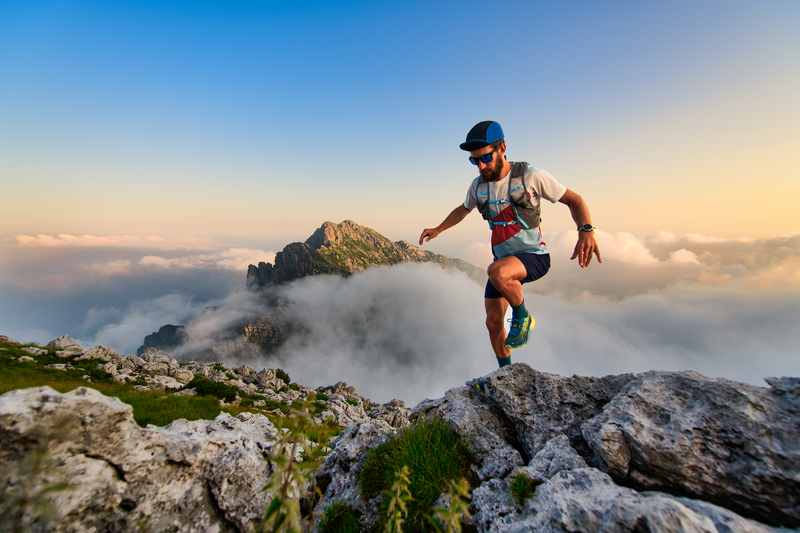
95% of researchers rate our articles as excellent or good
Learn more about the work of our research integrity team to safeguard the quality of each article we publish.
Find out more
ORIGINAL RESEARCH article
Front. Environ. Sci. , 23 March 2015
Sec. Environmental Toxicology
Volume 3 - 2015 | https://doi.org/10.3389/fenvs.2015.00019
This article is part of the Research Topic Redox homeostasis managers in plants under environmental stresses View all 18 articles
Salt stress, considered as one of the major environmental factors, decreases crop productivity world-wide and hence, investigations are being made to understand the cellular basis of salt tolerance in plants. In our earlier studies, maintenance of redox homeostasis and energetics were found as key determinants of salt tolerance in a halophyte Sesuvium portulacastrum (high salt accumulator). The redox homeostasis is defined as integrated ratio of different redox couples present inside the cell. In recent years, it has also been proposed as general stress response regulator in plants, bacteria as well as animals. In view of this, present study was performed to compare responses of redox state and energetics of S. portulacastrum with a glycophyte Brassica juncea (low salt accumulator). The data revealed activation of antioxidant defense in S. portulacastrum which either avoided or delayed the accumulation of different reactive oxygen species (ROS). In contrast, due to the lack of co-ordination, although the non-enzymatic antioxidants were increased, significant oxidative damage was seen in B. juncea. Further, the decreased NADPH oxidase activity suggested that basal redox signaling was also affected in B. juncea. In order to correlate these changes with chloroplastic and mitochondrial electron transport chain, NADP/NADPH and NAD/NADH ratios were measured. The NADP/NADPH ratio suggested that the process of photosynthesis was minimally affected in S. portulacastrum which might have contributed to its lower level of ROS under salt stress. The comparatively lower NAD/NADH and ATP/ADP ratios in S. portulacastrum as compared to B. juncea indicated the active and better utilization of energy generated to support different processes associated with salt tolerance. Thus, the findings suggest that co-ordinated regulation of antioxidant defense to avoid oxidative damage and proper utilization of energy are the key determinants of salt-tolerance in plants.
A major challenge toward world agriculture involves production of 70% more food crop for an additional 2.3 billion people by 2050 (FAO, 2009). Salinity is a major stress limiting the increase in the demand for food crops. More than 20% of cultivated land worldwide (~ about 45 hectares) is affected by salt stress and the amount is increasing day by day. Plants on the basis of adaptive evolution can be classified roughly into two major types: the halophytes (that can withstand salinity) and glycophytes (that cannot withstand salinity and eventually die). Majority of crop species belong to this second category. Thus salinity is considered as one of the most important stresses that hamper crop productivity worldwide (Gupta and Huang, 2014). During salinity stress, availability of atmospheric CO2 is reduced because of an increased stomatal closure in order to avoid water loss via transpiration and hence, consumption of NADPH by the Calvin cycle is decreased. Due to the over-reduction of electron transport chain, electrons get misleaded from oxygen to form reactive oxygen species (ROSs) like superoxide radicals (O2-) and hydrogen peroxide (H2O2). Additionally, to meet the increased energy demand of the cell which can support different defense processes such as enhanced antioxidant capacity, osmolytes biosynthesis, ion transport and vacuolar sequestration, the activity of mitochondrial electron transport is also increased which further contributes to the generation of ROSs (Munns and Tester, 2008). Plants have complex antioxidant defense mechanisms including superoxide dismutase (SOD) and the ascorbate (ASC)-glutathione (GSH) cycle (Mittler, 2002). SOD constitutes the first line of defense converting O•−2 to hydrogen peroxide (H2O2), which is further reduced to water and oxygen by ascorbate peroxidase (APX) and catalase (CAT). APX uses two molecules of ASC to reduce H2O2 to water, with the concomitant generation of two molecules of monodehydroascorbate (MDHA), whereas CAT does not need any reductant for action against H2O2. Regeneration of ASC from MDHA occurs in sequential steps and utilizes GSH. This results in generation of oxidized glutathione (GSSG), which is in turn, re-reduced to GSH by NADPH, a reaction catalyzed by glutathione reductase (GR). Ascorbate and GSH both can accumulate in millimolar concentrations in cells and function as molecular antioxidants, in addition to serving various other roles, reacting directly with various ROS (Noctor and Foyer, 1998).
