- 1Analytical and Environmental Chemistry Laboratory, Department of Chemistry, University of KwaZulu-Natal, Pietermaritzburg, South Africa
- 2Discipline of Microbiology, School of Life Sciences, University of KwaZulu-Natal, Pietermariztzburg, South Africa
Conventional wastewater treatment methods are often ineffective at fully removing heavy metals, leading to environmental and health risks. These methods are also chemically intensive and costly, emphasizing the need for sustainable alternatives. This study investigated Chlorella sp. biomass as a sustainable solution for removing heavy metals (copper, lead, and zinc) from wastewater in South Africa. The dried biomass was characterized using Energy Dispersive X-ray spectroscopy, Scanning Electron Microscopy, and Fourier-Transform Infrared Spectroscopy. Batch adsorption experiments were conducted under varying conditions: biomass dosage (5–100 mg), pH (3–11), contact time (15–115 minutes), and metal concentrations (0.25–16 mg/L) at 25°C. Adsorption isotherms, kinetics, and thermodynamic properties were also evaluated. The biomass composition revealed carbon, nitrogen, oxygen, phosphorus, and sulfur, with slightly higher nitrogen and sulfur levels than reported. Functional groups such as hydroxyl, carboxyl, carbonyl, amide, and ether were identified by FTIR. Optimal conditions for heavy metal removal were pH 7, 60 minutes of contact time, 12.5 mg biomass dosage, and 0.5 mg/L metal concentration. In wastewater samples, Chlorella sp. biomass achieved 100% removal of copper and zinc and 98% removal of lead. Adsorption followed the Langmuir isotherm for copper (R2 = 0.9888) and the Freundlich isotherm for lead (R2 = 0.976) and zinc (R2 = 0.968). Kinetic studies followed a pseudo-first-order model, and thermodynamic analysis indicated an endothermic and spontaneous adsorption process for copper and zinc, with lead showing complete removal at all temperatures. Chlorella sp. biomass demonstrated high efficiency and sustainability in removing heavy metals from wastewater, offering a promising alternative to conventional methods. Future research should focus on improving removal by combining Chlorella sp. biomass with other microorganisms and scaling up to pilot applications.
1 Introduction
Recent global assessments indicate that heavy metal contamination affects drinking water sources for approximately 40% of the lakes and rivers worldwide, highlighting the pervasive nature of this environmental and public health issue (Zamora-Ledezma et al., 2021; Pandey et al., 2023; Piwowarska et al., 2024). Heavy metals such as copper (Cu), cadmium (Cd), chromium (Cr), mercury (Hg), and lead (Pb) are persistent pollutants in water bodies, originating mainly from industrial discharges, agricultural runoff, mining activities, and improper waste disposal (Briffa et al., 2020; Balali-Mood et al., 2021; Naicker et al., 2024) The toxicity of heavy metals poses substantial health risks to human populations. Acute exposures can lead to severe neurological disorders, gastrointestinal disturbances, and cardiovascular diseases (Balali-Mood et al., 2021; Spain et al., 2021). Chronic exposure, even at low levels, is linked to various cancers, kidney diseases, and reproductive disorders (Cherono et al., 2021; Prabakaran and Rajan, 2021). Vulnerable groups such as children, pregnant women, and the elderly are particularly susceptible due to their higher sensitivity to toxic substances and prolonged exposure durations (Briffa et al., 2020; Balali-Mood et al., 2021). In addition to direct health impacts, heavy metals bioaccumulate in aquatic organisms, entering the food chain and posing ecological threats. Fish and shellfish, vital protein sources for millions, can accumulate high levels of these contaminants, leading to food safety concerns and economic impacts on fisheries (Tattibayeva et al., 2022). Furthermore, heavy metal contamination jeopardizes ecosystem health, disrupting aquatic biodiversity and ecosystem services crucial for human wellbeing, such as water purification and nutrient cycling (Briffa et al., 2020; Balali-Mood et al., 2021; Tattibayeva et al., 2022; Naicker et al., 2024; Piwowarska et al., 2024).
Although conventional wastewater treatment processes (e.g., activated sludge, chemical precipitation) and advanced techniques (e.g., reverse osmosis, membrane filtration, ion exchange) can achieve significant heavy metal removal, these approaches face notable challenges (Briffa et al., 2020; Piwowarska et al., 2024). Advanced methods, in particular, are characterized by high operational costs, reliance on chemicals that generate secondary pollution, limited efficiency at low metal concentrations, and complex processes that require skilled operators (Ni et al., 2019; Pfeifer and Skerget, 2020; Cherono et al., 2021; Tattibayeva et al., 2022). As a result, heavy metals often persist in treated wastewater and subsequently contaminate receiving rivers (Naicker et al., 2024). Despite this challenge, studies focusing on heavy metal removal using alternative, cost-effective methods remain limited within regions like South Africa, where heavy metal contamination is a huge environmental problem. This limitation underscores a critical research gap, emphasizing the urgent need to develop and implement efficient, affordable, and environmentally sustainable solutions tailored to the specific environmental and socio-economic contexts of South Africa and similar regions.
In recent years, biological adsorbents, such as microalgal biomass, have emerged as promising alternatives to conventional methods due to their eco-friendly nature, low cost, and high adsorption capacity. Among various microalgae, Chlorella sp. has shown considerable potential owing to their fast growth rates, high surface area, ease of cultivation, and the presence of functional groups (e.g., amide, carboxyl, hydroxyl) on their cell walls that facilitate metal binding (Yadav et al., 2021; Al-Khiat et al., 2023; Ma and Jian, 2023). The processes that govern the sequestration of heavy metals by microalgae like Chlorella sp. include ion exchange, complexation, surface complexation, precipitation, and reduction (Al-Khiat et al., 2023). Despite this promise, significant research gaps remain in understanding the adsorption mechanisms of heavy metals by specific Chlorella strains under varying environmental conditions (e.g., pH, biomass dose, and contact time). Furthermore, previous studies have largely focused on single-metal systems, failing to reflect the complexity of real-world wastewater environments where multiple metals coexist (Pfeifer and Skerget, 2020; Tattibayeva et al., 2020; Al-Khiat et al., 2023).
Therefore, this study addressed these gaps by systematically investigating the adsorption of selected heavy metals (Cu, Pb, and Zn) using Chlorella sp. biomass. Unlike heavy metals such as Hg, Cd, and Cr, which are primarily linked to mining, metallurgical processes, and specific industries; Cu, Pb, and Zn are more commonly found in urban wastewater due to their widespread use in plumbing, metal finishing, automotive industries, aging infrastructure, and their non-biodegradable nature (Ni et al., 2019; Briffa et al., 2020; Zamora-Ledezma et al., 2021; Piwowarska et al., 2024). Furthermore, a recent study (Naicker et al., 2024) has reported elevated levels of Cu, Pb, and Zn in South African urban wastewater, further justifying their selection. The research objectives were to: 1) Characterize the functional groups and elemental composition of the biomass to understand its adsorption properties. 2) Optimize conditions for maximum heavy metal removal, including biomass dosage, pH levels, contact times, and metal concentrations. 3) Identify the best-fitting adsorption isotherm models (Freundlich, Langmuir, and Temkin) and analyze the kinetics and thermodynamics of the adsorption process. This research holds significant promise for addressing heavy metal contamination in low-resource settings, particularly in African regions where conventional methods are often inaccessible. Moreover, the findings align with Sustainable Development Goal 6 (Clean Water and Sanitation) by advancing sustainable water treatment practices that can be readily implemented in communities affected by heavy metal pollution (Pachouri et al., 2024).
