- 1Fatigue Countermeasures Laboratory, Department of Psychology, San José State University, San José, CA, United States
- 2School of Business, Menlo College, Atherton, CA, United States
- 3Fatigue Countermeasures Laboratory, Human Systems Integration Division, NASA Ames Research Center, Moffett Field, CA, United States
Introduction: Fatigue is a known issue in aviation due to long and irregular working hours. In some regions, pilots are permitted to sleep during a controlled rest period on the flight deck to manage unexpected sleepiness. We aimed to determine: (1) the relative influence of pre-flight sleep-wake history and time of day on the likelihood to take controlled rest; (2) whether neurobehavioral measures taken pre-flight are predictive of controlled rest use in-flight; and (3) the impact of controlled rest on neurobehavioral measures at top-of-descent.
Methods: Data from 120 long (>6 h flight duration), unaugmented (two-pilot) flights were analyzed (n = 31 pilots). Pilots wore actiwatches and completed sleep logs before and during trips. At pre-flight and top-of-descent, pilots completed a 5-min psychomotor vigilance task (PVT) and Karolinska Sleepiness Scale (KSS). A series of mixed-effects models with relevant covariates were conducted to assess the likelihood of controlled rest based on several predictors and the impact of controlled rest on outcome measures at top-of-descent.
Results: Pilots were more likely to take controlled rest on night flights [p = .01, η2p = .13, OR = 13.81, 95% CI: (1.99, 95.80)] and when pre-flight sleepiness ratings were higher [p = .01, η2p = .14, OR = 4.14, 95% CI: (1.48, 11.57)]. Pilots who took controlled rest had faster response speeds [1000/reaction time (ms)] [p = .03, η2p = 0.07; estimated marginal mean (EMM) = 4.19, standard error (SE) = 0.07, 95% CI: (4.08, 4.29)] than those who did not [EMM = 4.00, SE = 0.05, 95% CI: (3.86, 4.14)].
Discussion: Our results suggest that taking controlled rest may improve vigilant attention at critical phases of flight and may be a useful fatigue management tool, particularly during unaugmented night flights. Further research is needed to determine the impact of psychological and cultural influences on controlled rest and to understand how the policy is applied in practice.
1 Introduction
Under European Aviation Safety Agency (EASA) regulations, an acclimatized (biological clock aligned with the time zone), unaugmented (two-pilot) crew can operate a flight duty period with a single flight of up to 13 h depending on the start time of the duty (1). In an unaugmented crew, pilots do not have the opportunity to leave the flight deck to take scheduled in-flight rest in a designated bunk. Instead, in Europe and some other regions, a fatigue mitigation strategy called controlled rest is available to pilots who experience unanticipated sleepiness in-flight. Controlled rest refers to a pilot taking a brief nap on the flight deck within a set of clearly defined guidelines as outlined by an airline's fatigue risk management system policy (2).
Although the potential benefits of controlled rest need to be weighed with the risks, studies across a range of aviation operations and regions consistently show that napping on the flightdeck occurs both intentionally (3, 4) and unintentionally (3–7), even where controlled rest is not allowed. In a real-world incident, both pilots operating an aircraft over Hawaii fell asleep causing them to miss the destination and be uncontactable for 18 min (8). These studies and events demonstrate a role for controlled rest as a way to formalize procedures within safety guidelines and, thus, reduce the risk of uncontrolled napping on the flight deck.
A survey of pilots operating under regulations which allow controlled rest found that 52% of pilots reported having used controlled rest in the past 12 months, with some reporting use of the countermeasure 13 times (9). However, for those pilots for which controlled rest is a legal option, unintentional sleep still occurs on the flight deck (10, 11). In a recent survey of nearly 7,000 European pilots, 76% of respondents reported experiencing at least one brief, uncontrolled sleep episode during flight in the past month (10). There are also real-world incidents involving non-compliant controlled rest periods (i.e., implemented in breach of standard operating procedures) (12–14). These surveys and incidents point to a need for a greater understanding of how controlled rest is being used in practice, and the importance of education for pilots to understand the foundation of the policy.
Previously, our group has characterized a cross-sectional view of the prevalence and distribution of controlled rest in long-haul flights, regardless of crew complement (i.e., augmented or unaugmented) (15). Controlled rest was taken on nearly half (46%) of all observed flights, especially on unaugmented and nighttime flights returning to base (15). Other studies have also identified peaks in controlled rest use at night (16, 17). However, there is limited research on other individual physiological and neurobehavioral factors that are associated with the use of this countermeasure.
