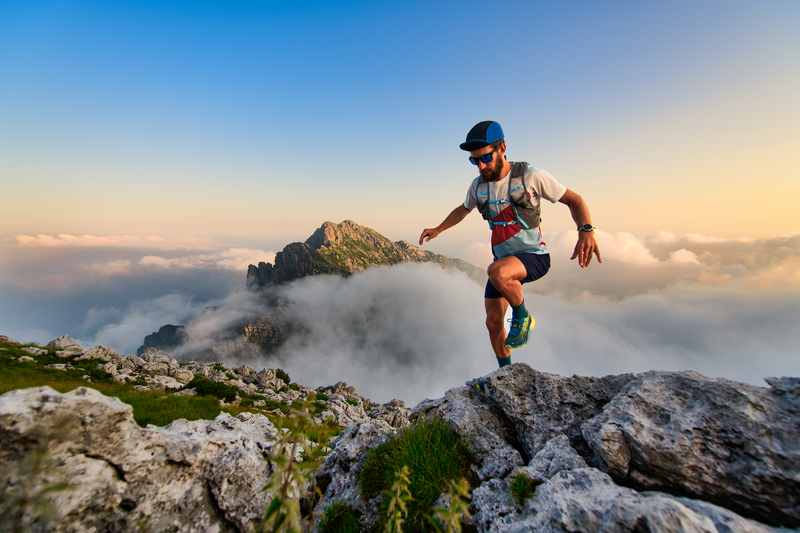
94% of researchers rate our articles as excellent or good
Learn more about the work of our research integrity team to safeguard the quality of each article we publish.
Find out more
ORIGINAL RESEARCH article
Front. Environ. Sci. , 15 October 2015
Sec. Interdisciplinary Climate Studies
Volume 3 - 2015 | https://doi.org/10.3389/fenvs.2015.00069
Because the current technologies for capturing CO2 are still too energy intensive, new materials must be developed that can capture CO2 reversibly with acceptable energy costs. At a given CO2 pressure, the turnover temperature (Tt) of the reaction of an individual solid that can capture CO2 is fixed. Such Tt may be outside the operating temperature range (ΔTo) for a practical capture technology. To adjust Tt to fit the practical ΔTo, in this study, three scenarios of mixing schemes are explored by combining thermodynamic database mining with first principles density functional theory (DFT) and phonon lattice dynamics calculations. Our calculated results demonstrate that by mixing different types of solids, it's possible to shift Tt to the range of practical operating temperature conditions. According to the requirements imposed by the pre- and post- combustion technologies and based on our calculated thermodynamic properties for the CO2 capture reactions by the mixed solids of interest, we were able to identify the mixing ratios of two or more solids to form new sorbent materials for which lower capture energy costs are expected at the desired pressure and temperature conditions.
Today, fossil fuels are still the main energy sources for the world's economy. One consequence of the use of these fuels is the emission of huge quantities of CO2 into the atmosphere, creating environmental problems such as climate change (White et al., 2003; Aaron and Tsouris, 2005; Allen et al., 2009; Haszeldine, 2009; Li et al., 2013). To mitigate such problems, CO2 emissions into the atmosphere must be reduced by being captured and stored (Ochoa-Fernandez et al., 2005; Pfeiffer and Bosch, 2005; Li et al., 2013). Current technologies for capturing CO2, including solvent-based (amines) and CaO-based materials, are still too energy intensive. Hence, development of new materials that can capture and release CO2 reversibly with acceptable energy costs are critical. In particular, solid oxide sorbent materials have been proposed for capturing CO2 through a reversible chemical transformation leading primarily to formation of carbonate products. Solid sorbents containing alkali and alkaline earth metals have been reported in previous studies to be promising candidates for CO2 sorbent applications due to their high CO2 absorption capacity at moderate working temperatures (Duan and Sorescu, 2009, 2010; Duan et al., 2012a).
During the past few years, NETL developed a theoretical methodology to identify promising solid sorbent candidates for CO2 capture by combining thermodynamic database searching with ab initio thermodynamics obtained based on the first-principles density functional theory (DFT) and lattice phonon dynamics (Duan and Sorescu, 2009, 2010; Duan and Parlinski, 2011; Duan et al., 2011, 2012a,b,c; Zhang et al., 2012). As shown in Figure 1, the primary outcome of our screening scheme is a list of promising CO2 sorbents with optimal energy usage. After screening a given material databank, we selected only a short list of candidates for further experimental validations.
