial light at night (ALAN) increases the flux of aquatic insects into the riparian area through an increase in emergence under lamps and increased attraction of aquatic insects to light. The community of riparian ground-dwelling predators and scavengers is altered in the lit area and some night-active riparian spiders extend their activity into the day. Both likely are the result of the large increase in input of freshwater-derived prey.
Flying Insects
Artificial illumination attracted a large number of aquatic and terrestrial flying insects to the lit site. Light sources function as an ecological trap (van Langevelde et al., 2011; Degen et al., 2016) for many insects that are attracted to them. This occurs especially during swarming events, when very large numbers of individuals can be attracted to artificial light sources (Horváth et al., 2009). If not killed immediately, insects are often unable to disperse and migrate elsewhere (Perkin et al., 2011; Degen et al., 2016). Several studies have shown how artificial illumination disrupts dispersal patterns in arthropods, confounding natural sources of orientation (e.g., moonlight) and attracting positively phototactic insects (Horváth et al., 2011; Meyer and Sullivan, 2013). The majority of insects collected in the lit traps were of aquatic origin, suggesting that aquatic insects might be particularly sensitive and thus more vulnerable to ALAN than terrestrial insects (see also Perkin et al., 2014a). Indeed, aquatic insects perceive polarised light generated by reflections from the water surface as an important orientation cue and indicator of a suitable egg-laying habitat (Horváth et al., 2009; Perkin et al., 2014a). The presence of artificial light adjacent to water bodies may affect the distance that organisms move away from the water and therefore the spatial scale of the aquatic-terrestrial subsidy signature in a river landscape (Perkin et al., 2011; Gurnell et al., 2016). A recent review found the density of aquatic insects was reduced by 50% within the first 1.5 m from the water's edge (Muehlbauer et al., 2014). Our traps were located 3 m from the water and collected 85-fold more aquatic insects compared to unlit controls. This suggests that ALAN in a riparian ecosystem may virtually shut down most aquatic-terrestrial subsidy fluxes beyond the immediately lit area, thereby sealing off surrounding terrestrial ecosystems and contributing to isolation and loss of connectivity by interfering critical matter exchange and dispersal in a landscape.
Ground-Dwelling Arthropods
Ground-dwelling predators and scavengers were expected to be attracted by the large number of available “easy” prey, present as exhausted or dead individuals on the ground (Eisenbeis et al., 2006; Perkin et al., 2011). Contrary to expectations, we found a lower number of ground-dwelling predators and scavengers in the lit site compared to the control. It might be that these species found more food at the Treatment site, and therefore were less active and fell into the traps less. Conversely, ALAN appeared to affect the composition of ground-dwelling arthropods, with some evident taxa-specific responses. Pachygnatha clercki, Lycosidae spiders and Opiliones (harvestmen) were more abundant in lit sites, likely attracted by the increased prey availability around the lamps (Davies et al., 2012). Meyer and Sullivan (2013) reported a reduction in the abundance of Tetragnathidae spiders in illuminated locations. This diverging result is probably explained by the fact that Tetragnathidae in our study were dominated by P. clercki which has an atypical ecology for the family. While most species of this family are sit-and-wait predators that build webs, P. clercki are night-active visual hunters that do not use webs. P. clercki has a tapetum in the secondary eyes that increases visual efficiency at low light levels (Land, 1985). The increase in abundance we observed suggests it is able to efficiently use light levels provided by ALAN. We conclude that the alteration in the riparian community of ground-dwelling predators and scavengers was driven by the large increase in input of freshwater-derived prey; however, responses were taxon/specific. Most ground beetles (e.g., Agonum duftschmidi, Pterostichus nigrita, Carabus granulatus; see appendix S3 for a taxon list) had reduced abundance in the lit site. These taxa may have been directly repelled by light, or may have suffered from increased predation from, or competition with, the abundant ground spiders (Punzo et al., 2006). Similar mechanisms may explain patterns in P. piraticus and Staphylinidae. Because CPUE for these taxa were similar at the Treatment site before and after illumination, we cannot exclude factors other than ALAN in explaining the increased abundance at the Control site.