Among different experimental approaches adopted to understand plant responses to salt stress, one of the strategies is to compare halophytes and glycophytes. In this context, Thellungiella salsuginea is widely used as halophytic model and its responses are compared with its close relative Arabidopsis thaliana. The lipidomic analysis has revealed that remodeling of plastidic lipids is important for maintaining the integrity and fluidity of plastidic membranes which contributes to PEG-induced osmotic tolerance of T. salsuginea (Yu and Li, 2014). A proteomics study has been done to identify novel proteins associated with high salt tolerance of T. salsuginea (Vera-Estrella et al., 2014). The physiological responses of T. salsuginea and A. thaliana have also been studied to understand the mechanism of boron exclusion and tolerance in plants (Lamdan et al., 2012). The species of Eutrema such as Eutrema parvulum and E. salsugineum have been contrasted with A. thaliana to understand the regulatory role of genes associated with aldehyde dehydrogenase gene superfamily (Hou and Bartels, 2015). Using Mesembryanthemum crystallinum (halophyte) and Brassica juncea, the mechanism of nickel accumulation and tolerance has been studied (Amari et al., 2014). The halophyte Cakile maritima has been compared with Brassica juncea to understand cadmium accumulation and tolerance (Taamalli et al., 2014). In the same line, Sesuvium portulacastrum is a mangrove associate which is known for its high capacity to accumulate salt. Its physiological, redox, and energetics behavior toward salt stress has been reported (Lokhande et al., 2011). In the present study, these responses were compared with that of B. juncea which is a glycophyte and low salt accumulator. The findings confirmed the significance of co-ordinated antioxidant responses and effective utilization of energy as important determinants of salt tolerance in plants.
The seeds of Indian mustard (B. juncea cv. TM- 2) were surface sterilized with 30% ethanol for 3 min and then washed thoroughly to remove any traces of ethanol. They were then allowed to germinate in plastic pots containing sand: soil (1:1) mixture at 25°C under light providing 115 μmol photons m−2s−1 illumination, with 12 h photoperiod. After 6-days of germination, water was changed with ½strength Murashige and Skoog's (MS) medium (Murashige and Skoog, 1962). After 15-days of germination, plants having secondary leaves were used for salt treatment. The Sesuvium—MH clone (Lokhande et al., 2010) was used for the present study. Four nodal sectors (~4.0 cm) containing single pre-existing axillary bud with two opposite leaves were planted in the plastic pots containing sand: soil (1:1) mixture. The 45-day-old plants were used for the present study. For salt treatment, B. juncea was subjected to 250 mM NaCl, while S. portulacastrum was given two different NaCl concentrations (250 or 1000 mM). All the salt solutions were prepared in ½strength MS medium. An independent set with no NaCl was maintained as control. At 2, 4, and 8-days after treatment, secondary leaves were harvested and stored at -80°C until further analyses. The NaCl concentrations were selected on the basis of preliminary experiments to assess plant tolerance in terms of growth to various NaCl concentrations (100–300 mM for B. juncea and 200–1000 mM for S. portulacastrum).
For the estimation of hydrogen peroxide (H2O2) levels, plant samples were homogenized in 0.5% (w/v) trichloroacetic acid (TCA) in an ice bath and centrifuged at 14,000 × g for 15 min at 4°C. For H2O2 determination, 0.5 ml of supernatant was mixed with 0.5 ml 100 mM potassium phosphate buffer (pH 7.0) and 1 ml of freshly prepared 1 M potassium iodide. Reaction was allowed to develop for 1 h in dark and absorbance was measured at 390 nm (Alexieva et al., 2001). The amount of H2O2 was calculated from a standard curve prepared using the known concentrations of H2O2. The rate of superoxide radicals (O•−2) production was measured following the method of Chaitanya and Naithani (1994). About 500 mg of fresh plant samples were homogenized under N2 atmosphere in cold (0-4°C) in 100 mM sodium phosphate buffer (pH 7.2) containing 10 mM sodium azide to inhibit SOD activity. After centrifugation at 22,000 × g at 4°C for 20 min, the level of O•−2 was measured in the supernatant by its capacity to reduce NBT (extinction coefficient; ε = 12.8 mM−1 cm−1). The reaction mixture (1 ml) contained 100 mM sodium phosphate buffer (pH 7.8), 0.05% (w/v) NBT (Nitro blue tetrazolium chloride), 10 mM sodium azide and 0.2 ml of extract. Absorbance was measured at 580 nm at 0 and 60 min. The level of O•−2 is expressed as an increase in absorbance per min per gram fresh weight. Lipid peroxidation was determined by estimation of the malondialdehyde (MDA) content following Heath and Packer (1968) as described previously (Srivastava et al., 2006).