A practical CO2 capture technology has optimal operating conditions, such as the absorption/desorption of CO2 at the necessary pressure and operating temperature range (ΔTo). As a good sorbent, its CO2 capture/release temperature should fit into such a range. However, at a given CO2 pressure, the turnover temperature (Tt) of an individual solid capture CO2 reaction is fixed. Such Tt may be outside the operating temperature range (ΔTo) for a particular capture technology. In order to adjust Tt to fit the practical ΔTo, its corresponding thermodynamic property must be changed by changing its structure by reacting (mixing) it with other materials or doping it with other elements. In this study, we demonstrate that by mixing different types of solids in three scenarios, it's possible to shift Tt to the range of practical operating conditions.
A complete description of the computational methodology together with relevant applications can be found in our previous publications (Duan and Sorescu, 2009, 2010; Duan, 2011; Duan and Parlinski, 2011; Duan et al., 2011, 2012a,b,c; Zhang et al., 2012). The CO2 capture reactions of solids can be expressed generically in the form (for convenient description, we normalized the reaction to 1 mole of CO2)
where nRi, nPj are the numbers of moles of reactants (Ri) and products (Pj) involved in the capture reactions. As discussed in the following section, the reactants Ri can simply be mixed solids or a newly formed solid by mixing different kinds of solids with certain mixing ratios. We treat the gas phase CO2 as an ideal gas. By assuming that the difference between the chemical potentials (Δμ0) of the solid phases of reactants (Ri) and products (Pj) can be approximated by the difference in their total energies (ΔEDFT), obtained directly from DFT calculations, and their vibrational free energies of phonons dynamics and by ignoring the PV contribution terms for solids, the variation of the Gibbs free energy (ΔG) for reaction (a) with temperature and CO2 pressure can be written as (Duan and Sorescu, 2009, 2010; Duan, 2011; Duan and Parlinski, 2011; Duan et al., 2011, 2012a,b,c; Zhang et al., 2012)
where
Here, ΔEDFT is the DFT energy difference between the reactants and products of reaction (a), ΔEZP is the zero point energy difference between the reactants and products and can be obtained directly from phonon calculations. ΔFPH is the phonon free energy change excluding zero-point energy (which is already counted into the ΔEZP term) between the solids of products and reactants. PCO2 is the partial pressure of CO2 in the gas phase and P0 is the standard state reference pressure taken to be 1 bar. The heat of reaction [ΔHcal(T)] can be evaluated through the following equation
where ΔSPH(T) is the difference of entropies between product solids and reactant solids. The free energy of CO2 () can be obtained from standard statistical mechanics (Duan and Sorescu, 2009, 2010; Zhang et al., 2012), and its entropy (SCO2) can be found in the empirical thermodynamic databases (Chase, 1998). Equation (1) provides the relationships of Gibbs free energy change of reaction (a) vs. temperature and CO2 pressure. Obviously, when set ΔG = 0, the P-T relationship (van't Hoff plot) is obtained to determine the Tt:
Based on this equation, at giving CO2 pressure PCO2, the Tt can be determined for each CO2 capture reaction.
For a given CO2 capture process, its optimal working conditions [CO2 pressures of pre- and after-capture, absorption/desorption temperature range (ΔTo), etc.] were fixed. However, at a given CO2 pressure, the Tt of an individual solid capture CO2 reaction is also fixed. Such Tt may be outside the operating temperature range ΔTo for a particular capture technology. To adjust Tt to fit the practical working range through reversible chemical transformations, the chemical properties (such as structure, phase, etc.) of solids must be modified to change the ΔG(T, P) in Equation (1). If we want to increase the Tt to a higher temperature range, the ΔG(T, P) should be more negative. To achieve it, we can either destabilize the reactants (sorbents), stabilize the products, or do both. Conversely, if we want to decrease Tt to a lower temperature range, the ΔG(T, P) should be less negative, which can either stabilize the reactant (sorbents), destabilize the products, or do both. In other words, mixing stabilizer/destabilizer with a solid could change the thermodynamic properties of their CO2 capture reactions to shift Tt. Some mixing examples are given in Table 1. As one can see that the mixed sorbent could be a new formed solid (e.g., lithium silicates) or just a simple mixture (e.g., MgO + Na2CO3) to change the chemical properties of reactants and products. Depending on the main captor A and the direction of Tt shifts, a different mixed solid B and mixing ratio could be determined. Although one can mix any number of solids to form a new CO2 sorbent, to focus on exploring the nature of mixtures, here we restrict ourselves with cases of two and three mixed solids.