Our experimental results also suggest that artificial illumination was able to influence the circadian activity of ground-dwelling consumers (see also Davies et al., 2012). The night-active spiders P. clercki, Trochosa sp. and the night-active scavenger carrion beetle, Silpha sp., all either increased their activity into the night or extended their activity into the day in the Treatment site. These predators may have benefited from the presence of exhausted or dead insects that were attracted to the lights the night before. In contrast, Carabidae drastically decreased their activity during the night when exposed to artificial illumination.
Environmental Relevance
Here we assessed responses to ALAN in natural ecosystems previously unexposed to artificial light. This could not have been mimicked adequately under laboratory conditions. As a result, a trade-off exists between replication and realism when dealing with complex questions at the ecosystem scale, as effort and cost associated with such large-scale experiments limits the use of replicated units (Carpenter, 1990, 1996; Skelly and Kiesecker, 2001; Davies and Gray, 2015; Barley and Meeuwig, 2017). It is thus possible that the effects observed here are the result of one or more factors other than the treatment, although we tried to capture potential effects by careful monitoring of important environmental variables (see Holzhauer et al., 2015). Likewise, the experimental installation of street lights in a previously ALAN-naïve area allowed us (1) to disentangle the effects of ALAN from other aspects of urbanization such as pollution, noise, and habitat alteration that confounds most studies; and (2) to minimize the effects of potential long-term adaptations that may have already occurred in areas that have been lit for many generations. Despite the existing uncertainties, we did not observe obvious confounding treatment effects, and hence the patterns emerging from our study hint that ALAN can exert strong ecological changes in riparian systems, warranting consideration of these interactions in future artificial light installations. This becomes even more important considering the fact that this study relied on the use of high-pressure sodium lamps, which are considered to be less attractive or disruptive to insects (Eisenbeis et al., 2006). The current global shift to the use of LED lamps, with peaks in spectral white-blue (short wavelengths), is thus likely to have even greater effects on nocturnal invertebrates given their sensitivity to short wavelength light (van Langevelde et al., 2011; Pawson and Bader, 2014; van Grunsven et al., 2014).
Conclusions
Given the global abundance of artificial lights along streams and rivers and along the shores of lakes, reservoirs, and wetlands, ALAN can potentially alter cross-ecosystem fluxes at regional and global scales. Due to the important role of aquatic subsidies to consumers in recipient ecosystems, the impact of artificial illumination has to be considered as a relevant stressor in urban and landscape planning (Schroer and Hölker, 2016). We argue that it is important to include mitigation measures into new lighting concepts in order to consider ecological impacts. This requires multidisciplinary efforts by landscape- and urban planners, lighting engineers, and terrestrial and aquatic ecologists to mitigate any effects. We suggest the installation of artificial lights directly adjacent to stream riverbanks should be designed with consideration given to riparian buffers in which movement and dispersal of aquatic and terrestrial organisms are preserved.
Author Contribution
AM: study design; organized and performed field and laboratory work; data analysis; interpretation of the data; drafting and final version of the manuscript to be published; check of accuracy and integrity. GS: data analysis; interpretation of the data; drafting the work; approval of the final version; accuracy or integrity check. SL: study design; data analysis; interpretation of the data; final approval of the version to be published; accuracy or integrity check. NW: study design; organized and performed field and laboratory work; data analysis; interpretation of the data; drafting and final accuracy or integrity approval. RvG: data analysis; interpretation of the data; drafting and final version of the manuscript to be published; accuracy or integrity check, N-SW: study design; organized and performed field and laboratory work; data analysis; interpretation of the data; final approval of the version to be published. SW: study design; organized and performed field and laboratory work; data analysis; interpretation of the data; approval of the final version. MTM: study design; data analysis; interpretation of the data; drafting and approval final version; final accuracy or integrity approval. FH: study design; data analysis; interpretation of the data; drafting and approval final version; accuracy, integrity approval.