Plants were homogenized in 20 mM HEPES [4-(2-hydroxyethyl)-1-piperazineethanesulfonic acid; pH 7.0] containing 1 mM EDTA, 1 mM EGTA, 1 mM phenylmethyl sulfonyl fluoride (PMSF) and 2.5% polyvinylpyrrolidone (PVP) under chilled conditions. Homogenate was squeezed through four layers of cold cheese cloth and centrifuged at 12,000 × g for 15 min at 4°C. Protein content of supernatant was measured following the protocol of Lowry et al. (1951). NADPH-dependent O•−2 generation was measured using NBT as an electron acceptor, whose reduction was monitored at 530 nm. Monoformazan concentrations (and therefore O•−2 concentrations) were calculated using ε of 12.8 mM−1 cm−1. The reaction mixture consisted of Tris buffer (50 mM Tris-HCI, pH 7.4), 5 mM NBT, 1 mM MgCl2, 1 mM CaCl2, 5 mM NADPH and a suitable aliquot of enzyme extract. The selective reduction of NBT by O•−2 was calculated from the difference in the NBT reduction rate in the presence and absence of SOD (50–100 units ml−1; Sigma, USA). No NBT reduction with NADPH was observed in the absence of protein fractions.
For the assay of antioxidant enzymes, plant samples (500 mg) were homogenized in 100 mM chilled potassium phosphate buffer (pH 7.0) containing 1 mM EDTA, 1 mM PMSF and 1% PVP (soluble, MW 3,60,000) at 4°C. Homogenate was squeezed through four layers of cold cheese cloth and the extract thus obtained was centrifuged at 15,000 g for 15 min at 4°C. For all enzyme assays, respective sample and reagent blanks were run in duplicate. The activity of SOD (EC 1.15.1.1) was assayed following the method of Beauchamp and Fridovich (1971). The reaction mixture for SOD activity assay contained 40 mM phosphate buffer (pH 7.8), 13 mM methionine, 75 μM NBT, 2 μM riboflavin, 0.1 mM EDTA and a suitable aliquot of enzyme extract. After the reaction under light for 15 min, the absorbance was taken at 560 nm. One unit of activity is the amount of protein required to inhibit 50% initial reduction of NBT under light. For measurement of the CAT (EC 1.11.1.6) activity, extraction was done in the buffer containing 50 mM Tris–HCl (pH 7.0), 0.1 mM EDTA, 1 mM PMSF and 0.3 g g−1 fw PVP. Activity was measured by the method of Aebi (1974). The reaction mixture comprised of 50 mM sodium phosphate buffer (pH 7.0), 20 mM H2O2 and suitable aliquot of enzyme. Decrease in the absorbance was taken at 240 nm (molar extinction coefficient of H2O2 was 0.04 cm2 μmol−1). Enzyme activity was expressed as units mg−1 protein. The activity of APX (EC 1.11.1.11) was measured by estimating the rate of ascorbate oxidation (extinction coefficient 2.8 mM−1cm−1). The reaction mixture contained 50 mM phosphate buffer (pH 7.0), 0.1 mM H2O2, 0.5 mM sodium ascorbate, 0.1 mM EDTA and suitable aliquot of enzyme. The change in absorbance was monitored at 290 nm (Nakano and Asada, 1981) and enzyme activity was expressed as units mg−1 protein. For the estimation of the GR (EC 1.6.4.2) activity plant material was extracted in 0.1 M potassium phosphate buffer (pH 7.5) containing 0.5 mM EDTA. Activity was assayed by following the method of Smith et al. (1988). The reaction was started by adding following in order; 1.0 ml of 0.2 M potassium phosphate buffer (pH 7.5) containing 1 mM EDTA, 500 μl 3 mM 5,5′-dithiobis (2-nitrobenzoic acid) in 0.01 M phosphate buffer (pH 7.5), 250 μl H2O, 100 μl 2 mM NADPH, 50 μl enzyme extract, and 100 μl 20 mM GSSG. The increase in absorbance was monitored for 5 min at 412 nm. The rate of enzyme activity was calculated using standard curve prepared by known amounts of GR (Sigma, USA). Activity of enzyme was expressed as units mg−1 protein.
The level of reduced (GSH) and oxidized (GSSG) glutathione was determined fluorometrically using o–phthaldialdehyde (OPT) as fluorophore (Hissin and Hilf, 1976). The level of total, reduced and oxidized ascorbate (ASC) contents in plants was measured following the protocol of Gillespie and Ainsworth (2007). Plant samples (50 mg) were homogenized in 1 ml 6% trichloroacetic acid (TCA) under chilled conditions and centrifuged at 13,000 × g for 5 min at 4°C. To 200 μl of sample 100 μl 75 mM phosphate buffer (pH 7.0) was added. In total ASC, 100 μl DTT (dithiothreitol; 10 mM) was added and incubated for 10 min at room temperature to reduce the pool of oxidized ASC. Then, 100 μl NEM (N-ethylmaleimide; 0.5%) was added to remove excess DTT. For reaction, 500 μl 10% TCA, 400 μl 43% orthophosphoric acid, 400 μl 4% 2,2′-bipyridyl, and 200 μl 3% FeCl3 were added to all tubes. After incubation at 37°C for 1 h, absorbance was measured at 525 nm. The level of dehydroascorbate (DHA) was calculated by subtracting ASC values from total ASC.