2 Materials and methods
2.1 Study site, sample collection, storage, and preparation
2.1.1 Study site
The Darvill Wastewater Treatment Plant (WWTP) (29.60125°S, 30.42881°E) in Pietermaritzburg, KwaZulu-Natal, South Africa, was selected as the study site. This WWTP is a major facility designed to treat up to 100,000 m3 of wastewater daily (Umgeni. Annual Report, 2022/2023, 2023). Since its establishment in 1992, the plant has played a crucial role in managing the city’s domestic and industrial wastewater (Sikhakhane, 2001). The plant utilizes a combination of biological filtration and activated sludge process technologies, both of which are key components of secondary treatment, to effectively reduce organic matter and suspended pollutants before discharging the treated wastewater. The treated effluent from Darvill WWTP is released into the Msunduzi River, a significant tributary of the Umgeni River. The Umgeni River is a vital water resource in the region, serving as the primary drinking water source for rural communities in the Valley of a Thousand Hills and eventually feeding into Inanda Dam. This dam is part of the Umgeni-Uthukela catchment and supplies potable water to the Durban metropolitan area, supporting millions of residents and businesses.
2.1.2 Sample collection
The water samples were collected from the effluent point using high-quality plastic containers (PE, Lasec, South Africa) to prevent sample contamination and adsorption on the walls of the container. The containers were then sealed airtight to prevent the heavy metals from oxidizing and forming insoluble compounds in the presence of air (Abd-El-Nabey et al., 2022). A total of five 2-L samples were collected at the sampling point, ensuring sufficient volume for the experiments conducted in this study. Samples were temporarily stored in a cooler box with ice during transportation and kept in a fridge at about 4°C at the laboratory.
2.1.3 Measurements
Parameters such as pH, conductivity, temperature, total dissolved solids (TDS), salinity, and dissolved oxygen (DO) were measured using a portable multiparameter meter (Bante Instruments 900P, China). Before experiments, the wastewater effluent samples underwent filtration using a 55 mm CHM shift filtration paper (F1001 grade, Separations, South Africa) to eliminate large particles.
2.2 Reagents and certified reference material
Purelab ultrapure water with a conductivity of 18.2 MΩ cm was employed to prepare calibration standards, and all glassware was cleaned using a diluted nitric acid solution. The 1,000 mg/L ICP heavy metals CRMs (Copper–Cu, Lead–Pb, and Zinc–Zn) procured from Sigma Aldrich (Johannesburg, South Africa) were used in the analysis.
2.3 ICP-OES instrument calibration
The metal determination in water was conducted using the Varian 720-ES ICP-OES instrument (Varian, Johannesburg, South Africa). The instrument operated at a frequency of 40 MHz, with an RF power of 1.00 kW. A pneumatic concentric nebulizer was utilized, delivering a flow rate of 0.75 L/min, and an inert carrier gas (Argon) was introduced at a rate of 15 rpm (Naicker et al., 2023). The wavelengths acquired were 327.396 nm for copper, 220.353 nm for lead, and 202.548 nm for zinc.
2.4 Validation of the analytical method for the determination of heavy metals
The evaluation of the performance of the analytical methods involved assessments of linearity, limits of detection (LOD), limits of quantification (LOQ), and percentage recovery tests. The ICP-OES calibration and the method linearity assessment were performed using a mixture of heavy metals with concentrations ranging from 0.1 to 20 mg/L. The intensities of ten blank samples (n = 10) were measured. To determine the LODs and LOQs for the specific analytes, these values were calculated as 3 and ten times the standard deviation (σ) of the average of the ten individually prepared blank solutions. The method’s accuracy was evaluated by spiking wastewater effluent samples with 1 mg/L standard containing a mixture of heavy metals of interest. Subsequently, the percentage recoveries were computed using Equation 1:
2.5 Chlorella sp. culture and maintenance
2.5.1 Cultivation and growth monitoring
The uMgeni-uThukela Water (Pietermaritzburg, South Africa) provided the Chlorella sp. strain (FSB41.2) used in this study, with cultivation routinely done using tris-acetate phosphate (TAP) medium, as outlined by Harris (Harris, 1989). An axenic culture was established by sequential subculturing and purification of single colonies of Chlorella sp. using an acetate-free medium under autotrophic growth conditions with regular culture-based (Nutrient agar) and microscopic (phase contrast) monitoring as reported previously (Adedoyin and Schmidt, 2023). Subsequently, biomass production occurred in a controlled growth environment (approximately 23°C with a 20-hour light-dark cycle) using 1,000 mL Erlenmeyer flasks, each filled with 600 mL of the TP culture medium following autoclaving. The growth of cultures was monitored over time by absorbance measurements at 680 nm using a UV/Visible Spectrophotometer (JENWAY 7310, United Kingdom).
2.5.2 Biomass harvesting and processing
The Chlorella sp. biomass was extracted from the TP culture medium after reaching the late exponential growth phase by centrifugation (3354 g for 15 min) and washed 3 times with distilled water to remove the remaining medium. Following centrifugation, the resulting Chlorella sp. cell pellets were subjected to overnight oven drying at 80°C, since very low or exceedingly high temperatures negatively affect the cell structure (Tattibayeva et al., 2022). Subsequently, an electric grinder (MRC SM-450, Material Research Company, Israel) was used to grind the dried pellet, followed by sieving (KINGTEST sieve shaker VB200/300, Kingtest, China) and storing the dried biomass in a sealed container to prevent rehydration.
2.6 Characterisation of Chlorella sp. biomass
The dried Chlorella sp. biomass was characterized by analyzing its elemental composition, surface properties, and functional groups using Fourier-Transform Infrared Spectroscopy (PerkinElmer Inc., United States), and Scanning Electron Microscopy (SEM Zeiss EVO LS15, Germany) equipped with Energy Dispersive X-ray (EDX) detector. This study also investigated the zero-point charge (pHZPC) of Chlorella sp. biomass. A total of 12.5 mg of biomass was added to Erlenmeyer flasks, each containing 30 mL of 0.1 M sodium nitrate (NaNO3) solution. The pH in each flask was adjusted to values between 2 and 12 using 0.1 M hydrochloric acid (HCl) and 0.1 M sodium hydroxide (NaOH). The flasks were then placed on an orbital shaker for 24 h. Afterward, the pH was measured again, and the change in pH (∆pH) was calculated as the difference between the initial and final pH values: ∆pH = pHi − pHf. Then, the optimization for the adsorption studies was performed by adjusting parameters such as biomass dosage, pH, contact time, and initial heavy metal ion concentration.
2.7 Adsorption studies
2.7.1 Optimization of adsorption conditions
Dried Chlorella sp. biomass (12.5 ± 0.1 mg) obtained from axenic cultures (see Section 2.5 for biomass preparation details) was added to conical flasks containing 30 mL of deionized water. The water was spiked with a mixture of Cu, Pb, and Zn ions, each applied at the same initial concentration within the range of 0.25–16 mg/L. The initial experiments were conducted to optimize adsorption conditions, including biomass dosage, metal ion concentration, pH, and contact time.
2.7.2 Application to real wastewater
The optimal conditions were then applied to real wastewater to assess adsorption performance in a realistic scenario and evaluate the removal efficiency of contaminants in actual water systems. Adsorption behavior was evaluated using Freundlich, Langmuir, and Temkin isotherms, while kinetic studies were assessed using intraparticle diffusion models, pseudo-first-order, and pseudo-second-order kinetics. For isotherm and kinetic experiments, the samples were incubated in a rotary shaker at 150 rpm and 25°C for 60 min. The initial concentrations of heavy metals and the contact time of 60 min were chosen based on the optimization results, which identified conditions that achieved optimal adsorption performance. The temperature and shaker speed were maintained constant throughout the study, as these parameters are widely supported in the literature for ensuring consistency and reliability in adsorption experiments (Li et al., 2020; Pfeifer and Skerget, 2020; Cherono et al., 2021; Tattibayeva et al., 2022; Wang and Guo, 2023). The samples were centrifuged at 3354 g for 15 min to separate the Chlorella cells from the liquid. The liquid samples were then filtered with a 0.22 μm pore size filter (Merck Mille, Germany) and a 30 mm sterile syringe to remove residual algal cells before analysis with ICP OES. The impact of temperature on adsorption equilibrium was explored by altering the temperature within the 298–333 K range (Tang et al., 2017; Shi et al., 2023; Soto-Ramírez et al., 2023).