Conflict of Interest Statement
The authors declare that the research was conducted in the absence of any commercial or financial relationships that could be construed as a potential conflict of interest.
Acknowledgments
We thank Stefan Heller, Maja Grubisic, Stephanie Holzhauer, Sibylle Schroer, Martin Oehlert, Christian Schomaker, Liliana Lehmann, and Babette Pohlmann for help during the experiments. We thank Francesca Pilotto, Kirsten Pohlmann, Kate Laskowski, Ulrike Scharfenberger, Sven Teurlincx and Thomas Mehner for advice on statistical analysis. We also thank Ellard Hunting and the two referees for critical comments that improved the manuscript. This work was carried out within the Erasmus Mundus Joint Doctorate Program SMART, funded by the Education, Audiovisual and Culture Executive Agency of the European Commission. Funding was also provided by the Federal Ministry of Research and Technology, Germany (BMBF-033L038A) and the Federal Agency for Nature Conservation, Germany (FKZ 3514821700). SL was supported by an individual fellowship from the German Centre for Integrative Biodiversity Research (iDiv), Leipzig, Germany.
Supplementary Material
The Supplementary Material for this article can be found online at: https://www.frontiersin.org/articles/10.3389/fenvs.2017.00061/full#supplementary-material
Footnotes
1. ^Google Earth Pro 7.3.0.3832 (32-bit). (March 5, 2011). Lochow, Germany. 52° 41′ 29.81″ N, 12° 27′ 37.54″ E, Eye alt 1.09 Km. DigitalGlobe 2017. https://www.google.com/earth/ [September 27, 2017].
References
Barley, S. C., and Meeuwig, J. J. (2017). The power and the pitfalls of large-scale, unreplicated natural experiments. Ecosystems 20, 331–339. doi: 10.1007/s10021-016-0028-5
Bartels, P., Cucherousset, J., Steger, K., Eklöv, P., Tranvik, L. J., and Hillebrand, H. (2012). Reciprocal subsidies between freshwater and terrestrial ecosystems structure consumer resource dynamics. Ecology 93, 1173–1182. doi: 10.1890/11-1210.1
Becker, A., Whitfield, A. K., Cowley, P. D., Järnegren, J., and Næsje, T. F. (2013). Potential effects of artificial light associated with anthropogenic infrastructure on the abundance and foraging behaviour of estuary-associated fishes. J. Appl. Ecol. 50, 43–50. doi: 10.1111/1365-2664.12024
Bishop, J. E. (1969). Light control of aquatic insect activity and drift. Ecology 50, 371–380. doi: 10.2307/1933885
Carpenter, S. R. (1990). Large-Scale perturbations: opportunities for innovation. Ecology 71, 2038–2043. doi: 10.2307/1938617
Carpenter, S. R. (1996). Microcosm experiments have limited relevance for community and ecosystem ecology. Ecology 77, 677–680. doi: 10.2307/2265490
Cerri, R. D., and Fraser, D. F. (1983). Predation and risk in foraging minnows: balancing conflicting demands. Am. Nat. 121, 552–561. doi: 10.1086/284082
Connolly, N., Crossland, M., and Pearson, R. (2004). Effect of low dissolved oxygen on survival, emergence, and drift of tropical stream macroinvertebrates. J. North Am. Benthol. Soc. 23, 251–270. doi: 10.1899/0887-3593(2004)023<0251:EOLDOO>2.0.CO;2
Davies, G. M., and Gray, A. (2015). Don't let spurious accusations of pseudoreplication limit our ability to learn from natural experiments (and other messy kinds of ecological monitoring). Ecol. Evol. 5, 5295–5304. doi: 10.1002/ece3.1782
Davies, T. W., Bennie, J., and Gaston, K. J. (2012). Street lighting changes the composition of invertebrate communities. Biol. Lett. 8, 764–767. doi: 10.1098/rsbl.2012.0216
Degen, T., Mitesser, O., Perkin, E. K., Weiß, N. S., Oehlert, M., Mattig, E., et al. (2016). Street lighting: sex-independent impacts on moth movement. J. Animal Ecol. 85, 1352–1360. doi: 10.1111/1365-2656.12540
Eisenbeis, G., Rich, C., and Longcore, T. (2006). “Artificial night lighting and insects: attraction of insects to streetlamps in a rural setting in Germany” in Ecological Consequences of Artificial Night Lighting, ed G. C. Rich and T. Longcore (Washington, DC: Island Press), 191–198.