The analysis of adenine and pyridine nucleotides was performed by High Performance Liquid Chromatography (HPLC) as described previously by Srivastava et al. (2011). In brief, samples (100 mg) were subjected to either acid extraction using 0.6 M perchloric acid (for the measurement of ATP, ADP, NADP, and NAD) or alkaline extraction using 0.5 M potassium hydroxide (for the measurement of NADPH and NADH). The extract was centrifuged at 14,000 × g at 4°C for 10 min followed by neutralization with either 0.5 M KOH or 1 M KH2PO4, respectively and re-centrifuged at 14,000 × g at 4°C for 10 min to remove the precipitate. Supernatant was filtered using 0.22 μm syringe filters and used for the HPLC injection. The mobile phase consisted of 0.1 M KH2PO4 solution at pH 6.0 (Buffer A) and a 0.1 M KH2PO4 solution at pH 6.0, containing 10% (v/v) of CH3OH (Buffer B). The chromatographic conditions were as follows: 8 min at 100% of buffer A, 7 min at up to 25% of buffer B, 2.5 min at up to 90% of buffer B, 2.5 min at up to 100% of buffer B, held for 7 min at 100% B, 5 min at up to 100% buffer A and held for 8 min at 100% buffer B to restore the initial condition. The flow rate was 1 mL/min and detection was performed at 254 nm (Waters 996, PDA detector). Separation was performed on a 10 μm C18 analytical column (250 × 4.6 mm) equipped with a guard column. The peaks were identified using the standard samples. The analytical recovery was tested by adding a known amount of standard compound prior to extraction and recovery was found to be 94 to 100% for different compounds. The data were analyzed using Empower software.
The experiments were carried out in a randomized block design. One–Way analysis of variance (ANOVA) was done on all the data to confirm the variability of data and validity of results and Duncan's multiple range test (DMRT) was performed to determine the significant difference between treatments using the statistical software SPSS 10.0.
In S. portulacastrum, no significant change in MDA level was observed at any salt concentration and duration. The increase in ROS levels was also limited to longer duration. At 8 d, H2O2 level was increased under both 250 and 1000 mM NaCl treatment; while O•−2 level was increased only under 1000 mM NaCl treatment as compared to that of control (Figures 1A–C). By contrast, in B. juncea, time-dependent increase in MDA level was observed (Figure 1C) and ROS levels also increased significantly. The maximum increase of 2.5- and 4.8-fold in O•−2 and H2O2 levels were observed at 8 d after treatment, as compared to that of control (Figures 1A–B).
Figure 1. Time dependent modulation of superoxide radicals (A), hydrogen peroxide (B) and malondialdehyde (C) in NaCl-exposed Sesuvium portulacastrum and Brassica juncea. All values represent the mean of three replicates. Different letters indicate significantly different values at a particular duration (DMRT, P = 0.01).
The activity of NADPH oxidase was not affected significantly in S. portulacastrum except for the 22% increase at 1000 mM NaCl after 2 d treatment. In B. juncea, NADPH oxidase activity declined significantly at all the durations as compared to that of control (Figure 2).
Figure 2. Time dependent modulation of NADPH oxidase activity in NaCl exposed Sesuvium portulacastrum and Brassica juncea. All values represent the mean of three replicates. Different letters indicate significantly different values at a particular duration (DMRT, P = 0.01).
In S. portulacastrum, dose-dependent increase in SOD activity was observed until 4 d, with the maximum of 30% under 1000 mM NaCl as compared to that of control. At 2 and 8 d after treatment, SOD activity was almost unchanged, except at 1000 mM at 2 d where it was increased by 20% as compared to that of control (Figure 3A). Unlike SOD, CAT activity was increased at all the durations, except at 8 d where the increase was observed only at 1000 mM NaCl concentration (Figure 3B). The APX activity increased at both NaCl concentrations; however, maximum of 2.8-fold was observed under 250 mM at 2 d after treatment as compared to that of control. At 4 and 8 d, comparable increase in APX activity was observed under both 250 and 1000 mM NaCl as compared to that of control (Figure 3C). The GR activity was almost unchanged at both NaCl concentrations and all durations (Figure 3D). In B. juncea, except for CAT, activities of SOD, APX, and GR decreased at all the durations (Figures 3A–D).
Figure 3. Time dependent modulation of activities of superoxide dismutase (SOD; A), catalase (CAT; B), ascorbate peroxide (APX; C), and glutathione reductase (GR; D) in NaCl exposed Sesuvium portulacastrum and Brassica juncea. All values represent the mean of three replicates. Different letters indicate significantly different values at a particular duration (DMRT, P = 0.01).
In S. portulacastrum, GSH level was unaltered until 4 d; however; at 8 d it showed significant reduction with the maximum of 40% under 1000 mM NaCl as compared to that of control (Figure 4A). The GSH/GSSG ratio showed an increase in comparison to control until 4 d at 250 mM NaCl but was at par to control at 1000 mM NaCl. At 8 d, GSH/GSSG ratio was decreased with the maximum of 66% under 1000 mM NaCl as compared to that of control (Figure 4B). In B. juncea, GSH level increased beyond 2 d and at 8 d, it was 2.5-fold higher than that of control. The GSH/GSSG ratio was at par with control till 4 d and at 8 d, it was 1.8-fold higher than that of control.
Figure 4. Time dependent modulation of reduced glutathione (GSH; A), reduced and oxidized glutathione ratio (GSH/GSSG; B), ascorbate (ASC; C), reduced and oxidized ascorbate ratio (ASC/DHA; D) in NaCl exposed Sesuvium portulacastrum and Brassica juncea. All values represent the mean of three replicates. Different letters indicate significantly different values at a particular duration (DMRT, P = 0.01).
Under NaCl stress, a distinct increase in ASC level was observed in both S. portulacastrum and B. juncea, however, the level of increase was comparatively higher in B. juncea (Figure 4C). The ASC/DHA ratio was unchanged in S. portulacastrum at 250 mM NaCl; however, at 1000 mM, it significantly increased. In contrast, B. juncea showed a significant decrease of ASC/DHA ratio at all the durations (Figure 4D).