Early experimental studies of short naps taken on the flight deck show that, on average, 26 min of sleep can be achieved in a 40-min nap opportunity (5). Such naps are associated with improvements to objective alertness (as measured using polysomnography) and objective performance (as measured by the psychomotor vigilance task, PVT) during the final, critical stages of flight (5, 18). These studies demonstrated the efficacy of short naps in an operational context under planned intervention conditions in which pilots were assigned a rest period. Currently, the effectiveness of controlled rest taken at a pilot's discretion is unknown.
We aimed to determine: (1) individual-level predictors of the likelihood of taking controlled rest; and (2) the impact of controlled rest on self-rated sleepiness and cognitive performance at top-of-descent in unaugmented crew flying normal long-haul operations. Understanding why controlled rest is taken and whether it is effective in promoting alertness and performance at critical phases of flight will help to inform guidance on the best practices for the use of this countermeasure (19, 20).
2 Materials and methods
2.1 Participants
All pilots flying long-haul operations (flights > 6.5 h) for a European airline were invited to participate in the study. There were no exclusion criteria applied within this population. All participants provided informed consent. The study was approved by the Institutional Review Board at NASA Ames Research Center (Protocol HRI-346).
2.2 Protocol
Each participant collected data over an approximately 14-day period of normal scheduling between May and October. Participants used a sleep log in a custom-built application (NASA PVT + app) on an iPod touch (6th gen, 10.3.3, Apple Inc., Cupertino, CA, USA) to record all sleep periods during the study including pre-flight, in-flight (e.g., controlled rest), layover, and post-flight rest periods. To objectively monitor sleep-wake activity, participants wore an activity monitor (Actiwatch Spectrum PRO, Philips Respironics, Murrysville, PA, USA) on their non-dominant wrist. Actigraphy was recorded in 1-minute epochs. The start and end of rest opportunities were defined by data provided by the pilots in the sleep log and applied according to internal standard operating procedures for actigraphy scoring. Actiware software (v6.0.9, Philips Respironics, Murrysville, PA, USA) was then used to estimate sleep duration within these rest periods. The software was set to the medium threshold (wake threshold 40) with sleep onset/offset thresholds set at 10 min of immobility (21). Participants completed the Karolinska Sleepiness Scale (KSS) and a 5-minute psychomotor vigilance task (PVT) (22, 23) at pre-flight, top-of-descent in-flight, and post-flight for each flight.
Researchers provided training on equipment use and study procedures, but they did not provide additional guidance on controlled rest. Pilots had previously received training on controlled rest use through the airline directly. The airline provided flight schedule data as flown.
2.3 Data analysis
Descriptive statistics were calculated for sleep and flight characteristics.
Two mixed-effects logistic models were constructed to test for the predictive utility of candidate variables in taking controlled rest. The first model (Model 1) incorporated sleep and flight variables including: (1) total sleep duration in the prior 24 h; (2) total sleep duration in the prior 48 h; (3) number of hours of continuous wakefulness; and (4) timing of the flight (night = flight touched 0200–0459, relative to homebase time) (24). Sleep variables were measured relative to flight departure time. The second model (Model 2) assessed the predictive utility of pre-flight neurobehavioral measures including: (1) KSS score; (2) PVT speed [1,000/Reaction Time (RT, ms)]; and (3) PVT lapses (RT > 500 ms). For both models, participant was entered as a random intercept effect. Due to missing data, complete data from 117 flights (n = 31 participants) were included in the first model and 89 flights (n = 29 participants) for the second model. All variables were treated as continuous except timing of flight (binary).
A series of mixed-effects models were conducted to investigate the impact of controlled rest on top-of-descent alertness and performance. Linear models were utilized for the analyses of KSS scores (Model 3a) and PVT speed (Model 3b). Due to overdispersion, we implemented a negative binomial model in the analysis of PVT lapses (Model 3c). Based on the results of our initial models examining predictors of taking controlled rest (Model 1 and Model 2), we included sleep duration in the prior 48 h and timing of the flight as covariates in these models. In addition, we included pre-flight scores for each measure as covariates, given their potential influence on the measures at top-of-descent. Due to missing data, 83 flights (n = 29 participants) were evaluated in the analyses of the KSS, while data from 76 flights (n = 28 participants) were utilized in the models examining the PVT metrics.
A series of similar mixed-effects models were performed to evaluate the effect of sleep amount during successful controlled rest attempts on each outcome measure at top-of-descent. Sleep amount is defined as the number of minutes spent asleep within the sleep period, as estimated by actigraphy. Sleep period duration (total time from the start of the sleep period to the end of the sleep period) was also investigated but did not differ from sleep amount, so the results of this analysis are not presented here. Successful controlled rest attempts were defined as attempts in which actigraphy estimated at least 1 min of sleep. Identical covariates were utilized for these analyses. For the model examining KSS (Model 4a), complete data from 48 flights in which controlled rest was successfully attempted were considered (n = 25 participants). For the models analyzing the PVT measures (Models 4b and 4c), complete data from 43 flights were utilized (n = 24 participants).