Fox, J., Weisberg, S., Adler, D., Bates, D., Baud-Bovy, G., Ellison, S., et al. (2016). Package ‘Car.’ Available online at: http://cran-r.project.org/web/packages/car/car.pdf
Fraser, N., and Metcalfe, N. (1997). The costs of becoming nocturnal: feeding efficiency in relation to light intensity in juvenile Atlantic salmon. Funct. Ecol. 11, 385–391. doi: 10.1046/j.1365-2435.1997.00098.x
Gaston, K. J., Visser, M. E., and Hölker, F. (2015). The biological impacts of artificial light at night: the research challenge. Philos. Trans. R. Soc. Lond. B 370:20140133. doi: 10.1098/rstb.2014.0133
Grubisic, M., Singer, G., Bruno, M. C., van Grunsven, R. H. A., Manfrin, A., Monaghan, M. T., et al. (2017). Artificial light at night decreases biomass and alters community composition of benthic primary producers in a sub-alpine stream. Limnol. Oceanogr. doi: 10.1002/lno.10607. [Epub ahead of print].
Gurnell, A. M., Bertoldi, W., Tockner, K., Wharton, G., and Zolezzi, G. (2016). How large is a river? Conceptualizing river landscape signatures and envelopes in four dimensions. Wiley Interdiscip. Rev. 3, 313–325. doi: 10.1002/wat2.1143
Harper, M. P., and Peckarsky, B. L. (2006). Emergence cues of a mayfly in a high-altitude stream ecosystem: potential response to climate change. Ecol. Appl. 16, 612–621. doi: 10.1890/1051-0761(2006)016[0612:ECOAMI]2.0.CO;2
Hölker, F., Wolter, C., Perkin, E. K., and Tockner, K. (2010). Light pollution as a biodiversity threat. Trends Ecol. Evol. 25, 681–682. doi: 10.1016/j.tree.2010.09.007
Hölker, F., Wurzbacher, C., Weißenborn, C., Monaghan, M. T., Holzhauer, S. I., and Premke, K. (2015). Microbial diversity and community respiration in freshwater sediments influenced by artificial light at night. Philos. Trans. R. Soc. Lond. B 370:20140130. doi: 10.1098/rstb.2014.0130
Holzhauer, S. I., Franke, S., Kyba, C., Manfrin, A., Klenke, R., Voigt, C. C., et al. (2015). Out of the dark: establishing a large-scale field experiment to assess the effects of artificial light at night on species and food webs. Sustainability 7, 15593–15616. doi: 10.3390/su71115593
Honnen, A.-C., Johnston, P. R., and Monaghan, M. T. (2016). Sex-specific gene expression in the mosquito Culex pipiens f. molestus in response to artificial light at night. BMC Genomics 17, 1–22. doi: 10.1186/s12864-015-2336-0
Horváth, G., Kriska, G., Malik, P., and Robertson, B. (2009). Polarized light pollution: a new kind of ecological photopollution. Front. Ecol. Environ. 7:129. doi: 10.1890/080129
Horváth, G., Móra, A., Bernáth, B., and Kriska, G. (2011). Polarotaxis in non-biting midges: female chironomids are attracted to horizontally polarized light. Physiol. Behav. 104, 1010–1015. doi: 10.1016/j.physbeh.2011.06.022
International Dark Sky Association (IDA) (2015). International Dark Sky Reserves: Westhavelland (Germany). Available online at: http://darksky.org/idsp/reserves/westhavelland/ (Accessed on November 13, 2015).