In S. portulacastrum, ATP level was declined at both 250 and 1000 mM NaCl (Supplementary Figure 1A), hence, ATP/ADP ratio declined significantly (Figure 5A). Similar profile was also observed for NADP/NADPH and NAD/NADH ratios. The maximum decrease in these ratios was observed under 1000 mM NaCl at 8 d after treatment (Figures 5B,C). In B. juncea, ATP/ADP ratio remained at par with control, except at 4 d at which it was increased by 1.5-fold as compared to that of control (Figure 5A). The NADP and NADPH levels although increased (Supplementary Figures 2, 3), NADP/NADPH ratio was reduced with the maximum of 60% at 8 d after treatment as compared to that of control (Figure 5B). In contrast, the NAD/NADH ratio was increased beyond 2 d of stress treatment (Figure 5C).
Figure 5. Time dependent modulation of ATP/ADP (A), NADP/NADPH (B), NAD/NADH (C) ratios in NaCl exposed Sesuvium portulacastrum and Brassica juncea. All values represent mean of three replicates.
The salinity stress in plants is known to drastically reduce its growth and productivity (Gupta and Huang, 2014). Thus, in order to maintain the crop productivity in saline affected regions, it is essential to understand the mechanism of salt toxicity in plants. Generally, most crop plants are glycophytes and can withstand salt concentration in the range of 50–250 mM; however, there are specific type of plants referred as halophytes which can tolerate upto 1 M salt concentration. In an earlier study, physiological responses of a halophyte, S. portulacastrum have been reported and redox homeostasis and energetics were found to be the key determinants of salt tolerance (Lokhande et al., 2011). To further strengthen this hypothesis, in the present work, similar responses were studied in B. juncea (Indian mustard – a glycophyte) and compared with that of S. portulacastrum.
In the presence of salt stress, series of events leading to perturbation of cellular metabolism are: less water availability, stomata closure, altered gaseous exchange, inhibition of photosynthesis, effect on electron flow in ETC in chloroplast and mitochondria, increase in the production of ROS and disturbed status of adenine (ATP) and pyridine nucleotides (NADH, NADPH). Thus, the oxidative damage and altered energetics are mainly responsible for affecting the general metabolism and plant growth under salt stress (Srivastava et al., 2011). In S. portulacastrum, the increase in ROS levels was seen only at longer duration; however, in B. juncea, the increase in ROS and MDA levels were seen as early as 2 d after treatment (Figures 1A–C). This clearly suggested that S. portulacastrum has better ability to avoid or delay the oxidative damage under salt stress conditions. In recent years, significant progress has been made with respect to ROS function in plants and they are now not seen as negative by-product of oxidative metabolism but they are known to serve as important signaling mediators under stress (Gilroy et al., 2014). The ROS based signaling is termed as “redox signaling” and is important for growth and survival of the plants under normal as well as salt stress conditions (Srivastava and Suprasanna, in press). There are many pro-oxidant enzymes in plants which are responsible for the ROS formation. NADPH oxidase is one such pro-oxidant which is an important regulator of calcium signaling and downstream signal transduction associated with salt tolerance (Marino et al., 2012; Drerup et al., 2013). The NADPH oxidase activity in S. portulacastrum was unaffected while in B. juncea, it decreased significantly (Figure 2). This indicates that apart from oxidative damage, the basal ROS signaling is also disturbed in glycophytes under salt stress. Thus, differential ability of halophytes and glycophytes to perceive stress seems to be responsible for differential stress response. The NADPH oxidase might be one of the possible candidates responsible for this; however, this needs to be investigated further.
Significant research has been conducted to establish that antioxidant defense is important for maintaining proper plant growth under different abiotic stress conditions, especially halophytes (Boestfleisch et al., 2014; Canalejo et al., 2014; Yildiztugay et al., 2014). In the same line, to see how the stress imposition and oxidative stress in two plant species (glycophytes and halophyte) affected their antioxidant metabolism and vice versa, activities of enzymatic antioxidant and levels of non-enzymatic antioxidants were measured. The enzymatic antioxidants showed a significant decline except CAT in B. juncea, whereas in S. portulacastrum, there was either a increase or no change (Figures 3A–D). Apart from suggesting the better ability of S. portulacastrum to activate its antioxidant defense under stress, this also indicated that halophytic enzymes are comparably more robust and stable than glycophytic enzymes. In recent years, various efforts have been made where antioxidant gene from a halophyte has been used to make transgenic glycophytes with higher salt tolerance. For instance, cytosolic copper/zinc SOD from a mangrove plant Avicennia marina has been used to increase the abiotic stress tolerance in rice (Prashanth et al., 2008). The peroxisomal APX from halophyte Salicornia brachiata was used to confer salt and drought tolerance in tobacco (Singh et al., 2014). Among molecular antioxidants, ASC and GSH are involved in the functioning of ASC-GSH cycle and can also independently function as antioxidants. Hence, maintenance of ASC/DHA and GSH/GSSG ratio is necessary to allow antioxidant functions to operate properly. In B. juncea, the GSH/GSSG and ASC/DHA ratio was either maintained or decreased which indicated plant's inability to stimulate their ASC and GSH regenerating systems as observed in the case of GR. Thus, despite an increase in absolute ASC and GSH, ROS levels continued to increase significantly. On the contrary in S. portulacastrum, both ASC/DHA and GSH/GSSG showed increasing trend; except for the decrease in GSH/GSSG ratio at 1000 mM NaCl which signifies the active utilization of GSH to avoid the oxidative damage at such a higher salt concentration.