As shown in Table 1, as effective main CO2 captors through chemical reactions to form carbonates, alkali and alkaline earth metal oxides are of interest due to their ease in reacting with CO2 and low costs. The problem is that they can strongly react with CO2 to form carbonates, but their Tt are very high, and they can only be regenerated at very high temperatures, which are unsuitable for many CO2 capture technologies. Hence, mixing with other solids to shift their Tt becomes important for their suitability as CO2 sorbents. Generally, when we mix two solids A and B to form a new sorbent C, the Tt of the new system (TC) is located between the A and B (TA, TB). Here, it was assumed that A is a strong CO2 sorbent, while B is a weak CO2 sorbent and TA > TB. Also, we assumed that the desired operating temperature TO is between TA and TB (TA > TO > TB). Depending on the properties of A and B, as shown in Table 1, we typically have three scenarios to synthesize the mixing sorbent C.
In this case, by mixing B into A, a new solid C is formed. Because B is not involved in the CO2 capture, it serves as a stabilizer to stabilize A to form solid C, and the reaction possesses lower energy than A and B individually. Therefore, the energy state of reactant is lower than pure A. The Δμ0 in Equation (2) will become higher (less negative). According to Equation (4), the corresponding TC should be lower than TA. In other words, the mixed sorbent B shifts TA to a lower temperature range. The amount of shifted temperature depends on the mixing ratio.
An example of this case is represented by Li2O. As we know, Li2O is a very strong CO2 sorbent that forms Li2CO3. However, its regeneration from Li2CO3 can only occur at very high temperatures (TA > 1000 K). In order to move its TA to lower temperatures, one can mix in some weak CO2 sorbents (such as SiO2, ZrO2). With different mixing ratios of Li2O/SiO2 (or ZrO2), different stable lithium silicates (or zirconates) can be formed, such as Li8SiO6, Li4SiO4, Li6Si2O7, Li2SiO3, Li2Si2O5, Li2Si3O7; Li8ZrO6, Li6Zr2O7, Li2ZrO3, etc. The crystal structures of these lithium silicates and zirconates can be found in the literature. By performing ab initio thermodynamic property calculations on these solids capturing CO2 reactions (Duan and Sorescu, 2009; Duan, 2011, 2012, 2013; Duan and Parlinski, 2011; Duan et al., 2013), Figure 2 shows the calculated relationships of Gibbs free energy (ΔG), PCO2, and T of the CO2 capture reactions by the mixed Li2O/SiO2 and Li2O/ZrO2 solids with different mixing ratios. Figure 3 shows the turnover temperatures and the CO2 capture capacities of Li2O/SiO2 and Li2O/ZrO2 mixtures vs. the ratio of Li2O/SiO2 or Li2O/ZrO2 (Duan and Sorescu, 2009, 2010; Duan, 2011, 2012, 2013; Duan and Parlinski, 2011; Duan et al., 2012a; Duan and Lekse, 2015).
Figure 2. Plots of the calculated free energy vs. CO2 pressures and temperatures for the CO2 capture reaction by Li2O+SiO2 or Li2O+ZrO2 mixtures. Y-axis plotted in logarithm scale. The line shows ΔG(T, P) = 0. For each reaction, above its ΔG(T, P) = 0 curve, its ΔG < 0, which means the solids absorb CO2 and the reaction goes forward, whereas below the ΔG(T, P) = 0 curve, its ΔG > 0, which means the CO2 starts to release and the reaction goes backward to regenerate the sorbents.
Figure 3. The calculated turnover temperatures and CO2 capture capacity vs. molar percentage of SiO2 or ZrO2 in the lithium silicates or zirconates. T1 are the turnover temperatures under pre-combustion conditions with CO2 partial pressure at 20 bars, while T2 are the turnover temperatures under post-combustion conditions with CO2 partial pressure at 0.1 bar.
From Figures 2, 3, one can see that after mixing SiO2 (or ZrO2) in Li2O with different Li2O/SiO2 (or Li2O/ZrO2) ratios, the TC of the newly formed C compound (silicate or zirconate) is lower than TA of pure Li2O and could be close to the ΔTo range to fit the practical needs. Although SiO2 and ZrO2 do not capture CO2, they can exothermically react with Li2O to form silicates and zirconates (as shown in Figure 2). Such reactions bring the energy levels of sorbents (reactants) down to more negative values, but do not affect the products (carbonates). Therefore, the heat of reaction and free energy change of the CO2 capture reactions by these lithium silicates and zirconates will be increased to less negative values compared to pure Li2O reacting with CO2, and in turn, the turnover temperatures are shifted to a lower temperature range. When the SiO2/Li2O or ZrO2/Li2O ratios are increased by adding more SiO2 or ZrO2 into Li2O, as shown in Figures 2, 3, the turnover temperatures of mixed sorbents (Tt) are shifted to a lower temperature range. Therefore, by controlling the mixing ratio, it is possible to move the CO2 capture temperature down to the range required by certain capture technology.