2.7.3 Adsorption performance calculations
The adsorption process was quantified using Equation 2:
Where qe is the adsorption capacity (mg/g), C0 is the initial concentration of the adsorbate (mg/L), Ce is the equilibrium concentration of the adsorbate (mg/L), V is the volume of the solution (L), m is the mass of the adsorbent (g).
The degree of removal (R) of heavy metal ions was calculated by using Equation 3:
Where: RE is the removal efficiency in percentage, C0 is the initial concentration before adsorption, and Ce is the concentration at equilibrium.
2.7.3.1 Kinetic studies
The kinetic models of pseudo-first order, pseudo-second order, and intraparticle diffusion were calculated using Equations 4-6 (Shi et al., 2023; Spain et al., 2021).
Where: qt: Adsorption capacity at time t (mg/g), qe: Adsorption capacity at equilibrium (mg/g), t: Time (min), k1: Rate constant of the pseudo-first-order kinetics (1/min).
Where: qt: Adsorption capacity at time t (mg/g), qe: Adsorption capacity at equilibrium (mg/g), t: Time (min), k2: Rate constant of the pseudo-second-order kinetics (1/min).
Where: qt: Adsorption capacity at time t (mg/g), k: intraparticle diffusion rate constant, t: Time (min), C: is the intercept that represents the boundary layer effect.
2.7.3.2 Isotherm studies
Equations 7-9 were used to calculate Freundlich, Langmuir, and Temkin isotherms:
Where log (qe) is the logarithm of the amount of solute adsorbed per unit mass of adsorbent at equilibrium, log(Kf): is the logarithm of the Freundlich constant, 1/n is the reciprocal of the Freundlich exponent or non-linearity factor, log(Ce): is the logarithm of the equilibrium concentration of the solute in the liquid phase.
Where qe (mg/g) represents the amount of adsorbate adsorbed at equilibrium, qmax (mg/g) is the maximum adsorption capacity, KL (L/mg) is the Langmuir adsorption constant, and Ce (mg/L) is the equilibrium concentration of the adsorbate.
Where qe is the amount of adsorbate adsorbed at equilibrium (mg/g), R is the gas constant (8.314 J/mol⋅K), T is the temperature (K), b is the Temkin constant related to the heat of adsorption (J/mol), A is the Temkin isotherm constant, and Ce is the equilibrium concentration of the adsorbate (mg/L).
2.7.3.3 Thermodynamic studies
Thermodynamic parameters were calculated using Equations 10-13:
Where: ΔG is the Gibbs free energy change (kJ/mol), ΔH is the enthalpy change (kJ/mol), ΔS is the entropy change (kJ/mol), T is the absolute temperature (K), R = Gas constant.
2.8 Re-usability
Reusability of adsorbents is key for practical and sustainable adsorption processes; reducing new material demand, enabling recovery of adsorbed substances, and supporting cost-efficiency as well as environmental sustainability (Simelane et al., 2024). In this study, desorption was achieved by treating dried Chlorella sp. biomass with 0.05 M Ethylenediaminetetraacetic acid (EDTA) and 0.05 M HCl. EDTA and HCl were chosen as desorption agents due to their complementary strengths. For example, HCl protonates binding sites to release metals like Cu, while EDTA, a chelating agent, effectively desorbs metals like Pb and Zn by forming stable complexes (Zhang et al., 2021a; Chatterjee and Abraham, 2019). A 0.05 M concentration has been reported to be optimal, as it provides effective desorption without risking damage to the biomass, allowing for repeated adsorption-desorption cycles in a safe and sustainable way (Kordialik-Bogacka, 2011; Kumar et al., 2018). In this study, reusability tests were conducted in a manner similar to the adsorption experiments (see Section 2.7). After adsorption, the Chlorella pellets were separated from the supernatant and rinsed three times with ultrapure water. The concentrations of Pb, Cu, and Zn in the supernatant were measured using ICP-OES. The Chlorella pellets were further treated with 50 mL of the eluent agent (either EDTA or HCl) separately and shaken using an orbital shaker at 150 rpm at 25°C for 120 min. After completing desorption, samples were collected and dried in a fume hood to prepare them for the next adsorption-desorption cycle.
2.9 Statistical analysis
Statistical analyses were conducted in Python (version 3.13.1) using Jupyter Notebook (version 7.3.2). A one-way analysis of variance (ANOVA) was performed separately for each factor (pH, contact time, initial concentration, and biomass dosage) to assess their individual effects on removal efficiency. When ANOVA indicated significant differences (p < 0.05), Tukey’s honestly significant difference (HSD) test was applied for pairwise comparisons between factor levels.
3 Results and discussion
3.1 Method validation for the ICP-OES instrument
The performance of the ICP-OES was assessed through regression equations from concentrations ranging from 0.1 to 20 mg/L. The correlation coefficients (R2) ranged from 0.9996 to 0.9998, signifying a strong linear relationship between the concentrations assessed and the ICP-OES, indicating exceptional accuracy and precision (Table 1).
When using ICP-OES, selecting optimum wavelengths is paramount for producing accurate and sensitive results as they maximize sensitivity, decrease spectral interferences, and increase measurement precision (Naicker et al., 2023). The LOD and LOQ obtained in this study ranged from 0.06 to 0.255 mg/L and 0.196–0.851 mg/L respectively. This implied a high sensitivity level, affirming the methods’ capability to detect and quantify trace levels of the three analyzed metals effectively. This study assessed precision by repeatability and reproducibility, expressed as %RSD. The results produced %RSD values of less than 1% for all analytes, indicating high precision and reliable performance.
3.2 Characterisation of Chlorella sp. biomass as the adsorbent
3.2.1 Surface morphology
Scanning electron microscopy (SEM) is a powerful tool for visualizing the surface features and morphology of microorganisms such as Chlorella sp. (Nasir et al., 2023). As expected, the cells of Chlorella sp. used even in the dried state, showed an almost intact surface area, enabling the binding of metal ions via interaction with exposed functional groups (Figure 1a) (Spain et al., 2021; Tattibayeva et al., 2022). An image taken after adsorption indicated morphological changes resembling cell shrinkage and increased surface irregularities, suggesting an interaction of the cell surface with metal ions (Figure 1b).
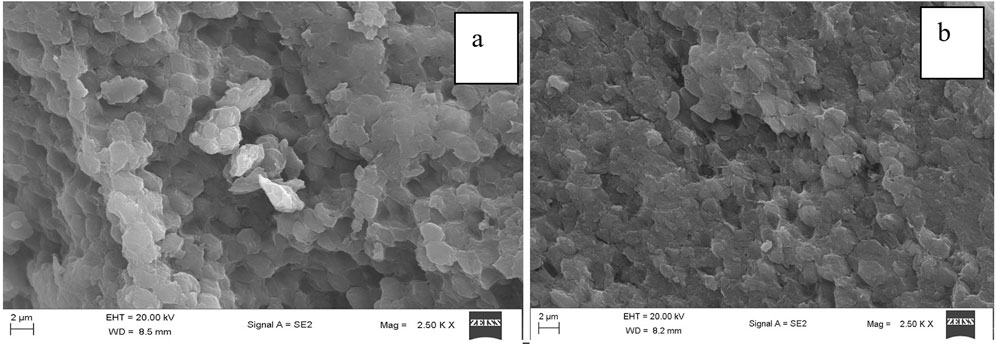
Figure 1. Scanning electron micrograph of dried Chlorella sp. cells before (a) and after (b) metal binding.