Apart from the lack of co-ordinated activation of antioxidant defense, the over-reduction of electron transport chain (ETC) is also an important contributor of oxidative damage under stress. The over-reduced ETC in chloroplasts and mitochondria apparently result in significant decline in ratios of NADP/NADPH and NAD/NADH, respectively (Munns and Tester, 2008). In the present study, both the plants demonstrated the decreasing trend in NADP/NADPH ratio; however the extent of decrease was higher in B. juncea than S. portulacastrum. This suggests two possibilities, first: either the photosynthesis was not affected to a significant level in S. portulacastrum; second: there might be the activation of alternative salvage pathway to utilize reducing equivalents, such as photorespiration. Although, comparison of glycophyte Arabidopsis and its halophytic relative Thellungiella showed that photosynthesis and gas exchange in halophyte is minimally affected under salt conditions (Stepien and Johnson, 2009), the contribution of salvage pathway cannot be completely ignored, however this needs further investigation. To see the effects on plant energetic, the levels of adenylate (ATP, ADP) and pyridine nucleotides (NAD, NADH) were measured. In B. juncea, NaCl stress increases the NAD/NADH ratio which signifies the effect on energy metabolism. However, the ATP/ADP ratio remained unchanged which suggests that ATP formed is not actively utilized. By contrast, in S. portulacastrum, significant consumption of ATP and NAD was observed which led to the decline in ATP/ADP and NAD/NADH ratios. Thus, apart from the ATP and NAD generation, their proper channelization or consumption is also important to activate different mechanisms responsible for imparting salt tolerance in plants.
In summary, two plant systems (S. portulacastrum – a halophyte and B. juncea – a glycophyte) differing in their salt-tolerance ability have been compared. The findings reveal better antioxidant defense to avoid oxidative damage and proper energy consumption as key determinants of salinity tolerance in these two contrasting plants. The differential response of NADPH oxidase is also seen which needs to be investigated further in the context of stress perception and downstream signaling.
The authors declare that the research was conducted in the absence of any commercial or financial relationships that could be construed as a potential conflict of interest.
The Supplementary Material for this article can be found online at: http://www.frontiersin.org/journal/10.3389/fenvs.2015.00019/abstract
Aebi, H. (1974). “Catalase,” in Methods of Enzymatic Analysis, ed H. U. Bergenmeyer (Weinheim: Verlag Chemie), 673–684. doi: 10.1016/B978-0-12-091302-2.50032-3
Alexieva, V., Sergiev, I., Mapelli, S., and Karanov, E. (2001). The effect of drought and ultraviolet radiation on growth and stress markers in pea and wheat. Plant Cell Environ. 24, 1337–1344. doi: 10.1046/j.1365-3040.2001.00778.x
Amari, T., Ghnaya, T., Debez, A., Taamali, M., Ben Youssef, N., Lucchini, G., et al. (2014). Comparative Ni tolerance and accumulation potentials between Mesembryanthemum crystallinum (halophyte) and Brassica juncea: Metal accumulation, nutrient status and photosynthetic activity. J. Plant Physiol. 171, 1634–1644. doi: 10.1016/j.jplph.2014.06.020
PubMed Abstract | Full Text | CrossRef Full Text | Google Scholar
Beauchamp, C., and Fridovich, I. (1971). Superoxide dismutase: improved assays and an assay applicable to acrylamide gels. Anal. Biochem. 44, 276–287. doi: 10.1016/0003-2697(71)90370-8
PubMed Abstract | Full Text | CrossRef Full Text | Google Scholar
Boestfleisch, C., Wagenseil, N. B., Buhmann, A. K., Seal, C. E., Wade, E. M., Muscolo, A., et al. (2014). Manipulating the antioxidant capacity of halophytes to increase their cultural and economic value through saline cultivation. AoB Plants 6:plu046. doi: 10.1093/aobpla/plu046
PubMed Abstract | Full Text | CrossRef Full Text | Google Scholar
Canalejo, A., Martínez-Domínguez, D., Córdoba, F., and Torronteras, R. (2014). Salt tolerance is related to a specific antioxidant response in the halophyte cordgrass, Spartina densiflora. Estuar. Coast. Shelf Sci. 146, 68–75. doi: 10.1016/j.ecss.2014.05.017
Chaitanya, K. S. K., and Naithani, S. C. (1994). Role of superoxide, lipid peroxidation and superoxide dismutase in membrane perturbation during loss of viability in seeds of Shorea robusta Gaertn. f. New Phytol. 26, 623–627. doi: 10.1111/j.1469-8137.1994.tb02957.x
Drerup, M., Schlücking, K., Hashimoto, K., Manishankar, P., Steinhorst, L., Kuchitsu, K., et al. (2013). The Calcineurin B-Like Calcium Sensors CBL1 and CBL9 Together with Their Interacting Protein Kinase CIPK26 Regulate the Arabidopsis NADPH Oxidase RBOHF. Mol. Plant 6, 559–569. doi: 10.1093/mp/sst009
PubMed Abstract | Full Text | CrossRef Full Text | Google Scholar
FAO. (2009). High Level Expert Forum—How to Feed the World in 2050, Economic and Social Development. Rome: Food and Agricultural Organization of the United Nations.