Knop, E., Zoller, L., Ryser, R., Gerpe, C., Hörler, M., and Fontaine, C. (2017). Artificial light at night as a new threat to pollination. Nature 548, 206–209. doi: 10.1038/nature23288
Kummu, M., De Moel, H., Ward, P. J., and Varis, O. (2011). How close do we live to water? A global analysis of population distance to freshwater bodies. PLoS ONE 6:e20578. doi: 10.1371/journal.pone.0020578
Kurvers, R. H., and Hölker, F. (2015). Bright nights and social interactions: a neglected issue. Behav. Ecol. 26, 334–339. doi: 10.1093/beheco/aru223
Land, M. (1985). “The morphology and optics of spider eyes,” in Neurobiology of Arachnids, ed F. G. Barth (Berlin; Heidelberg: Springer), 53–78.
Lee, C. Y., Kim, D. G., Baek, M. J., Choe, L. J., and Bae, Y. J. (2013). Life history and emergence pattern of Cloeon dipterum (Ephemeroptera: Baetidae) in Korea. Environ. Entomol. 42, 1149–1156. doi: 10.1603/EN13012
Lenth, R. V. (2016). Least-squares means: the R Package lsmeans. J. Stat. Softw. 69, 1–33. doi: 10.18637/jss.v069.i01
Longcore, T., and Rich, C. (2004). Ecological light pollution. Front. Ecol. Environ. 2, 191–198. doi: 10.1890/1540-9295(2004)002[0191:ELP]2.0.CO;2
Marczak, L. B., and Richardson, J. S. (2007). Spiders and subsidies: results from the riparian zone of a coastal temperate rainforest. J. Anim. Ecol. 76, 687–694. doi: 10.1111/j.1365-2656.2007.01240.x
Mehner, T., Ihlau, J., Dörner, H., and Hölker, F. (2005). Can feeding of fish on terrestrial insects subsidize the nutrient pool of lakes? Limnol. Oceanogr. 50, 2022–2031. doi: 10.4319/lo.2005.50.6.2022
Meyer, L. A., and Sullivan, S. M. P. (2013). Bright lights, big city: influences of ecological light pollution on reciprocal stream–riparian invertebrate fluxes. Ecol. Appl. 23, 1322–1330. doi: 10.1890/12-2007.1
Moore, M. V., Pierce, S. M., Walsh, H. M., Kvalvik, S. K., and Lim, J. D. (2001). Urban light pollution alters the diel vertical migration of Daphnia. Int. Vereinigung fur Theor. Angewandte Limnol. Verhandlungen 27, 779–782.
Muehlbauer, J. D., Collins, S. F., Doyle, M. W., and Tockner, K. (2014). How wide is a stream? Spatial extent of the potential “stream signature” in terrestrial food webs using meta-analysis. Ecology 95, 44–55. doi: 10.1890/12-1628.1
Nakano, S., and Murakami, M. (2001). Reciprocal subsidies: dynamic interdependence between terrestrial and aquatic food webs. Proc. Natl. Acad. Sci. U.S.A. 98, 166–170. doi: 10.1073/pnas.98.1.166
Navara, K. J., and Nelson, R. J. (2007). The dark side of light at night: physiological, epidemiological, and ecological consequences. J. Pineal Res. 43, 215–224. doi: 10.1111/j.1600-079X.2007.00473.x
Nightingale, B., Longcore, T., and Simenstad, C. A. (2006). “Artificial night lighting and fishes,” in Ecological Consequences of Artificial Night Lighting, eds G. C. Rich and T. Longcore (Washington, DC: Island Press), 257–276.