3.2.2 Elemental composition and functional groups in Chlorella sp. biomass
The results of the analysis of the elemental composition of Chlorella sp. biomass, which comprises carbon (C), nitrogen (N), oxygen (O), phosphorus (P), and sulfur (S) are shown in Figure 2a. The obtained elemental composition in this study closely aligns with the literature values reported for Chlorella sp. by Mandalam and Palsson, with the exception of slightly higher levels of nitrogen (N) and sulfur (S) in this study (Mandalam and Palsson, 1998). The detected elements are associated with microalgal biomass, such as Chlorella sp., and are key elements present in microalgal proteins, nucleic acids, lipids, carbohydrates, and organic acids (Ma et al., 2020; Yaakob et al., 2021). Organic functional groups, such as carboxyl (-COO) and hydroxyl (-OH), are abundant on the cell surface of Chlorella sp. and are crucial in the adsorption of heavy metals by providing binding sites (Yang et al., 2019). Similarly, nitrogen-containing functional groups such as amide (C-N) associated with cell surface proteins form various interactions with metal ions (Giarikos et al., 2021), which applies as well to phosphate and sulfur-containing groups.
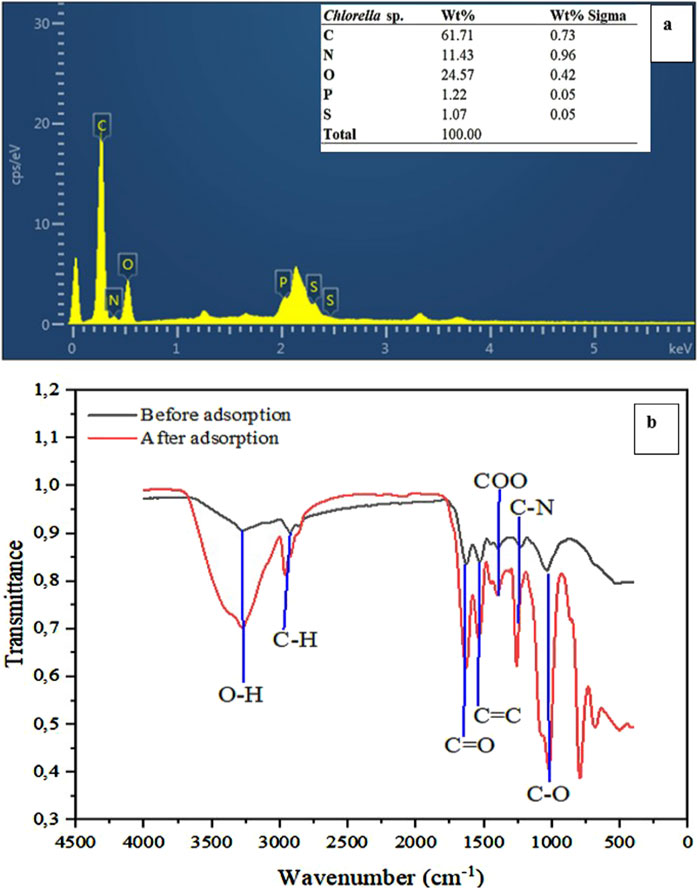
Figure 2. Energy Dispersive X-ray Spectroscopy before adsorption (a) and Fourier transform infrared spectroscopy (b) of Chlorella sp. biomass.
Analytical methods such as Fourier-transform infrared spectroscopy (FTIR) are vital in identifying functional groups present in microbial biomass. For Chlorella sp., the functional groups present in biomass samples analysed included hydroxyl (-OH), methyl (-CH3), carbonyl (C=O), alkene (C=C), carboxyl (-COO), amide (C-N), and ether (C-O) (Figure 2b). These functional groups increase the overall binding capacity and reactivity of Chlorella biomass. This study results agree with the findings of Ahmad et al. (2019), El-Naggar et al. (2020), Sultana et al. (2020), Shi et al. (2023), who reported the occurrence of similar functional groups that play a significant role in heavy metal adsorption. Notably, a shift from 3,270 to 3,281 cm-1 after adsorption was observed in the peak associated with the hydroxyl group, which enables hydrogen bonding interactions (Wohlert et al., 2022; Wang and Guo, 2023). This observed change in peak intensity and broadness confirmed the interactions of hydroxyl groups with metal ions. The observed shift in C-H stretching vibrations from 2,925 to 2,968 cm-1 provided evidence of their involvement in hydrophobic interactions (Barchi and Strain, 2023). The increased intensity, as well as shifts in C=O, C=C, and C-N stretching vibrations from 1,618 to 1,638 cm-1, 1,538 to 1,528 cm-1, and 1,234 to 1,250 cm-1, respectively, suggests the interaction of carbonyl, alkene, and amide groups with metal ions during the adsorption process through metal coordination (Spain et al., 2021; Li H. et al., 2022; Barchi and Strain, 2023). Other noticeable shifts in the carboxyl group (1,407–1,398 cm-1) and ether group (1,036–1,028 cm-1) were observed. The carboxyl groups promote electrostatic interactions through negatively charged sites, and ether groups play a crucial role in the overall surface polarity (Barczak, 2019; Zhang et al., 2019). These results demonstrated a diversity of crucial functional groups present on the dried Chlorella sp. biomass, enabling the complexity of interactions and ultimately impacting the adsorption capacity of the Chlorella biomass for target heavy metals.
3.3 Zero-point charge (pHZPC) of Chlorella sp. biomass as the adsorbent
When using Chlorella sp., NaNO3 is preferred over HCl for several scientific reasons. First, NaNO3 provides a stable and neutral ionic environment that does not alter the pH significantly, allowing for the precise determination of the point at which the surface charge of the biomass is neutral (Sočo et al., 2024). HCl, being a strong acid, can dramatically lower the pH, leading to the protonation of functional groups on the biomass surface (Li N. et al., 2022).
The pHZPC represents the pH at which the surface of the biomass has no net charge (Bakatula et al., 2018). At this point, the number of protonated and deprotonated sites is balanced, resulting in minimal adsorption of either cations or anions. For Chlorella sp. biomass, experimental data pinpoint the pHZPC to be near 6, as indicated by a negligible change in pH (ΔpH) (Figure 3). In pH below 5.8, the surface of the biomass is predominantly protonated due to the presence of functional groups such as carboxyl (-COO) and amino (-NH2) groups, which gain protons and become positively charged. This positive surface charge repels metal cations, thereby reducing the biomass’s affinity for adsorbing heavy metals at low pH. Conversely, as the solution pH increases above the pHZPC, these functional groups undergo deprotonation, imparting a net negative charge to the surface. This negative charge facilitates electrostatic attraction between the biomass and positively charged metal ions, thereby enhancing adsorption efficiency (Li N. et al., 2022; Raji et al., 2023). The zero-pH change observed at an initial pH of 2 can be attributed to the extreme acidic environment, where the solution is dominated by a high concentration of free H+ ions. At this pH, functional groups on the biomass are likely saturated with protons, leaving limited capacity for further interaction or buffering against the surrounding H+ (Charazińska et al., 2021). This proton saturation means the biomass cannot effectively neutralize or alter the solution’s pH, resulting in a negligible ΔpH. In some cases, strongly acidic conditions can also alter the structural properties of biomass, potentially reducing its ion-exchange efficiency.
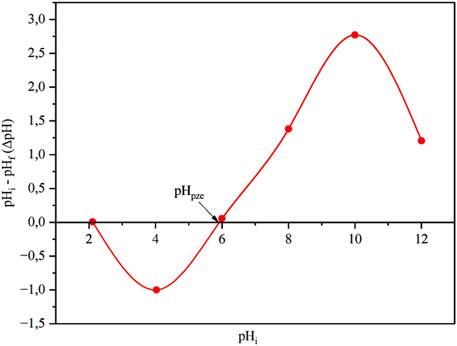
Figure 3. Point of zero charge for Chlorella sp. biomass in 0.1 M sodium nitrate (n = 3 replicates).