For each regression model, we computed both marginal and conditional R2 to indicate the variance explained by the fixed effects alone and the combined impact of the fixed and random effects, respectively (25). For each fixed effect, we also calculated partial eta squared (η2p) as a measure of effect size. Partial eta squared can be interpreted as follows: 0.01–0.06 = small; 0.06–0.14 = medium; >0.14 = large (26). Collinearity was assessed using the Variance Inflation Factor (VIF) for each independent variable.
3 Results
Participant demographics are displayed in Table 1 (n = 31 pilots). Data from 120 long-haul (> 6.5 h flight duration), non-augmented (two flight crewmembers) flights were analyzed.
The average flight duration was 8.32 h (SD = 0.79 h; Range = 6.75–10.43 h). Fifty-five percent of flights (n = 66) were classified as night flights (flight touched 0200–0459 relative to homebase time) (24). Controlled rest was taken on 70.0% of flights (n = 84). Controlled rest was successful (i.e., obtained sleep) on 63.3% of flights (n = 76). On 20.1% of flights (n = 25), controlled rest was taken twice within a single flight. The average total sleep amount obtained during controlled rest attempts (i.e., combining sleep amount in cases where two controlled rest periods occurred in a single flight) was 36.12 min (SD = 22.29 min; Range = 0–94 min). Table 2 displays the sleep metrics for individual controlled rest periods. Rest periods were 44.04 min on average (SD = 11.98 min), with 21.1% (n = 23) extending longer than the median and mode duration of 45 min. On average, pilots were awake for 11.06 h (SD = 3.66 h, Range: 6.53–20.18 h) when they attempted their first controlled rest period of a flight. The minimum duration of time between the end of a controlled rest period and the in-flight test was 2 h.
3.1 Predictors of taking controlled rest
Results from the mixed-effects logistic models showed significantly higher odds of taking controlled rest during flights that occurred at night [p = .01, η2p = .13, OR = 13.81, 95% CI: (1.99, 95.80); Model 1] and when participants reported higher pre-flight KSS scores [p = .01, η2p = .14, OR = 4.14, 95% CI: (1.48, 11.57); Model 2] with moderate to large effects. There were also marginal but moderate effects of sleep duration in the prior 48 h and PVT lapses, in which participants with higher such sleep durations [p = .052, η2p = .07, OR = 0.65, 95% CI: (0.42, 1.00); Model 1] and more PVT lapses [p = .051, η2p = .10, OR = 0.43, 95% CI: (0.18, 1.00); Model 2] were less likely to take controlled rest. Table 3 presents the results of these models.
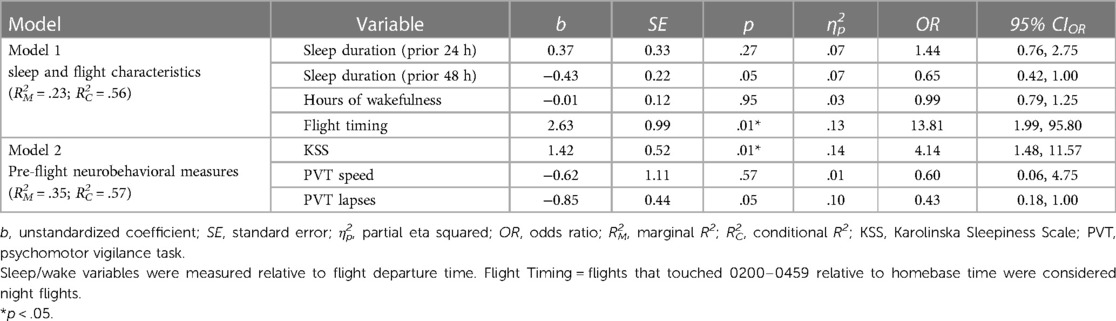
Table 3. Mixed-effects logistic model results for models 1 & 2: predictors of taking controlled rest.
3.2 Impact of taking controlled rest on neurobehavioral outcomes at top-of-descent
Overall, PVT response speed was significantly and moderately impacted by controlled rest such that pilots who took controlled rest had faster response speed at top-of-descent [p = .03, η2p = 0.07; estimated marginal mean (EMM) = 4.19, standard error (SE) = 0.07, 95% CI: (4.08, 4.29)] than those who did not [EMM = 4.00, SE = 0.05, 95% CI: (3.86, 4.14); Model 3b]. There were no differences in controlled rest for KSS scores and PVT lapses (p-values > .05; Models 3a and 3c). Table 4 provides a summary of these models. Figure 1 provides a visual representation of these results.