Pawson, S., and Bader, M.-F. (2014). LED lighting increases the ecological impact of light pollution irrespective of color temperature. Ecol. Appl. 24, 1561–1568. doi: 10.1890/14-0468.1
Perkin, E. K., Hölker, F., Richardson, J. S., Sadler, J. P., Wolter, C., and Tockner, K. (2011). The influence of artificial light on stream and riparian ecosystems: questions, challenges, and perspectives. Ecosphere 2, 1–16. doi: 10.1890/ES11-00241.1
Perkin, E. K., Hölker, F., and Tockner, K. (2014a). The effects of artificial lighting on adult aquatic and terrestrial insects. Freshw. Biol. 59, 368–377. doi: 10.1111/fwb.12270
Perkin, E. K., Hölker, F., Tockner, K., and Richardson, J. S. (2014b). Artificial light as a disturbance to light-naïve streams. Freshw. Biol. 59, 2235–2244. doi: 10.1111/fwb.12426
Péry, A. R., Mons, R., Flammarion, P., Lagadic, L., and Garric, J. (2002). A modeling approach to link food availability, growth, emergence, and reproduction for the midge Chironomus riparius. Environ. Toxicol. Chem. 21, 2507–2513. doi: 10.1002/etc.5620211133
Pinheiro, J., Bates, D., DebRoy, S., and Sarkar, D. (2015). R Development Core Team. (2014). nlme: Linear and Nonlinear Mixed Effects Models. R Package Version 3.1–117.
Polis, G. A., Anderson, W. B., and Holt, R. D. (1997). Toward an integration of landscape and food web ecology: the dynamics of spatially subsidized food webs. Annu. Rev. Ecol. Syst. 28, 289–316. doi: 10.1146/annurev.ecolsys.28.1.289
Punzo, F., Farmer, C., and Cook, J. (2006). Life history and ecology of the wolf spider Pardosa sierra Banks (Araneae: Lycosidae) in southeastern Arizona. Southwest. Nat. 51, 310–319. doi: 10.1894/0038-4909(2006)51[310:L.H.A.E.O.T.]2.0.C.O.;2
R Core Team (2015). R: A Language and Environment for Statistical Computing. Vienna: R Foundation for Statistical Computing; 2013. Available online at: http://www.r-project.org
Richardson, J. S., Zhang, Y., and Marczak, L. B. (2010). Resource subsidies across the land–freshwater interface and responses in recipient communities. River Res. Appl. 26, 55–66. doi: 10.1002/rra.1283
Roberts, M. J. (1996). Collins Field Guide to the Spiders of Britain and Northern Europe – Harper. London: Collins.
Sartori, M., and Landolt, P. (1999). Atlas de Distribution des Éphémères de Suisse (Insecta, Ephemeroptera) Vol. 3 (Neuchâtel, CH: Centre Suisse de Cartographie de la Faune).
Schroer, S., and Hölker, F. (2016). “Impact of lighting on flora and fauna,” in Handbook of Advanced Lighting Technology Reference, ed R. Karlicek (Cham: Springer), 1–33.
Shapiro, S. S., and Wilk, M. B. (1965). An analysis of variance test for normality (complete samples). Biometrika 52, 591–611. doi: 10.1093/biomet/52.3-4.591
Skelly, D. K., and Kiesecker, J. M. (2001). Venue and outcome in ecological experiments: manipulations of larval anurans. Oikos 94, 198–208. doi: 10.1034/j.1600-0706.2001.t01-1-11105.x
Spoelstra, K., van Grunsven, R. H., Donners, M., Gienapp, P., Huigens, M. E., Slaterus, R., et al. (2015). Experimental illumination of natural habitat—an experimental set-up to assess the direct and indirect ecological consequences of artificial light of different spectral composition. Philos. Trans. R. Soc. Lond. B 370:20140129. doi: 10.1098/rstb.2014.0129
Stewart-Oaten, A., Bence, J. R., and Osenberg, C. W. (1992). Assessing effects of unreplicated perturbations: no simple solution. Ecology 73, 1396–1404. doi: 10.2307/1940685
Stewart-Oaten, A., Murdoch, W. W., and Parker, K. R. (1986). Environmental impact assessment:“Pseudoreplication” in time? Ecology 67, 929–940. doi: 10.2307/1939815
Stresemann, E. (2011). Exkursions fauna von Deutschland – Band 2 Wirbellose Insekten, 11, Auflag. Heidelberg: Spektrum Akademischer Verlag.