3.4 Optimisation of the adsorption of metals to the biomass of Chlorella sp
3.4.1 Effects of the biomass dosage on the degree of removal efficiency
Finding optimum conditions for critical parameters such as biomass dosage is crucial in Chlorella-biomass-based adsorption studies as it ensures cost-effectiveness and enhances the efficiency and environmental sustainability of the process. The results depicting the effect of biomass dosage are illustrated in Figure 4a. The ANOVA results (p < 0.05) showed that biomass dosage significantly affects metal removal efficiencies (Supplementary Table S5).
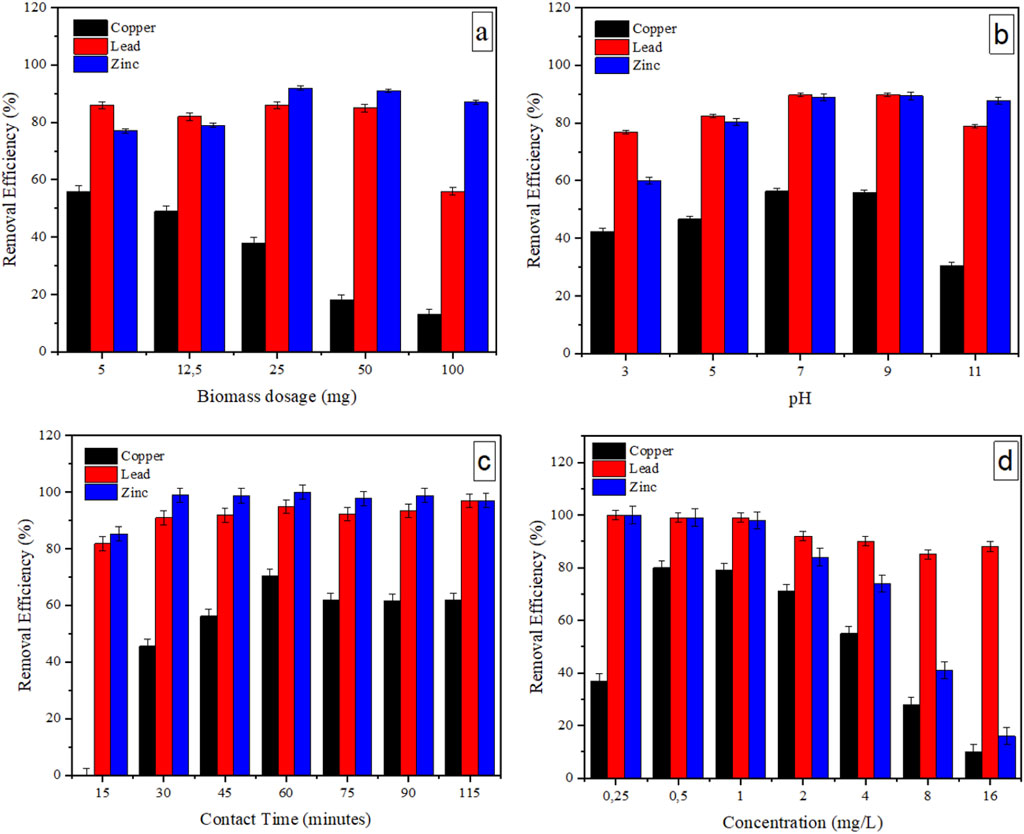
Figure 4. The effect of biomass dosage (a), pH (b), contact time (c), and metal concentration (d) on the metal removal efficiency of Chlorella sp. biomass with n = 3 replicates. Error bars represent the standard deviations.
Copper removal efficiency decreased as the biomass dosage increased (p < 0.05), with the highest removal being 56% at 5 mg. This could be ascribed to competitive adsorption as the heavy metals were present in a mixture, with the three metals potentially competing for the same available binding sites. These findings align with the observations made by Ni et al. (2019), who documented competitive adsorption behaviour between cadmium and lead, where cadmium showed a decreased adsorption in the presence of lead. This was ascribed to lead’s higher electronegativity and binding affinity, larger hydrolysis constant, atomic weight, ionic radius, and a larger Misono softness value, increasing its binding favourability (Al Hamouz and Ali, 2013; Ni et al., 2019; Musumba et al., 2020). In this study, the Chlorella sp. biomass consistently removed lead and zinc with high removal efficiencies, with maximum values of 86% and 95% recorded at 25 mg, respectively. Tukey’s post hoc test exhibited that adsorption at 25 mg and 50 mg was not significantly different for lead (p = 0.6539) and zinc (p = 0.5255), indicating a plateau in adsorption efficiency at these dosages (Supplementary Table S5). However, the 100 mg dosage led to a significant reduction in zinc removal (p < 0.05) (Supplementary Table S8), likely due to biomass aggregation, which reduces the surface area exposed to the solution, severely impacting adsorption capacity (Pfeifer and Skerget, 2020). A biomass dosage of 12.5 mg was selected for the later experiments. This selection was justified by the nearly identical removal efficiencies observed at 12.5 mg and 25 mg for lead and zinc (above 80%), as well as notably better removal for copper.
3.4.2 Effects of pH on the degree of removal efficiency by Chlorella sp. biomass
Figure 4b illustrates the effect of a varying pH at constant biomass dosage, contact time, temperature, and metal concentration upon the metal removal efficacy. The low removal efficiencies observed at acidic conditions (pH 3-5), particularly for copper and zinc, could be attributed to the protonation of metal binding functional groups (e.g., -NH2, -OH, -SH) in Chlorella biomass, causing them to behave like positively charged species that repel metal cations (Cherono et al., 2021; Tattibayeva et al., 2022). Similarly, several studies reported that low pH promoted competition between metal ions and protons for binding sites, resulting in reduced adsorption of their analytes (Kiruba et al., 2014; Gonte and Balasubramanian, 2016; Shi et al., 2023). Additionally, Almomani and Bhosale documented that the solubility of heavy metals in water increases in acidic conditions, resulting in decreased adsorption rates (Almomani and Bhosale, 2021). The removal efficiencies for copper, lead, and zinc remained relatively stable between pH 7 and 9, with values of 56.35% and 55.75% for copper, 89.68% and 89.86% for lead, and 88.98% and 89.40% for zinc, respectively. Tukey’s post hoc test confirmed that the differences in removal efficiencies between pH 7 and 9 were not statistically significant for copper (p = 0.3207), lead (p = 0.9989), and zinc (p = 0.5604), suggesting an equilibrium point for metal binding within this pH range (Supplementary Tables S1-S4). However, when the pH becomes too basic (pH 11), a decrease in removal efficiencies was observed, particularly for copper and, to a lesser degree, for lead and copper, which can be attributed to the formation of insoluble metal hydroxides, hindering metal adsorption onto binding sites (Al-Saadi et al., 2013; Cherono et al., 2021). These findings match studies by Zhang and Depci et al. (Depci et al., 2012; Zhang, 2011) that assessed the removal of heavy metals employing dairy manure compost and activated carbon derived from apple pulp. The significant drop in adsorption at pH 11, as indicated by Tukey’s post hoc test (p < 0.001), supports that extreme pH conditions negatively impact metal removal efficiency. Thus, pH 7 was chosen as the optimum condition as it helps to prevent the disruption of pH-sensitive biological processes and the accumulation of toxic substances in receiving water bodies, contributing to the overall health and sustainability of the environment.