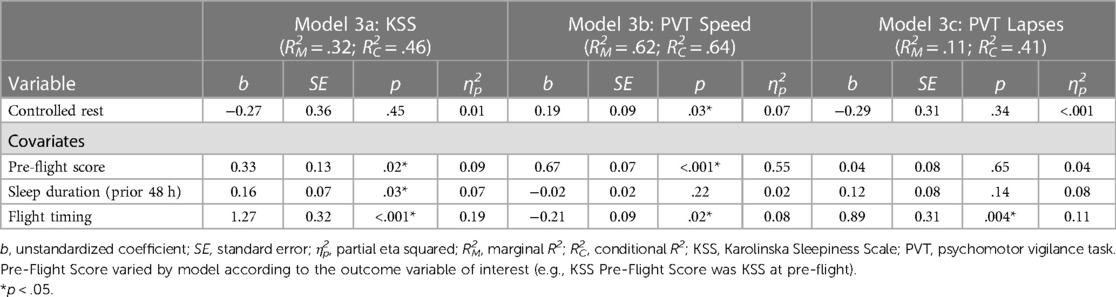
Table 4. Mixed-effects results for models 3a-3c: the impact of controlled rest on neurobehavioral outcomes at top-of-descent, adjusted by pre-flight score, sleep duration in the prior 48 h, and timing of the flight.
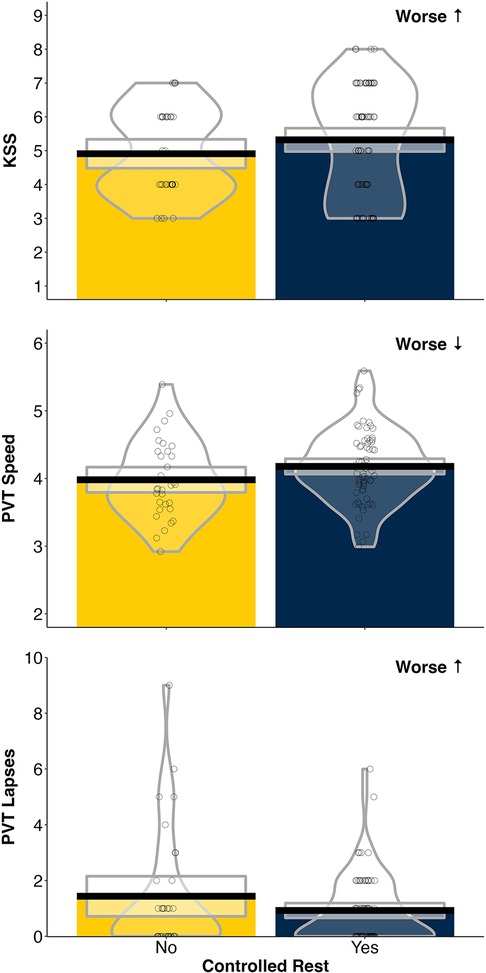
Figure 1. Pirate plots depicting Karolinska Sleepiness Scale (KSS) scores, psychomotor vigilance task (PVT) speed, and PVT lapses at top-of-descent on flights with (Yes = blue) or without (No = yellow) controlled rest. Bold line indicates the mean, box hinges represent the 95% confidence interval, individual data points displayed and visualized with density curve.
3.3 Impact of sleep amount during controlled rest on neurobehavioral outcomes at top-of-descent
The mixed-effects models revealed no significant effect of sleep amount during controlled rest on any of the outcome measures (p-values > .05; Models 4a−4c). Table 5 summarizes these results.
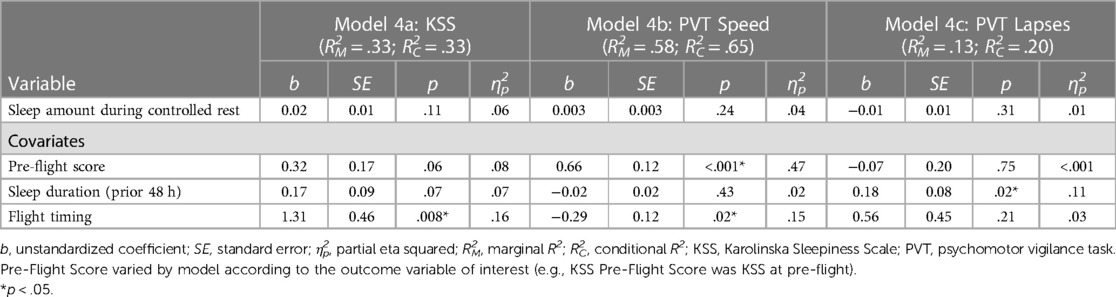
Table 5. Mixed-effects results for models 4a-4c: the effect of sleep amount during successful controlled rest periods on neurobehavioral outcomes at top-of-descent, adjusted by pre-flight score, sleep duration in the prior 48 h, and timing of the flight.