Gillespie, K. M., and Ainsworth, E. A. (2007). Measurement of reduced, oxidized and total ascorbate content in plants. Nat. Protoc. 2, 871–874. doi: 10.1038/nprot.2007.101
PubMed Abstract | Full Text | CrossRef Full Text | Google Scholar
Gilroy, S., Suzuki, N., Miller, G., Choi, W. G., Toyota, M., Devireddy, A. R., et al. (2014). A tidal wave of signals: calcium and ROS at the forefront of rapid systemic signaling. Trends Plant Sci. 19, 623–630. doi: 10.1016/j.tplants.2014.06.013
PubMed Abstract | Full Text | CrossRef Full Text | Google Scholar
Gupta, B., and Huang, B. (2014). Mechanism of salinity tolerance in plants: physiological, biochemical, and molecular characterization. Int. J. Genomics 2014:701596. doi: 10.1155/2014/701596
PubMed Abstract | Full Text | CrossRef Full Text | Google Scholar
Heath, R. L., and Packer, L. (1968). Photoperoxidation in isolated chloroplasts 1. Kinetics and stoichiometry of fatty acid peroxidation. Arch. Biochem. Biophy. 125, 189–198. doi: 10.1016/0003-9861(68)90654-1
PubMed Abstract | Full Text | CrossRef Full Text | Google Scholar
Hissin, P. J., and Hilf, R. (1976). A fluorometric method for determination of oxidized and reduced glutathione in tissues. Anal. Biochem. 74, 214–226. doi: 10.1016/0003-2697(76)90326-2
PubMed Abstract | Full Text | CrossRef Full Text | Google Scholar
Hou, Q., and Bartels, D. (2015). Comparative study of the aldehyde dehydrogenase (ALDH) gene superfamily in the glycophyte Arabidopsis thaliana and Eutrema halophytes. Ann. Bot. 115, 465–479. doi: 10.1093/aob/mcu152
PubMed Abstract | Full Text | CrossRef Full Text | Google Scholar
Lamdan, N. L., Attia, Z., Moran, N., and Moshelion, M. (2012). The Arabidopsis-related halophyte Thellungiella halophila: boron tolerance via boron complexation with metabolites?. Plant Cell Environ. 35, 735–746. doi: 10.1111/j.1365-3040.2011.02447.x
PubMed Abstract | Full Text | CrossRef Full Text | Google Scholar
Lokhande, V. H., Nikam, T. D., Ghane, S. G., and Suprasanna, P. (2010). In vitro culture, plant regeneration and clonal behaviour of Sesuvium portulacastrum (L.) L.: a prospective halophyte. Physiol. Mol. Biol. Plants 16, 187–193. doi: 10.1007/s12298-010-0020-z
PubMed Abstract | Full Text | CrossRef Full Text | Google Scholar
Lokhande, V. H., Srivastava, A. K., Srivastava, S., Nikam, T. D., and Suprasanna, P. (2011). Regulated alterations in redox and energetic status are the key mediators of salinity tolerance in the halophyte Sesuvium portulacastrum (L.) L. Plant Growth Regul. 65, 287–298. doi: 10.1007/s10725-011-9600-3
Lowry, O. H., Rosenbrough, N. J., Farr, A. L., and Randall, R. J. (1951). Protein measurement with folin phenol reagent. J. Biol. Chem. 193, 265–275.
Marino, D., Dunand, C., Puppo, A., and Pauly, N. (2012). A burst of plant NADPH oxidases. Trends Plant Sci. 17, 9–15. doi: 10.1016/j.tplants.2011.10.001
PubMed Abstract | Full Text | CrossRef Full Text | Google Scholar
Mittler, R. (2002). Oxidative stress, antioxidants and stress tolerance. Trends Plant Sci. 7, 405–410. doi: 10.1016/S1360-1385(02)02312-9
PubMed Abstract | Full Text | CrossRef Full Text | Google Scholar
Munns, R., and Tester, M. (2008). Mechanisms of salinity tolerance. Ann. Rev. Plant Biol. 59, 651–681. doi: 10.1146/annurev.arplant.59.032607.092911
PubMed Abstract | Full Text | CrossRef Full Text | Google Scholar
Murashige, T., and Skoog, F. (1962). A revised medium for rapid growth and bioassays with tobacco tissue cultures. Physiol. Plant. 15, 473–497. doi: 10.1111/j.1399-3054.1962.tb08052.x
Nakano, Y., and Asada, K. (1981). Hydrogen peroxide is scavenged by ascorbate-specific peroxidase in spinach chloroplasts. Plant Cell Physiol. 22, 867–880.