3.4.3 Effects of time on the degree of removal efficiency by Chlorella biomass
The effect of contact time on removing selected heavy metals was investigated (Figure 4c). An increase in lead and zinc removal efficiencies from 15 to 30 min was observed, attributable to lead and zinc having a larger ionic radius than copper, resulting in stronger interactions with binding sites on the Chlorella sp. cell surface, leading to higher and faster adsorption than small copper ions (Ni et al., 2019). Tukey’s post hoc test confirmed significant differences between 15 and 30 min for lead (p < 0.05) and zinc (p < 0.05), reinforcing the impact of contact time on metal removal efficiency (Supplementary Tables S11, 12). As the contact time increased (45–115 min), the removal of these 2 compounds did not increase substantially, indicating saturated binding sites and the establishment of an equilibrium between metal ions and the binding sites available on the surface of Chlorella biomass. Tukey’s post hoc further confirmed this trend as the results for lead showed that p-values between 45 and 75 min (0.9996), 45 and 90 min (0.0616), and 75 and 90 min (0.1226) were not statistically different (Supplementary Table S11). Similarly, for zinc, the p-values between 45 and 75 min (0.6205), 45 and 90 min (0.9170), and 75 and 90 min (0.1406) were not statistically different.
The copper removal efficiency increased from 30 to 60 min, stipulating different copper binding kinetics for the Chlorella biomass (p < 0.05). However, at ≥75–115 min, slightly lower removal efficacy indicated partial saturation or changes in binding site accessibility. The decrease observed could also indicate desorption, where previously adsorbed copper ions are released from the binding sites back into the solution (Chatterjee and Abraham, 2019). Tukey’s post hoc results for copper showed no significant differences in adsorption between 75 and 90 min (p = 0.9999), 75 and 115 min (p = 0.7975), and 90 and 115 min (p = 9,166), indicating a plateau in adsorption efficiency beyond 60 minutes (Supplementary Table S10). It is important to note that at 15 min, copper removal was not apparent, possibly due to the microalgal biomass not having enough time to adsorb copper ions effectively. Thus, the optimum time was established as 60 min, with maximum removals of 70% observed for copper, 95% for lead, and 100% for zinc.
3.4.4 Effects of metal concentration on the degree of removal efficiency by Chlorella biomass
This study explored the effect of metal concentration on the adsorption by Chlorella sp. biomass to understand the dynamic relationship between initial metal ion concentrations and their adsorption behaviour. For this analysis, the other four variables (e.g., biomass dosage, pH, temperature, and contact time were kept constant (Figure 4d). The ANOVA results (p < 0.05) showed that metal concentration significantly affected the adsorption (Supplementary Table S13). A notable increase in copper removal efficiency was observed, from 37% at 0.25 mg/L to 79% at 1.0 mg/L. This could be due to the increased availability of copper ions at higher concentrations (0.5 and 1.0 mg/L), providing more opportunities for adsorption onto the Chlorella biomass. Tukey’s post hoc test confirmed this trend, revealing a significant increase in adsorption between 0.25 mg/L and 0.5 mg/L (p < 0.05), as well as between 0.25 mg/L and 1.0 mg/L (p < 0.05) (Supplementary Table S14). Al-Homaidan et al. (2015) reported a similar trend when assessing the removal of cadmium ions by dried biomass of Spirulina (Arthrospira) platensis. However, the lead and zinc removal efficiencies from 0.25 mg/L to 1.0 mg/L did not change notably. This can also be ascribed to the abundant availability of the binding sites on the biomass surface at low metal concentrations. Tukey’s post hoc test showed no significant difference in lead and zinc removal between 0.25 mg/L, 0.5 mg/L, and 1.0 mg/L (p > 0.05), supporting the observation that their adsorption remained relatively constant at lower concentrations (Supplementary Tables S15, S16). Above metal concentrations of 1 mg/L, a decrease in copper and zinc removal efficiencies was observed, but to a lesser degree for lead. This is statistically supported by Tukey’s post hoc test, which confirmed significant reductions in copper and zinc removal efficiencies at concentrations above 1 mg/L, with all comparisons yielding p-values < 0.05 (Supplementary Tables S15, S16). The increased concentration of heavy metal ions in the solution may overwhelm the active sites, resulting in heightened competition among available ions for limited binding sites (Musumba et al., 2020). Overall, copper showed lower adsorption, contributing to it being less efficiently removed than the other two metals. Kiruba et al. also reported reduced removal as cadmium concentrations increased due to the saturation of the active sites of the surface-modified Eucalyptus seeds system (Kiruba et al., 2014).
3.5 Kinetic studies
The results of adsorption kinetics model coefficients using the dried biomass of Chlorella sp. as a biosorbent are presented in Table 2. The pseudo-first-order kinetic model produced a calculated equilibrium adsorption capacity close to the experimentally determined values, suggesting the accuracy and reliability of the model’s predictive capabilities. The rate constant (k1) for copper illustrated a slow adsorption process (0.045 min-1), while lead and zinc exhibited fast adsorption rates (0.117 and 0.131 min-1, respectively). This can be ascribed to lead and zinc possessing larger electronegativity, hydrolysis constant, atomic weight, ionic radius, and Misono softness values than copper (Al Hamouz and Ali, 2013; Ni et al., 2019; Musumba et al., 2020). The coefficient of determination (R2) ranged from 0.9979 to 0.9974, indicating a perfect fit of the first-order model to the experimental data. The pseudo-second-order kinetic model yielded a slightly less accurate fit for copper, lead, and zinc. The calculated equilibrium adsorption capacities were closer to the experimental values for all 3 metals. The obtained rate constants (k2) indicated slow copper adsorption and a faster lead and zinc adsorption process. The coefficients of determination values were greater or equal to 0.987 for all three metals. For the intraparticle diffusion model, copper showed slower intraparticle diffusion - Kdiff, while lead and zinc exhibited faster rates. The lower R2 values (0.611–0.884) relative to the first and second-order models confirm that intraparticle diffusion alone cannot be the only controlling factor. Thus, the pseudo-first-order kinetic model was the best-fitted model.
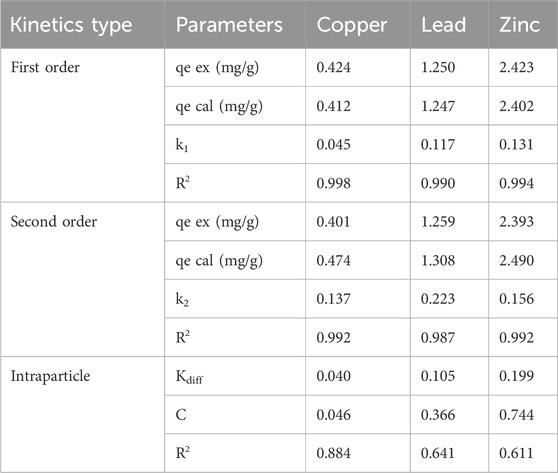
Table 2. The results of adsorption kinetics model coefficients for three metals and Chlorella sp. biomass as a biosorbent.
3.6 Isotherm studies of adsorption
The isotherm results in Table 3 shed light on the distribution of heavy metal ions between the surface of Chlorella sp. cells and the aqueous phase. The Freundlich isotherm results exhibited favourable adsorption behaviours for the 3 heavy metals. The Freundlich exponent (1/n) values were below one for all heavy metals, suggesting that a heterogeneous surface in view of available adsorption sites characterized the adsorption of the heavy metal ions (Abin-Bazaine et al., 2022). The Freundlich constants obtained for the heavy metals affirmed the adsorption capacities. The high R2 values obtained collectively confirmed the suitability of the Freundlich model, which suggests heterogeneous and multi-layered adsorption onto Chlorella biomass (Saleh, 2022). However, copper exhibited highly favoured adsorption according to Langmuir isotherms compared to Freundlich, as evidenced by a substantially higher R2 value of 0.988, indicating monolayer adsorption on a homogeneous surface (Kalam et al., 2021). The dimensionless separation factor (RL) values were less than 1 but greater than zero, indicating favourable adsorption. When the RL values are less than 1, the adsorption is favourable, meaning that the adsorbent material efficiently removes these target analytes from the solution (Selahle et al., 2022). The Temkin isotherm parameters provided information about the interaction strengths between the heavy metal ions and the surface of dried Chlorella sp. cells. Different Temkin constants (KT) indicated varied levels of interaction strength, with lead and zinc showing higher strength. The R2 values obtained (0.8–0.927) exhibited a reasonable fit of the Temkin model.