4 Discussion
During this study, we assessed: (1) factors contributing to the likelihood to take controlled rest; and (2) the impact of controlled rest taken at the pilot's discretion on sleepiness and vigilant attention at top-of-descent during normally scheduled flights. Our results show that flying during the night was the greatest predictor of taking controlled rest. Subjective sleepiness prior to a flight was predictive of the decision to take controlled rest later in the flight. However, pre-flight PVT performance was not significantly associated with the decision to take controlled rest. Taking controlled rest moderately improved PVT response speed at top-of-descent, but there were no differences in KSS or PVT lapses. The average sleep amount during successful controlled rest attempts was 31 min, but sleep amount was not significantly related to outcomes at top-of-descent. These results suggest that taking controlled rest can modestly improve vigilant attention at critical phases of flight and may be a useful fatigue management tool, particularly during unaugmented night flights.
Night flights were the strongest predictor of taking controlled rest with pilots nearly 14 times more likely to take controlled rest on these flights compared to “day” flights. Although this is only a crude proxy for circadian phase, this time likely coincides with the circadian low of pilots, when alertness is most difficult to maintain. This finding reflects our previous analysis of long-haul flights which included augmented flights (15) and supports prior studies that show an increased use of controlled rest on night flights (16, 17). However, the strength of the relationship we observed should be interpreted with caution given the large confidence interval. More accurate measures of circadian phase are needed to confirm these observations, especially in this cohort of pilots operating trans-meridian flights, which makes circadian phase hard to predict from homebase clock time (27).
From an individual neurobehavioral perspective, self-rated sleepiness pre-flight was more predictive of taking controlled rest than objective measures of vigilant attention. For every 1-unit increase on the KSS (a 9-point scale), pilots were approximately four times more likely to take controlled rest. Our results suggest that pilots may be using self-assessment of alertness states to determine whether to take controlled rest. The number of lapses on the PVT taken pre-flight trended towards significance with a modest effect size; however, we observed an unexpected negative relationship in which fewer lapses were associated with increased odds for taking controlled rest. Others have demonstrated the potential predictive ability of PVT lapse count under well-rested conditions as a metric to determine vulnerability vs. resilience across periods of sleep deprivation (28). An important difference to note is that our pre-flight measures do not necessarily represent baseline (i.e., well-rested) performance, and there are many other confounding variables such as caffeine use. This highlights, though, the challenges in applying such predictive values in operational environments in which hours of wakefulness and circadian misalignment are among a number of factors at play. Rather, it appears that self-assessment of sleepiness may be more useful in predicting the need for controlled rest in these complex operational environments.
We observed significant improvements to vigilant attention at top-of-descent on flights in which pilots chose to take controlled rest. This relationship was significant even when accounting for the influence of time of the flight (night vs. day) and pre-flight performance. Interestingly, sleepiness ratings at top-of-descent were not lower for those taking controlled rest. These results mirror a previous in-flight nap intervention study which also showed significant improvements to objective (as measured by electroencephalography and PVT) but not subjective (as measured by a visual analog scale) alertness at top-of-descent (5). It may be that sleepiness is higher overall on flights which contain controlled rest, as evidenced by higher ratings pre-flight. Conversely, pilots who were not sleepy enough to need this countermeasure may have overall lower sleepiness ratings throughout the flight. As Rosekind et al. explain (5), the amount of sleep obtained during an in-flight nap is not designed to be enough to overcome the cumulative sleep loss associated with international operations. Instead, the goal is for short-term acute relief for improving alertness at critical phases of flight relative to no nap.
The amount of sleep obtained during successful controlled rest attempts was not significantly related to neurobehavioral outcomes at top-of-descent. Pilots in our study obtained an average 40 min of sleep per flight and 31 min per controlled rest period, as estimated by actigraphy. Accounting for overestimation by actigraphy, and particularly self-report (21), this finding is on par with other studies that used different sleep measurements [46 min by self-report (17); 26 min by polysomnography (5)]. Specifically, Rosekind's et al.'s (5) study showed that amount of sleep was sufficient to improve PVT performance and eliminate microsleeps after top-of-descent. Napping studies both in the field and the laboratory consistently demonstrate improvements in alertness and performance following short naps (29, 30). Even a 10-min nap can stabilize or improve short-term performance under certain conditions (31–33). It may be that the distribution of nap lengths in our study did not allow for meaningful assessment of the impact of sleep amount. Most controlled rest policies limit the rest period to a maximum of 45 min. Nearly 80% of the controlled rest periods were within this threshold, and those exceeding this often did by only a few minutes, demonstrating that the majority of pilots were following the standard operating procedures for this fatigue countermeasure (19).