Opposite to the previous case, in this case, we want to increase the Tt. Because TB is lower than TO, mixing A into B will increase the turnover temperature TC of the C solid to a value closer to To. In this way, A will either destabilize solid B or stabilize the captured products. For example, pure MgO has a very high theoretical CO2 capture capacity. However, its Tt (250°C) is lower than the required temperature range of 300–470°C used in warm gas clean up technology and its practical CO2 capacity is very low. Therefore, pure MgO cannot be used directly as a CO2 sorbent in such capture technology (Zhang et al., 2013, 2014; Chi et al., 2014). Figure 4 shows an example of mixing Na2O (or Na2CO3) with MgO to move the ΔG(T, P) = 0 curve of MgO (red line in Figure 4) to a higher temperature range (green line in Figure 4).
Figure 4. Plots of the calculated free energy vs. CO2 pressures and temperatures for the CO2 capture reaction by MgO + Na2CO3 (or Na2O) to form the double salt Na2Mg(CO3)2. Y-axis plotted in logarithm scale. The line shows ΔG(T, P) = 0. For each reaction, above its ΔG(T, P) = 0 curve, its ΔG < 0, which means the solids absorb CO2 and the reaction goes forward, whereas below the ΔG(T, P) = 0 curve, its ΔG > 0, which means the CO2 starts to release and the reaction goes backward to regenerate the sorbents.
One can see that in Figure 4, Na2O (or Na2CO3) does not react with the reactant MgO directly, but it reacts exothermically with the carbonate MgCO3 to form the double salt Na2Mg(CO3)2 and, hence, stabilizes the product. Therefore, the heat of reaction and free energy change of CO2 capture reaction by MgO + Na2CO3 are lower (more negative) compared with pure MgO reacting with CO2. According to Equations (1) and (4), the Tt of the Na2CO3-promoted MgO sorbent capturing CO2 is higher than that of pure MgO. Figure 5 shows the changes of MgO turnover temperatures by adding different oxides or carbonates. Generally, by mixing alkali metal oxides, M2O (M = Na, K, Cs, Ca) or their carbonates (M2CO3) into MgO, the corresponding newly formed mixing systems have higher turnover temperatures, making them useful as CO2 sorbents through the reaction MgO + CO2 + M2CO3 = M2Mg(CO3)2 (Zhang et al., 2013; Duan et al., 2014).
Figure 5. The shifts of MgO turnover temperatures by adding several oxides (carbonates) with a molar ratio of 1:1. T1 are the turnover temperatures under pre-combustion conditions with CO2 partial pressure of 20 bars, while T2 are the turnover temperatures under post-combustion conditions with CO2 partial pressure of 0.1 bar.
From Figure 5, one can see that, in these CO2 capture reactions, the Na2CO3-promoted MgO possesses a larger Tt shift to a higher temperature range than the K2CO3 + MgO and CaCO3 + MgO do. Because the desired Tt of the mixture is lower than the dissociation temperature of promotors (Na2CO3, K2CO3, CaCO3), during the CO2 capture sorption process, these promoters act as stabilizers to react with MgCO3 to form a double salt, while during the CO2 desorption, the double salt dissociates to MgO and the promoter.
In this case, we want both A and B components active to capture CO2, and the CO2 capture capacity of the mixture is the summation of those of A and B. As we know, another potential advantage of mixing solids is to increase the surface area of the solids to have a faster reaction rate. Such a mixing scenario does not show too much advantage in shifting the capture temperature, but it may enhance the kinetics of the capture process and eventually make the mixtures more efficient for capturing CO2. Although, up to now, no such report has appeared in literature, we think such an attempt is worthwhile, and we are working on several doped systems. Here we show a case of Li-/K- doped Na2ZrO3 capture for CO2 through the following reaction (b): (Duan, 2014; Duan and Lekse, 2015; Duan et al., 2015)
Figure 6 demonstrated the calculated relationships among the Gibbs free energy change, CO2 pressure and temperature for CO2 capture reactions by Na2−xMxZrO3 (M = Li, K, x = 0.0, 0.5, 1.0, 1.5, 2.0). Figure 7 showed the dependence of Tt and CO2 capture capacity on Li/K doping molar percentage in NaxM2−xZrO3 (M = Li, K). One can see from Figure 6 that when Na2ZrO3 is doped with a different molar ratio of Li or K, the thermodynamic properties of the doped systems are quite different from pure Na2ZrO3.