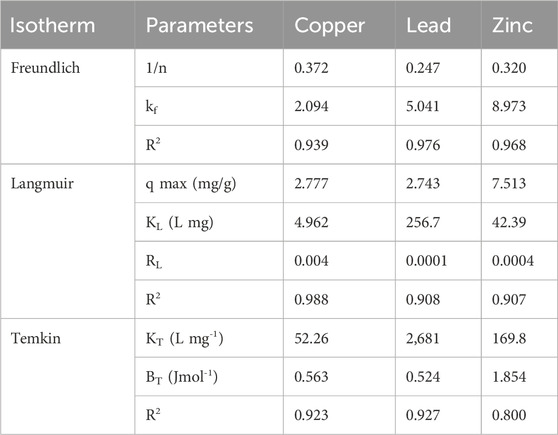
Table 3. The results of Freundlich, Langmuir, and Temkin models biosorption isotherms for three metals and Chlorella sp. biomass as biosorbent.
3.7 Thermodynamic studies of adsorption
Table 4 presents the effect of temperature on copper and zinc adsorption. For copper and zinc, the adsorption thermodynamic parameters calculated illustrate endothermic processes, indicated by the positive enthalpy change (ΔH) of 48.80 kJ/mol for copper, 9.48 kJ/mol for zinc, matching data reported by Musumba et al. (2020). Increased enthalpy during adsorption was denoted by the positive entropy change (ΔS) of 163.74 kJ/mol for copper and 43.63 kJ/mol for zinc, matching a study by Saleh (2022). For the same compounds, negative Gibbs free energy change (ΔG) values (−0.20 kJ/mol to −5.81 kJ/mol) were obtained across tested temperatures, along with via high R2 values (0.989, 0.933), signifying the spontaneous nature of copper and zinc adsorption onto Chlorella biomass, confirming the reliability of the model employed and the data resulting from it.
3.8 Re-usability
Initially, all metals show high removal efficiencies; however, distinct declines occurred in subsequent cycles, with notable differences between the two treatments.
3.8.1 Desorption efficiency of EDTA
For EDTA (Figure 5a), there is a rapid decline in removal efficiencies, particularly by Cycle 2, suggesting that the employed EDTA concentration has limited effectiveness in desorbing metals from the biomass after the first cycle. This is likely because lead (Pb) and zinc (Zn) exhibit strong binding affinities to functional groups, such as carboxyl, hydroxyl, and amino groups, on the Chlorella sp. biomass surface (Al Hamouz and Ali, 2013; Ni et al., 2019; Musumba et al., 2020). These metals form strong ionic bonds or stable complexes that require more energy to disrupt. EDTA at the tested concentration may have acted as a weaker chelating agent, failing to fully break these bonds, leaving residual metals that progressively reduce the biomass’s adsorption capacity over cycles. Thus, increasing the EDTA concentration could potentially improve desorption efficiency by providing a stronger chelating effect. Supporting this, Kumar et al. (2018) reported that desorption capacity increased with higher EDTA concentrations when desorbing cadmium. Other studies have similarly shown that the desorption efficiency of HCl improves with higher concentrations (Wu et al., 2015; Zhang et al., 2021b).
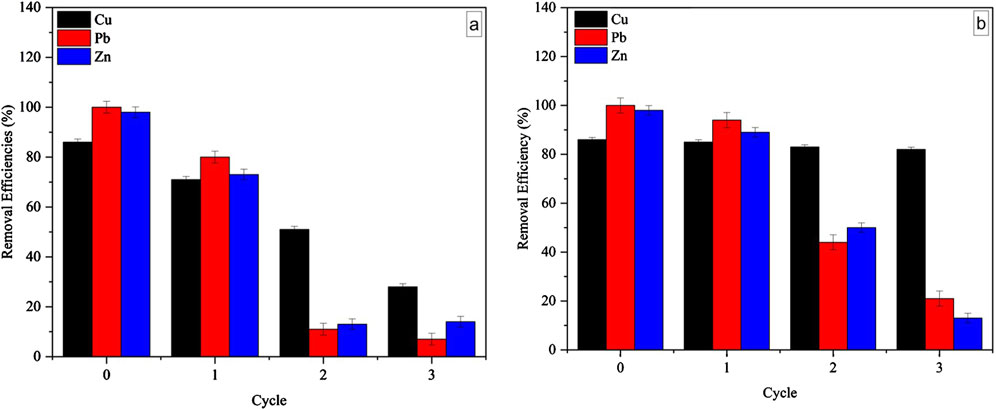
Figure 5. Effect of (a) 0.05 M ethylenediaminetetraacetic acid and (b) 0.05 M hydrochloric acid on the regeneration process and removal efficiency of Chlorella biomass (sample dose: 12.5 mg, temperature: 25°C, time: 120 min) with n = 3 replicates.
3.8.2 Desorption efficiency of HCl
Unlike EDTA, the use of 0.05 M HCl (Figure 5b) resulted in a slightly slower decline in removal efficiencies, suggesting that HCl is more effective at regenerating the biomass for reuse. The acidic environment created by HCl protonates the biomass’s functional groups by donating protons (H+ ions) to surface groups like carboxyl and hydroxyl on the Chlorella sp. biomass (Chatterjee and Abraham, 2019; Zhang et al., 2021b; Yadav et al., 2022). This protonation reduces electrostatic interactions between the biomass and adsorbed metal ions, facilitating metal desorption by weakening the strong bonds with lead and zinc (Martínez et al., 2023). Additionally, HCl promotes ion exchange between hydrogen ions (H+) and the adsorbed metal ions, releasing metals like lead, zinc, and copper more effectively (Qasem et al., 2021). Although HCl demonstrated slightly better performance, after repeated cycles, 0.05 M HCl may still be insufficient to fully disrupt all metal-biomass interactions, particularly for Pb and Zn, which may require a stronger acid or longer desorption time. Moreover, the observed decline in adsorption in later cycles may also be attributed to alterations in the biomass’s surface chemistry, such as damaged physical structure, degraded functional groups, reduced surface area, and decreased porosity, diminishing the biomass’s adsorption capacity in future cycles. Additionally, the desorption process lasted only 2 h, which may not have been sufficient to fully desorb all metal ions, especially Pb and Zn, which form stronger bonds with the biomass. Extending the desorption time could potentially enhance removal efficiency, particularly for metals that bind tightly. The results further indicated that 0.05 M HCl maintains higher removal efficiencies for copper across cycles compared to EDTA. The protonation effect weakens copper’s coordination bonds with the biomass surface (Chatterjee and Abraham, 2019), and HCl also supplies chloride ions, which can form soluble CuCl2 complexes, facilitating copper desorption. This enables the biomass to retain its adsorption efficiency for copper across cycles, resulting in better overall performance with HCl as the desorption agent.
3.8.3 Future optimization strategies
The results of this study showed that while Chlorella sp. offers promise for heavy metal removal, its reusability is limited by a decline in adsorption efficiency after multiple cycles. The slower decline observed with HCl suggests that it may be a more effective desorption agent compared to EDTA. Future studies should focus on optimizing desorption processes, including adjusting desorption times and concentrations, to improve long-term effectiveness. Additionally, exploring ways to maintain or enhance the biomass’s structural integrity and surface properties could help retain its adsorption capacity in subsequent cycles.