Ninety percent of controlled rest attempts were successful at achieving sleep (as estimated by actigraphy). Despite our study involving self-chosen—compared to prescribed—rest opportunities, this success rate is comparable to that reported by Rosekind et al. (5). In both these studies, however, it is important to note that even within-subjects (i.e., the same pilot), controlled rest attempts were not always successful. In a survey of over 1,000 long-haul pilots, two-thirds said they could never or seldom obtain sleep in a cockpit chair (34). Despite the high success rate observed in both naturalistic and experimental conditions, it is still important to note that sleep is not guaranteed in a controlled rest attempt. Further, it is unclear from existing data whether the decision not to take controlled rest on certain flights was operational (e.g., weather or other factors prevented use of controlled rest per policies and safety guidelines) or individual (e.g., pilot did not feel tired, or personally prefers not to take controlled rest). Together these points highlight the need for adequate fatigue management measures beyond controlled rest (e.g., flight time limitations, augmentation) and the role of controlled rest as one of many tools to manage inflight sleepiness.
4.1 Limitations
Our field study of controlled rest during regularly scheduled long-haul flight operations with objective measures of sleep and performance and self-rated sleepiness at a critical phase of flight is not without limitation. Although we were able to assess the contribution of individual physiological factors by proxy (e.g., time of day), we do not have direct measures of circadian phase in this study. Furthermore, our study lacks insight into psychological (e.g., individual preferences, rest strategies) and cultural factors (both regionally and within the airline) that may influence the decision to take controlled rest. While observing volitional controlled rest on regularly scheduled operations gives this study ecological validity, we are unable to directly assess outcomes within-subject under the same flight conditions. Thus, we cannot directly assess the impact of taking controlled rest vs. not taking controlled rest in each situation. As a secondary analysis of a larger dataset, our original sample size was reduced and potentially underpowered. The observed medium effect size (η2p = 0.07) of controlled rest on our primary outcome measure (inflight PVT) suggests we had sufficient power to detect a significant difference in this comparison. Furthermore, post-hoc power analyses confirm our observations with power greater than 0.80 to detect a moderate effect size with α = 0.05. However, we may have been underpowered for other comparisons. Finally, while we deliberately excluded flights with augmented crew and bunk rest opportunity for this analysis, controlled rest can also be taken on augmented flights (15) and our results may not generalize to those conditions or other operational environments.
4.2 Conclusions and future directions
Our results suggest that taking controlled rest can modestly improve vigilant attention at critical phases of flight and may be a useful fatigue management tool, particularly during unaugmented night flights. Self-assessment of sleepiness prior to a flight was a better predictor of the decision to take controlled rest than pre-flight PVT performance. Further research is needed to understand the influence of other scheduling and operational factors, as well as individual differences in pre-flight rest strategies and qualitative factors such as attitudes and behaviors within various safety cultural contexts. Future research would benefit from evaluating sleepiness and performance at more frequent time points throughout a flight, particularly in relation to the controlled rest period (e.g., pre and post). Collection of electroencephalography during in-flight sleep, while challenging, may extend our understanding of the impact of sleep quality during these periods. Additionally, collecting data from the pilot flying while their co-pilot is taking controlled rest would help to address risk factors associated with this countermeasure.
Data availability statement
The datasets presented in this article are not readily available because the data are protected by the data sharing policy and guidelines established by NASA Ames Research Center. Requests to access the datasets should be directed toZXJpbi5lLmZseW5uLWV2YW5zQG5hc2EuZ292.
Ethics statement
The studies involving humans were approved by Institutional Review Board at NASA Ames Research Center (Protocol HRI-346). The studies were conducted in accordance with the local legislation and institutional requirements. The participants provided their written informed consent to participate in this study.
Author contributions
CH: Conceptualization, Data curation, Formal Analysis, Investigation, Methodology, Writing – original draft, Writing – review & editing. LA: Conceptualization, Data curation, Investigation, Methodology, Writing – review & editing. SP: Data curation, Formal Analysis, Visualization, Writing – original draft, Writing – review & editing. KG: Data curation, Formal Analysis, Writing – review & editing. EF-E: Conceptualization, Investigation, Methodology, Project administration, Supervision, Writing – review & editing.
Funding
The author(s) declare that financial support was received for the research, authorship, and/or publication of this article.
NASA Airspace Operations and Safety Program, System-Wide Safety Project.