Figure 6. The contour plotting of calculated Gibbs free energy vs. CO2 pressures and temperatures of the CO2 capture reactions by NaxM2-xZrO3 and M2ZrO3(M = Li, Na, K, x = 0, 0.5, 1.0, 1.5, 2.0) through reaction NaxM2−xZrO3 + CO2 = x/2Na2CO3 + 2−x/2M2CO3 + ZrO2. Y-axis plotted in logarithm scale. The line shows ΔG(T, P) = 0. For each reaction, above its ΔG(T, P) = 0 curve, its ΔG < 0, which means the solids absorb CO2 and the reaction goes forward, whereas below the ΔG(T, P) = 0 curve, its ΔG > 0, which means the CO2 starts to release and the reaction goes backward to regenerate the sorbents.
Figure 7. The dependence of the turnover temperatures and CO2 capture capacity on Li/K doping molar percentage in NaxM2−xZrO3 (M = Li, K). T1 is the turnover temperatures under pre-combustion conditions with CO2 partial pressure at 20 bars, while T2 is the turnover temperatures under post-combustion conditions with CO2 partial pressure at 0.1 bar.
Based on the calculated relationships among the Gibbs free energy change, CO2 pressure and temperature for CO2 capture reactions by Na2−xMxZrO3 are shown in Figure 6. Compared to pure Na2ZrO3, overall, the Li- and K-doped mixtures Na2−xMxZrO3 have lower Tt, shown in Figure 7. The calculated results show that the shift in Tt depends not only on the doping element, but also on the doping level. As one can see from Figure 7, the Li-doped systems have larger Tt decreases than the K-doped systems. When increasing the Li-doping level x, the Tt of the corresponding mixture Na2−xLixZrO3 decreases further to a low temperature range. However, in the case of K-doped systems Na2−xKxZrO3—although initial doping of K into Na2ZrO3 can shift its Tt to a lower temperature range—further increasing the K-doping level x results in an increase in Tt. Therefore, compared to K-doping, lithium inclusion into Na2ZrO3 structure has a larger influence on its CO2 capture performance.
All of these obtained results may be of great interest in the development of specific CO2 capture applications. As shown in Figures 6, 7, the Na2−xLixZrO3 and Na2−xKxZrO3 compositions can produce modifications in the CO2 capture temperatures, which may be used in the design of a specific composition, depending on the temperature range that industry requires. These results have identified that the capture of CO2 in zirconate materials is not simply a matter of a substitutional element but also the doping level. This insight will need to be considered during future sorbent development. The obtained results have also demonstrated that computational methods can be used to accurately predict aspects of CO2 capture and have the potential to drive future work by leading to researchers identifying and designing the most promising candidate materials.
In addition, the Na2−xMxZrO3 materials can be stoichiometrically regarded as a mixture of three oxides: Na2O, M2O, and ZrO2 in the ratio (2-x):x:1. These results provide ways that mixing/doping more than two oxides to form new sorbents that can fit the industrial needs to capture CO2 with better performances and proper working conditions. More complicated doping systems, such as N2−xMxMeSiO4 (N, M = Li, Na, K; Me = Fe, Co, Ni, Mn, Zn, Mg, etc.), can be regarded as a mixture of four oxides: N2O, M2O, MeO, SiO2 in the ratio (2-x):x:1:1. Their CO2 capture reactions could be written as:
By analyzing their CO2 capture behaviors with different doping/mixing ratios, researchers will draw more general conclusions. Analysis of the results is underway and will appear in future reports.
From the mixing systems mentioned, one can see that after mixing/doping solid B into A, the theoretical maximum of CO2 capture capacity of the mixture is decreased compared with pure A or B. However, it does not mean the practical CO2 capacity will be decreased. The CO2 capacity of solid sorbents should be above 3 mole CO2 per kilogram solid (~15 wt. %) to meet the industrial requirements and have a chance of providing energy reductions of 30–50% or more compared to the optimum aqueous-monoethanolamine(MEA)-based process (Gray et al., 2008). As shown in Figures 3, 5, 7, the theoretical CO2 weight percentage maxima of all these mixtures are greater than this minimum requirement (>15 wt. %). Therefore, from the CO2 capture capacity point of view, all of these systems could meet this criterion to be used as CO2 sorbents. In addition, after mixing another solid, the structure of the sorbent is changed, and more active sites could be contacted by CO2 to enhance the capture kinetics, and in turn, to increase its practical capture capacity.
At a given CO2 pressure, the Tt of an individual solid capture CO2 reaction is fixed. Such Tt may be outside the operating temperature range (ΔTo) for a practical capture technology. To adjust Tt to fit the practical ΔTo, in this study, by combining thermodynamic database mining with first principles DFT and phonon lattice dynamics calculations, our calculated results demonstrate that by mixing different types of solids, it is possible to shift Tt to the range of practical operating temperature conditions.