Noctor, G., and Foyer, C. H. (1998). Ascorbate and Glutathione. Keeping active oxygen under control. Ann. Rev. Plant Physiol. Mol. Biol. 49, 249–279. doi: 10.1146/annurev.arplant.49.1.249
PubMed Abstract | Full Text | CrossRef Full Text | Google Scholar
Prashanth, S. R., Sadhasivam, V., and Parida, A. (2008). Over expression of cytosolic copper/zinc superoxide dismutase from a mangrove plant Avicennia marina in indica rice var Pusa Basmati-1 confers abiotic stress tolerance. Transgenic Res. 17, 281–291. doi: 10.1007/s11248-007-9099-6
PubMed Abstract | Full Text | CrossRef Full Text | Google Scholar
Singh, N., Mishra, A., and Jha, B. (2014). Over-expression of the peroxisomal ascorbate peroxidase (SbpAPX) gene cloned from halophyte Salicornia brachiata confers salt and drought stress tolerance in transgenic tobacco. Marine Biotechnol. 16, 321–332. doi: 10.1007/s10126-013-9548-6
PubMed Abstract | Full Text | CrossRef Full Text | Google Scholar
Smith, I. K., Vierheller, T. L., and Thorne, C. A. (1988). Assay of glutathione reductase in crude tissue homogenates using 5,5′-dithiobis(2-nitrobenzoic acid). Anal. Biochem. 175, 408–413. doi: 10.1016/0003-2697(88)90564-7
PubMed Abstract | Full Text | CrossRef Full Text | Google Scholar
Srivastava, A. K., Srivastava, S., Suprasanna, P., and D'Souza, S. F. (2011). Thiourea orchestrates regulation of redox state and antioxidant responses to reduce the NaCl-induced oxidative damage in Indian mustard (Brassica juncea (L.) Czern.). Plant Physiol. Biochem. 49, 676–686. doi: 10.1016/j.plaphy.2011.02.016
PubMed Abstract | Full Text | CrossRef Full Text | Google Scholar
Srivastava, A. K., and Suprasanna, P. (in press). “Redox regulated mechanisms: implications for enhancing plant stress tolerance and crop yield,” in Elucidation of Abiotic Stress Signaling in Plants, ed G. K. Pandey (Springer). Available online at: http://www.springer.com/life+sciences/plant+sciences/book/978-1-4939-2210-9
Srivastava, S., Mishra, S., Tripathi, R. D., Dwivedi, S., and Gupta, D. K. (2006). Copper-induced oxidative stress and responses of antioxidants and phytochelatins in Hydrilla verticillata (L.f.) Royle. Aquat. Toxicol. 80, 405–415. doi: 10.1016/j.aquatox.2006.10.006
PubMed Abstract | Full Text | CrossRef Full Text | Google Scholar
Stepien, P., and Johnson, G. N. (2009). Contrasting responses of photosynthesis to salt stress in the glycophyte Arabidopsis and the Halophyte Thellungiella: role of the plastid terminal oxidase as an alternative electron sink. Plant Physiol. 149, 1154–1165. doi: 10.1104/pp.108.132407
Taamalli, M., Ghabriche, R., Amari, T., Mnasri, M., Zolla, L., Lutts, S., et al. (2014). Comparative study of Cd tolerance and accumulation potential between Cakile maritima L. (halophyte) and Brassica juncea L. Ecol. Eng. 71, 623–627. doi: 10.1016/j.ecoleng.2014.08.013
Vera-Estrella, R., Barkla, B. J., and Pantoja, O. (2014). Comparative 2D-DIGE analysis of salinity responsive microsomal proteins from leaves of salt-sensitive Arabidopsis thaliana and salt-tolerant Thellungiella salsuginea. J. Proteomics 111, 113–127. doi: 10.1016/j.jprot.2014.05.018
PubMed Abstract | Full Text | CrossRef Full Text | Google Scholar
Yildiztugay, E., Ozfidan-Konakci, C., and Kucukoduk, M. (2014). The role of antioxidant responses on the tolerance range of extreme halophyte Salsola crassa grown under toxic salt concentrations. Ecotoxicol. Environ. Saf. 110, 21–30. doi: 10.1016/j.ecoenv.2014.08.013
PubMed Abstract | Full Text | CrossRef Full Text | Google Scholar
Yu, B., and Li, W. (2014). Comparative profiling of membrane lipids during water stress in Thellungiella salsuginea and its relative Arabidopsis thaliana. Phytochemistry 108, 77–86. doi: 10.1016/j.phytochem.2014.09.012
PubMed Abstract | Full Text | CrossRef Full Text | Google Scholar
Keywords: antioxidants, ascorbate, energetics, glutathione, redox state, reactive oxygen species
Citation: Srivastava AK, Srivastava S, Lokhande VH, D'Souza SF and Suprasanna P (2015) Salt stress reveals differential antioxidant and energetics responses in glycophyte (Brassica juncea L.) and halophyte (Sesuvium portulacastrum L.). Front. Environ. Sci. 3:19. doi: 10.3389/fenvs.2015.00019
Received: 30 December 2014; Accepted: 28 February 2015;
Published: 23 March 2015.
Edited by:
Naser A. Anjum, University of Aveiro, PortugalReviewed by:
Naser A. Anjum, University of Aveiro, PortugalCopyright © 2015 Srivastava, Srivastava, Lokhande, D'Souza and Suprasanna. This is an open-access article distributed under the terms of the Creative Commons Attribution License (CC BY). The use, distribution or reproduction in other forums is permitted, provided the original author(s) or licensor are credited and that the original publication in this journal is cited, in accordance with accepted academic practice. No use, distribution or reproduction is permitted which does not comply with these terms.
*Correspondence: Ashish K. Srivastava, Plant Stress Physiology and Biotechnology Section, Nuclear Agriculture and Biotechnology Division, Bhabha Atomic Research Centre, Trombay, Mumbai 400085, IndiaYXNoaXNoYmFyY0BnbWFpbC5jb20=
†Present Address: Sudhakar Srivastava, Institute of Environment and Sustainable Development, Banaras Hindu University, Varanasi, India;
Vinayak H. Lokhande, Department of Botany, Shri Shiv Chhatrapati College of Art, Commerce and Science, Pune, India
Disclaimer: All claims expressed in this article are solely those of the authors and do not necessarily represent those of their affiliated organizations, or those of the publisher, the editors and the reviewers. Any product that may be evaluated in this article or claim that may be made by its manufacturer is not guaranteed or endorsed by the publisher.
Research integrity at Frontiers
Learn more about the work of our research integrity team to safeguard the quality of each article we publish.