Acknowledgments
The authors would like to thank the participating pilots and airline management staff for their support of this study.
Conflict of interest
The authors declare that the research was conducted in the absence of any commercial or financial relationships that could be construed as a potential conflict of interest.
Publisher's note
All claims expressed in this article are solely those of the authors and do not necessarily represent those of their affiliated organizations, or those of the publisher, the editors and the reviewers. Any product that may be evaluated in this article, or claim that may be made by its manufacturer, is not guaranteed or endorsed by the publisher.
References
1. European Union Aviation Safety Agency (EASA). FTL 2016: Flight and Duty Time Limitations and Rest Requirements. Annex to Decision 2017/007/R AMC and GM to Part-ORO. Cologne, Germany: European Aviation Safety Agency (2017).
2. International Civil Aviation Organization (ICAO). Fatigue Management Guide for Airline Operators. 2nd edn. Montreal, Canada: ICAO (2015).
3. Co EL, Gregory KB, Johnson JM, Rosekind MR. Crew factors in flight operations XI: a survey of fatigue factors in regional airline operations. Moffett Field, California; (1999). Report No.: 1999–208799.
4. Rosekind MR, Co EL, Gregory KB, Miller DL. Crew factors in flight operations XIII: a survey of fatigue factors in corporate/executive aviation operations. Moffett Field, California. (2000).
5. Rosekind MR, Graeber RC, Dinges DF, Connell LJ, Rountree MS, Spinweber CL, et al. Crew factors in flight operations IX: effects of planned cockpit rest on crew performance and alertness in long-haul operations. Moffett Field, California. (1994).
6. Marqueze EC, Nicola ACB, Diniz DH, Fischer FM. Working hours associated with unintentional sleep at work among airline pilots. Revista de Saúde Pública. (2017) 51:61. doi: 10.1590/s1518-8787.2017051006329
7. Gregory KB, Winn W, Johnson K, Rosekind MR. Pilot fatigue survey: exploring fatigue factors in air medical operations. Air Med J. (2010) 29(6):309–19. doi: 10.1016/j.amj.2010.07.002
8. National Transportation Safety Board (NTSB). Aviation incident final report. Washington, DC. (2009). Report No.: SEA08IA080.
9. Petrie KJ, Powell D, Broadbent E. Fatigue self-management strategies and reported fatigue in international pilots. Ergonomics. (2004) 47(5):461–8. doi: 10.1080/0014013031000085653
11. Safety Matters Foundation. Safety Culture Survey (Fatigue). India: Safety Matters Foundation (2022).
12. Government of India Ministry of Civil Aviation. Report on accident to Air India Express Boeing 737−800 aircraft VT-AXV on 22nd May 2010 at Mangalore. New Delhi, India. (2010).
13. Transportation Safety Board of Canada. Pitch excursion: Air Canada, Boeing 767−333, C-GHLQ, North Atlantic Ocean, 55°00'N 029°00'W, 14 January 2011. Gatineau, Canada. (2011). Report No.: A11F0012.
14. ABC7 Chicago. 2 pilots allegedly fall asleep on flight from NYC to Rome as plane traveled 38K feet above ground. (2022). Available online at: https://abc7chicago.com/pilots-fall-asleep-pilot-during-flight-ita-airways-sleeping/11915028/ (accessed December 12, 2023).
15. Hilditch CJ, Arsintescu L, Gregory KB, Flynn-Evans EE. Mitigating fatigue on the flight deck: how is controlled rest used in practice? Chronobiol Int. (2020) 37(9-10):1483–91. doi: 10.1080/07420528.2020.1803898
16. European Commission. Effectiveness of flight time limitation (FTL) final report. Brussels, Belgium. (2018). Report No.: MOVE/C2/2016-360.
17. Gander PH, Gregory KB, Graeber RC, Connell LJ. Crew factors in Flight Operations: VIII. Factors Influencing Sleep Timing and Subjective Sleep Quality in Commercial Long-Haul Flight Crews. Moffett Field, California. (1991). Report No.: Technical Memorandum 103852.
18. Valk P, Simons M. Pros and cons of strategic napping on long haul flights. AGARD AMP Symposium on Aeromedical Support Issues in Contingency Operations Published as CP-599; 29 September–1 October, 1997; Rotterdam, The Netherlands (1997).
19. Fatigue Countermeasures Working Group. Controlled Rest on the Flight Deck: A Resource for Operators. Alexandria, VA: Flight Safety Foundation (2018).