The obtained results showed that by changing the mixing ratio of solid A and solid B to form mixed solid C, it is possible to shift Tt of the newly formed solid C to fit the practical CO2 capture technologies. In this study, we investigated three scenarios of mixing schemes: (i) TA > > TB, and the A component is the key component for capturing CO2. In this case, because TA is higher than TO, mixing B into A will decrease the turnover TA of the A solid to values closer to To. For example, Li2O is a very strong CO2 sorbent that forms Li2CO3. However, its regeneration from Li2CO3 only can occur at very high temperatures (TA). To move its TA to lower temperatures, Li2O can be mixed with some weak CO2 sorbents (such as SiO2, ZrO2). Our results showed that, in this way, the turnover Tt and the theoretical CO2 capture capacity of mixtures decrease as the ratios of Li2O/SiO2 or Li2O/ZrO2 decrease. (ii) TA > > TB and B component is the key part to capturing CO2. In this case, because TB is lower than TO, mixing A into B will increase the TB of the B solid to values closer to To. For example, pure MgO (as B component) has a very high theoretical CO2 capture capacity. However, its TB (250°C) is lower than the required temperature range of 300–470°C used in warm gas clean up technology. The obtained results showed that by mixing alkali metal oxides M2O (M = Na, K, Cs, Ca) or their carbonates (M2CO3) into MgO, the corresponding newly formed mixed systems have higher turnover temperatures by forming double salts through the reactions MgO + CO2 + M2CO3 = M2Mg(CO3)2. (iii) Both A and B components are active to capture CO2. In this case, the CO2 capacity of the mixture is the summation of those of A and B. Li2MSiO4 (M = Mg, Ca, etc.) and M2−xNxZrO3 (M, N = Li, Na, K) belong to this category. Those doped systems can be treated as the mixing of three solids (Li2O:MO:SiO2, M2O:N2O:ZrO2). This study summarized the results of Na2−xMxZrO3 (M = Li, Na, K, x = 0, 0.5, 1, 1.5, 2) doped sorbents. The results showed that when capturing CO2, the K-/Li- doped Na2ZrO3 have lower Tt compared to pure Na2ZrO3.
The obtained results can be used to provide insights for designing new CO2 sorbents. Therefore, although one single material taken in isolation might not be an optimal CO2 sorbent to fit the particular needs to operate at specific temperature and pressure conditions, by mixing or doping two or more materials to form a new solid, the calculated results showed that it is possible to synthesize new CO2 sorbent formulations that can fit the industrial needs. Our results also show that computational modeling can play a decisive role for identifying materials with optimal performance.
It should be pointed out that in this study we only focused on the thermodynamic properties of the CO2 capture reactions, which are essential and critical to determine whether the sorbents can capture CO2. Once the capture reaction is thermodynamically favorable, other properties (such as kinetics, mechanical resistance, toxicity, sulfur poisoning resistance, cost, etc.) also play important roles to select proper sorbent candidates for experimental validations. Generally speaking, further simulations can be performed on sorbents to optimize their performance for capturing CO2. For example, by calculating the transition states with DFT, the kinetic properties of CO2 capture reactions can be evaluated; by conducting mechanical and chemical engineering modeling with finite element method, the mechanical and sintering behaviors of sorbents can be obtained; by performing process modeling, the overall energetic and material costs can be estimated, and then some comparisons with amine-based solution capture technology can be drown. Poisoning gases (such as SOx, NOx, H2S, etc.) have big effects on the sorbent performance for CO2 capture. Further exploring the mechanisms of poisoning gases interacting with sorbents will provide useful information for designing new CO2 capture technologies.
This report was prepared as an account of work sponsored by an agency of the United States Government. Neither the United States Government nor any agency thereof, nor any of their employees, makes any warranty, express or implied, or assumes any legal liability or responsibility for the accuracy, completeness, or usefulness of any information, apparatus, product, or process disclosed, or represents that its use would not infringe privately owned rights. Reference therein to any specific commercial product, process, or service by trade name, trademark, manufacturer, or otherwise does not necessarily constitute or imply its endorsement, recommendation, or favoring by the United States Government or any agency thereof. The views and opinions of authors expressed therein do not necessarily state or reflect those of the United States Government or any agency thereof.
The author declares that the research was conducted in the absence of any commercial or financial relationships that could be construed as a potential conflict of interest.
The results in this report were presented at AIChE Spring and ACEME-15 conferences. The author wishes to acknowledge Drs. D. C. Sorescu, H. Pennline, K. Johnson, H. Pfeiffer, B. Y. Li, K. L. Zhang, D. L. King, X. S. Li, X. F. Wang, D. Luebke, J. Lekse, J. W. Halley, and B. Alcantar-Vazquez for their collaborations and fruitful discussions, and C. Wamsley (internal technical writer) for proofreading the manuscript.