Tabor, R. A., Brown, G. S., and Luiting, V. T. (2004). The effect of light intensity on sockeye salmon fry migratory behavior and predation by cottids in the Cedar River, Washington. North Am. J. Fish. Manage. 24, 128–145. doi: 10.1577/M02-095
Takimoto, G., Iwata, T., and Murakami, M. (2002). Seasonal subsidy stabilizes food web dynamics: balance in a heterogeneous landscape. Ecol. Res. 17, 433–439. doi: 10.1046/j.1440-1703.2002.00502.x
van Grunsven, R. H., Donners, M., Boekee, K., Tichelaar, I., Van Geffen, K., Groenendijk, D., et al. (2014). Spectral composition of light sources and insect phototaxis, with an evaluation of existing spectral response models. J. Insect Conserv. 18, 225–231. doi: 10.1007/s10841-014-9633-9
van Langevelde, F., Ettema, J. A., Donners, M., WallisDeVries, M. F., and Groenendijk, D. (2011). Effect of spectral composition of artificial light on the attraction of moths. Biol. Conserv. 144, 2274–2281. doi: 10.1016/j.biocon.2011.06.004
Waite, T. A., and Campbell, L. G. (2006). Controlling the false discovery rate and increasing statistical power in ecological studies. Ecoscience 13, 439–442. doi: 10.2980/1195-6860(2006)13[439:CTFDRA]2.0.CO;2
Wallace, J. B., Eggert, S., Meyer, J. L., and Webster, J. (1999). Effects of resource limitation on a detrital-based ecosystem. Ecol. Monogr. 69, 409–442. doi: 10.1890/0012-9615(1999)069[0409:EORLOA]2.0.CO;2
Ward, J. V., and Stanford, J. (1982). Thermal responses in the evolutionary ecology of aquatic insects. Annu. Rev. Entomol. 27, 97–117. doi: 10.1146/annurev.en.27.010182.000525
Keywords: light pollution, predator-prey, cross-ecosystem, ALAN, insects, aquatic subsidies
Citation: Manfrin A, Singer G, Larsen S, Weiß N, van Grunsven RHA, Weiß N-S, Wohlfahrt S, Monaghan MT and Hölker F (2017) Artificial Light at Night Affects Organism Flux across Ecosystem Boundaries and Drives Community Structure in the Recipient Ecosystem. Front. Environ. Sci. 5:61. doi: 10.3389/fenvs.2017.00061
Received: 31 August 2017; Accepted: 21 September 2017;
Published: 20 October 2017.
Edited by:
Ellard Roy Hunting, Leiden University, NetherlandsReviewed by:
Roel Van Klink, University of Bern, SwitzerlandRaul A. Loayza-Muro, Cayetano Heredia Peruvian University, Peru
Copyright © 2017 Manfrin, Singer, Larsen, Weiß, van Grunsven, Weiß, Wohlfahrt, Monaghan and Hölker. This is an open-access article distributed under the terms of the Creative Commons Attribution License (CC BY). The use, distribution or reproduction in other forums is permitted, provided the original author(s) or licensor are credited and that the original publication in this journal is cited, in accordance with accepted academic practice. No use, distribution or reproduction is permitted which does not comply with these terms.
*Correspondence: Alessandro Manfrin, YWxlc3NhbmRyby5tYW5mcmluQGhvdG1haWwuY29t
†Present Address: Alessandro Manfrin, Umwelt Campus Birkenfeld, Fachhochschule Trier, Birkenfeld, Germany
‡These authors have contributed equally to this work.