20. Rodrigues TE, Fischer FM, Helene O, Antunes E, Furlan E, Morteo E, et al. Modelling the root causes of fatigue and associated risk factors in the Brazilian regular aviation industry. Saf Sci. (2023) 157:105905. doi: 10.1016/j.ssci.2022.105905
21. Signal TL, Gale J, Gander PH. Sleep measurement in flight crew: comparing actigraphic and subjective estimates to polysomnography. Aviat Space Environ Med. (2005) 76(11):1058–63. PMID: 16313142.16313142
22. Arsintescu L, Kato KH, Cravalho PF, Feick NH, Stone LS, Flynn-Evans EE. Validation of a touchscreen psychomotor vigilance task. Accid Anal Prev. (2019) 126:173–6. doi: 10.1016/j.aap.2017.11.041
23. Arsintescu L, Mulligan JB, Flynn-Evans EE. Evaluation of a psychomotor vigilance task for touch screen devices. Hum Factors. (2017) 59(4):661–70. doi: 10.1177/0018720816688394
24. Sallinen M, van Dijk H, Aeschbach D, Maij A, Åkerstedt T. A large-scale European Union study of aircrew fatigue during long night and disruptive duties. Aerosp Med Hum Perform. (2020) 91(8):628–35. doi: 10.3357/AMHP.5561.2020
25. Nakagawa S, Schielzeth H. A general and simple method for obtaining R2 from generalized linear mixed-effects models. Methods Ecol Evol. (2013) 4(2):133–42. doi: 10.1111/j.2041-210x.2012.00261.x
26. Cohen J. Statistical Power Analysis for the Behavioral Sciences. 2nd ed. New York, NY: Lawrence Erlbaum Associates (1988).
27. Tresguerres JA, Ariznavarreta C, Granados B, Martín M, Villanúa MA, Golombek DA, et al. Circadian urinary 6-sulphatoxymelatonin, cortisol excretion and locomotor activity in airline pilots during transmeridian flights. J Pineal Res. (2001) 31(1):16–22. doi: 10.1034/j.1600-079X.2001.310103.x
28. St. Hilaire MA, Kristal BS, Rahman SA, Sullivan JP, Quackenbush J, Duffy JF, et al. Using a single daytime performance test to identify most individuals at high-risk for performance impairment during extended wake. Sci Rep. (2019) 9(1):16681. doi: 10.1038/s41598-019-52930-y
29. Ruggiero JS, Redeker NS. Effects of napping on sleepiness and sleep-related performance deficits in night-shift workers: a systematic review. Biol Res Nurs. (2014) 16(2):134–42. doi: 10.1177/1099800413476571
30. Dutheil F, Danini B, Bagheri R, Fantini ML, Pereira B, Moustafa F, et al. Effects of a short daytime nap on the cognitive performance: a systematic review and meta-analysis. Int J Environ Res Public Health. (2021) 18(19):10212. doi: 10.3390/ijerph181910212
31. Brooks A, Lack L. A brief afternoon nap following nocturnal sleep restriction: which nap duration is most recuperative? Sleep. (2006) 29(6):831–40. doi: 10.1093/sleep/29.6.831
32. Hilditch CJ, Centofanti SA, Dorrian J, Banks S. A 30-minute, but not a 10-minute nighttime nap is associated with sleep inertia. Sleep. (2016) 39(3):675–85. doi: 10.5665/sleep.5550
33. Hilditch CJ, Dorrian J, Centofanti SA, Van Dongen HP, Banks S. Sleep inertia associated with a 10-min nap before the commute home following a night shift: a laboratory simulation study. Accid Anal Prev. (2017) 99:411–5. doi: 10.1016/j.aap.2015.11.010
Keywords: aviation, controlled rest, naps, sleepiness, fatigue, cognitive performance
Citation: Hilditch CJ, Arsintescu L, Pradhan S, Gregory KB and Flynn-Evans EE (2024) Investigating the causes and consequences of controlled rest on the flight deck. Front. Environ. Health 3:1368628. doi: 10.3389/fenvh.2024.1368628
Received: 10 January 2024; Accepted: 11 March 2024;
Published: 7 May 2024.
Edited by:
Eva-Maria Elmenhorst, Institute of Aerospace Medicine, GermanyReviewed by:
Mathias Basner, University of Pennsylvania, United StatesSteven Hursh, Institutes for Behavior Resources, United States
© 2024 Hilditch, Arsintescu, Pradhan, Gregory and Flynn-Evans. This is an open-access article distributed under the terms of the Creative Commons Attribution License (CC BY). The use, distribution or reproduction in other forums is permitted, provided the original author(s) and the copyright owner(s) are credited and that the original publication in this journal is cited, in accordance with accepted academic practice. No use, distribution or reproduction is permitted which does not comply with these terms.
*Correspondence: Cassie J. Hilditch Y2Fzc2llLmouaGlsZGl0Y2hAbmFzYS5nb3Y=