Aaron, D., and Tsouris, C. (2005). Separation of CO2 from flue gas: a review. Sep. Sci. Technol. 40, 321–348. doi: 10.1081/SS-200042244
Allen, M. R., Frame, D. J., Huntingford, C., Jones, C. D., Lowe, J. A., Meinshausen, M., et al. (2009). Warming caused by cumulative carbon emissions towards the trillionth tonne. Nature 458, 1163–1166. doi: 10.1038/nature08019
Chase, M. W. J. (1998). NIST-JANAF themochemical tables, 4th edition. J. Phys. Chem. Ref. Data 9(Monogr.), 1–1951.
Chi, J. L., Zhao, L. F., Wang, B., Li, Z., Xiao, Y. H., and Duan, Y. (2014). Thermodynamic performance assessment and comparison of IGCC with solid cycling process for CO2 capture at high and medium temperatures. Int. J. Hydrogen Energy 39, 6479–6491. doi: 10.1016/j.ijhydene.2014.02.005
Duan, Y. (2011). Electronic structural and phonon properties of lithium zirconates and their capabilities of CO2 capture: a first-principle density functional approach. J. Renew. Sust. Energy 3, 013102. doi: 10.1063/1.3529427
Duan, Y. (2012). A first-principles density functional theory study of the electronic structural and thermodynamic properties of M2ZrO3 and M2CO3 (M = Na, K) and their capabilities for CO2 capture. J. Renew. Sust. Energy 4, 013109. doi: 10.1063/1.3683519
Duan, Y. (2013). Structural and electronic properties of Li8ZrO6 and its CO2 capture capabilities: an ab initio thermodynamic approach. Phys. Chem. Chem. Phys. 15, 9752–9760. doi: 10.1039/c3cp51101d
Duan, Y. (2014). Electronic, structural, phonon dynamical, and CO2 capture properties of LiMZrO3 (M = Na, K) by ab initio thermodynamic investigation. Sci. Lett. J. 3:56.
Duan, Y., and Lekse, J. (2015). Regeneration mechanisms of high-lithium content zirconates as CO2 capture sorbents: experimental measurements and theoretical investigations. Phys. Chem. Chem. Phys. 17, 22543–22547. doi: 10.1039/C5CP03968A
Duan, Y., Lekse, J., Wang, X. F., Li, B. Y., Alcantar-Vazquez, B., Pfeiffer, H., et al. (2015). Electronic structural, phonon dynamic properties, and CO2 capture capabilities of Na2−xMxZrO3 (M = Li, K): density functional theory calculations and experimental validations. Phys. Rev. Appl. 3:044013. doi: 10.1103/PhysRevApplied.3.044013
Duan, Y., Luebke, D., and Pennline, H. W. (2012a). Efficient theoretical screening of solid sorbents for CO2 capture applications. Int. J. Clean Coal Energy 1, 1–11. doi: 10.4236/ijcce.2012.11001
Duan, Y., Luebke, D. R., Pennline, H. W., Li, B. Y., Janik, M. J., and Halley, J. W. (2012b). Ab initio thermodynamic study of the CO2 capture properties of potassium carbonate sesquihydrate, K2CO3 · 1.5H2O. J. Phys. Chem. C 116, 14461–14470. doi: 10.1021/jp303844t
Duan, Y., and Parlinski, K. (2011). Density functional theory study of the structural, electronic, lattice dynamical, and thermodynamic properties of Li4SiO4 and its capability for CO2 capture. Phys. Rev. B 84:104113. doi: 10.1103/PhysRevB.84.104113
Duan, Y., Pfeiffer, H., Li, B., Romero-Ibarra, I. C., Sorescu, D. C., Luebke, D. R., et al. (2013). CO2 capture properties of lithium silicates with different ratios of Li2O/SiO2: an ab initio thermodynamic and experimental approach. Phys. Chem. Chem. Phys. 15, 13538–13558. doi: 10.1039/c3cp51659h
Duan, Y., and Sorescu, D. C. (2009). Density functional theory studies of the structural, electronic, and phonon properties of Li2O and Li2CO3: Application to CO2 capture reaction. Phys. Rev. B 79:014301. doi: 10.1103/PhysRevB.79.014301
Duan, Y., and Sorescu, D. C. (2010). CO2 capture properties of alkaline earth metal oxides and hydroxides: a combined density functional theory and lattice phonon dynamics study. J. Chem. Phys. 133, 074508. doi: 10.1063/1.3473043
Duan, Y., Zhang, B., Sorescu, D. C., and Johnson, J. K. (2011). CO2 Capture properties of M-C-O-H (M = Li, Na, K) systems: a combined density functional theory and lattice phonon dynamics study. J. Solid State Chem. 184, 304–311. doi: 10.1016/j.jssc.2010.12.005
Duan, Y., Zhang, B., Sorescu, D. C., Karl Johnson, J., Majzoub, E. H., and Luebke, D. R. (2012c). Density functional theory studies on the electronic, structural, phonon dynamical and thermo-stability properties of bicarbonates MHCO3, M = Li, Na, K. J. Phys. 24:325501. doi: 10.1088/0953-8984/24/32/325501
Duan, Y., Zhang, K., Li, X. S., King, D. L., Li, B., Zhao, L., et al. (2014). Ab initio thermodynamic study of the CO2 capture properties of M2CO3 (M = Na, K)- and CaCO3-promoted MgO sorbents towards forming double salts. Aerosol Air Qual. Res. 14, 470–479. doi: 10.4209/aaqr.2013.05.0178
Gray, M. L., Champagne, K. J., Fauth, D., Baltrus, J. P., and Pennline, H. (2008). Performance of immobilized tertiary amine solid sorbents for the capture of carbon dioxide. Int. J. Greenhouse Gas Control 2, 3–8. doi: 10.1016/S1750-5836(07)00088-6
Haszeldine, R. S. (2009). Carbon capture and storage: how green can black be? Science 325, 1647–1652. doi: 10.1126/science.1172246
Li, B. Y., Duan, Y., Luebke, D., and Morreale, B. (2013). Advances in CO2 capture technology: a patent review. Appl. Energy 102, 1439–1447. doi: 10.1016/j.apenergy.2012.09.009
Ochoa-Fernandez, E., Rusten, H. K., Jakobsen, H. A., Ronning, M., Holmen, A., and Chen, D. (2005). Sorption enhanced hydrogen production by steam methane reforming using Li2ZrO3 as sorbent: sorption kinetics and reactor simulation. Catal. Today 106, 41–46. doi: 10.1016/j.cattod.2005.07.146
Pfeiffer, H., and Bosch, P. (2005). Thermal stability and high-temperature carbon dioxide sorption on hexa-lithium zirconate (Li6Zr2O7). Chem. Mater. 17, 1704–1710. doi: 10.1021/cm047897+
White, C. M., Strazisar, B. R., Granite, E. J., Hoffman, J. S., and Pennline, H. W. (2003). Separation and capture of CO2 from large stationary sources and sequestration in geological formations-coalbeds and deep saline aquifers. J. Air Waste Manag. Assoc. 53, 645–715. doi: 10.1080/10473289.2003.10466206
Zhang, B., Duan, Y., and Johnson, J. K. (2012). First-principles density functional theory study of CO2 capture with transition metal oxides and hydroxides. J. Chem. Phys. 136, 064516. doi: 10.1063/1.3684901
Zhang, K. L., Li, X. H. S., Duan, Y., King, D. L., Singh, P., and Li, L. Y. (2013). Roles of double salt formation and NaNO3 in Na2CO3-promoted MgO absorbent for intermediate temperature CO2 removal. Int. J. Greenhouse Gas Control 12, 351–358. doi: 10.1016/j.ijggc.2012.11.013
Keywords: CO2 capture, mixed solid sorbent, ab initio thermodynamics, turnover temperature shift, mixing ratio
Citation: Duan Y (2015) Ab initio thermodynamic approach to identify mixed solid sorbents for CO2 capture technology. Front. Environ. Sci. 3:69. doi: 10.3389/fenvs.2015.00069
Received: 24 July 2015; Accepted: 02 October 2015;
Published: 15 October 2015.
Edited by:
Abdirashid A. Elmi, Kuwait University, KuwaitReviewed by:
Fabrizio Scala, University of Napoli Federico II, ItalyCopyright © 2015 Duan. This is an open-access article distributed under the terms of the Creative Commons Attribution License (CC BY). The use, distribution or reproduction in other forums is permitted, provided the original author(s) or licensor are credited and that the original publication in this journal is cited, in accordance with accepted academic practice. No use, distribution or reproduction is permitted which does not comply with these terms.
*Correspondence: Yuhua Duan, eXVodWEuZHVhbkBuZXRsLmRvZS5nb3Y=
Disclaimer: All claims expressed in this article are solely those of the authors and do not necessarily represent those of their affiliated organizations, or those of the publisher, the editors and the reviewers. Any product that may be evaluated in this article or claim that may be made by its manufacturer is not guaranteed or endorsed by the publisher.
Research integrity at Frontiers
Learn more about the work of our research integrity team to safeguard the quality of each article we publish.