3.9 Real wastewater effluent samples analysis
The collected wastewater effluent samples’ physicochemical properties were measured to assess the water quality (Table 5). The samples showed, at the time of sampling, a low dissolved oxygen (DO) content, which was below the recommended limits by the USEPA (6–9 mg/L) and DWAF (7.5 mg/L) (Department of Water Affairs and Forestry, 1995). Such low levels can be ascribed to oxygen consumption by reducing agents (e.g., hydrogen sulfide) as well as biological processes taking place in the presence of organic or inorganic electron donors driving microbial respiration. In addition, aquatic organisms can be negatively affected as copper, lead, and zinc toxicity increases at low DO (Kang et al., 2019; Olabode et al., 2020). At the time of sampling, the temperature of the Darvill effluent was found to be 24.7°C, which would impact the rate and extent of metal adsorption based on thermodynamic grounds (e.g., increased molecular movement and collision frequency) as well as biochemical reactions taking place in water. The pH value is another important parameter affecting the availability of heavy metals. For example, acidic pH levels enhance the solubility of heavy metals, increasing their availability in water. In contrast, alkaline pH levels reduce heavy metals solubility via the production of less soluble hydroxide compounds (Saalidong et al., 2022). However, the pH value of the water sample was 7.39, which is within the acceptable range for discharging domestic and industrial (Department of Water Affairs and Forestry, 1999). (Balali-Mood et al., 2021; Cherono et al., 2021; Prabakaran and Rajan, 2021; Spain et al., 2021) for discharging domestic and industrial wastewater into water bodies (Clean Water Team CWT, 2020). The measured conductivity was 538 µS. This value suggests the presence of dissolved ions, including the possibility of heavy metals (Clean Water Team CWT, 2020). The salinity was 0.27 psu, which agrees with the results of Kunene and Mahlambi (Kunene and Mahlambi, 2019), who reported 0.3 psu for the same site. Total dissolved solids (TDS) denote the overall concentration of dissolved substances, including inorganic salts and other small quantities of organic matter. High TDS can suggest a possibility for the presence of high levels of heavy metals since TDS serves as a carrier for dissolved substances, including heavy metals. In this study, 272 mg/L was obtained.

Table 5. Darvill WWE physicochemical parameters and removal efficiencies for copper, zinc, and lead by dried Chlorella sp. biomass with n = 3 replicates.
The study found lead concentrations of 0.0547 mg/L and zinc concentrations of 0.143 mg/L in Darvill wastewater effluent samples, both of which exceed the South African regulatory limits for discharge into water resources (0.01 mg/L for lead and 0.1 mg/L for zinc). Copper levels were below the detection limit. To assess removal efficiency, wastewater effluent samples were spiked with an optimum concentration of 0.5 mg/L of copper. Excellent metal removal performance was demonstrated for the effluent samples using Chlorella sp. biomass, achieving greater than 98% removal for all heavy metals, signifying that Chlorella sp. is indeed a promising, sustainable, and reliable adsorbent for heavy metal removal in real-world applications.
4 Conclusion
Characterization of Chlorella sp. biomass revealed functional groups (carboxyl, hydroxyl, methyl, carbonyl, and amide) essential for heavy metal adsorption. The maximum removal of copper, zinc, and lead was achieved at pH 7, with a 60-min contact time and an initial concentration of 0.5 mg/L, where 12.5 mg of biomass removed over 80% of zinc and lead, highlighting its potential for effective heavy metal bioremediation. Adsorption kinetics followed a pseudo-first-order model, while Freundlich isotherms described lead and zinc adsorption, while copper adsorption was described by Langmuir isotherm. Such results indicate efficient and favorable adsorption under the tested conditions. Thermodynamic analysis indicated endothermic, spontaneous, and entropically favorable adsorption. Copper and zinc were completely removed in real wastewater, while lead achieved 98% removal.
The results underline Chlorella sp. biomass as a promising bioremediation tool for heavy metal removal from wastewater samples. However, exploring mechanisms tied to adsorption, such as intracellular degradation, bioaccumulation, and metal detoxification, will be crucial for enhancing the efficiency of Chlorella sp. Furthermore, future studies should focus on optimizing desorption and increasing the structural integrity of Chlorella sp. biomass to enhance its long-term reusability. Expanding this research to pilot and full-scale applications will be essential for translating laboratory success into real-world solutions. The collaboration between researchers, industry stakeholders, and policymakers will help integrate these innovative approaches into sustainable water treatment practices, thus contributing to global environmental goals.
Data availability statement
The data is available within the manuscript and supplementary materials. Interested readers should contact the corresponding author.
Author contributions
NT: Conceptualization, Data curation, Formal Analysis, Investigation, Methodology, Project administration, Validation, Visualization, Writing – original draft, Writing – review and editing. SS: Conceptualization, Resources, Writing – review and editing. PM: Conceptualization, Funding acquisition, Project administration, Resources, Supervision, Writing – review and editing.
Funding
The author(s) declare that financial support was received for the research and/or publication of this article. This work was supported by the Moses Kotane Institute (MKI), the Energy and Water Sector Education and Training Authority (EWSETA), and the National Research Foundation (SRUG2204133298). uMgeni-uThekela Water provided the Chlorella strain and funded the strain identification.
Acknowledgments
We would like to acknowledge the Moses Kotane Institute (MKI), the Energy and Water Sector Education and Training Authority (EWSETA), and the National Research Foundation (SRUG2204133298) for financial support. We also acknowledge uMgeni-uThekela Water for providing the Chlorella strain and funding the strain identification.
Conflict of interest
The authors declare that the research was conducted in the absence of any commercial or financial relationships that could be construed as a potential conflict of interest.
Generative AI statement
The author(s) declare that no Generative AI was used in the creation of this manuscript.
Publisher’s note
All claims expressed in this article are solely those of the authors and do not necessarily represent those of their affiliated organizations, or those of the publisher, the editors and the reviewers. Any product that may be evaluated in this article, or claim that may be made by its manufacturer, is not guaranteed or endorsed by the publisher.
Supplementary material
The Supplementary Material for this article can be found online at: https://www.frontiersin.org/articles/10.3389/fenvc.2025.1531726/full#supplementary-material
References
Abd-El-Nabey, B., El-Housseiny, S., Abdel-Gaber, A., and Mohamed, M. (2022). Kinetics of oxidation of metals in the air at room temperature using EDX. Results Chem. 5, 100876. doi:10.1016/j.rechem.2023.100876
Abin-Bazaine, A., Campos Trujillo, A., and Olmos-Marquez, M. (2022). “Adsorption isotherms: enlightenment of the phenomenon of adsorption,” in Wastewater treatment. Editors M. Ince, and O. K. Ince (Rijeka: IntechOpen), 3–7. doi:10.5772/intechopen.104260
Adedoyin, A. E., and Schmidt, S. (2023). Demonstration of the antioxidant potential of three newly isolated carotenoid-producing microscopic algae from KwaZulu-Natal (South Africa). J. Pure Appl. Microbiol. 17 (2), 1163–1178. doi:10.22207/JPAM.17.2.46
Ahmad, A., Bhat, A. H., and Buang, A. (2019). Enhanced biosorption of transition metals by living Chlorella vulgaris immobilized in Ca-alginate beads. Environ. Technol. 40 (14), 1793–1809. doi:10.1080/09593330.2018.1430171
Al Hamouz, O. C. S., and Ali, S. A. (2013). Removal of zinc and cadmium ions using a cross-linked polyaminophosphonate. J. Macromol. Sci. A 50 (4), 375–384. doi:10.1080/10601325.2013.768110
Al-Homaidan, A. A., Alabdullatif, J. A., Al-Hazzani, A. A., Al-Ghanayem, A. A., and Alabbad, A. F. (2015). Adsorptive removal of cadmium ions by Spirulina platensis dry biomass. Saudi J. Biol. Sci. 22, 795–800. doi:10.1016/j.sjbs.2015.06.010
Al-Khiat, S. H., Bukhari, N. A., Ameen, F., and Abdel-Raouf, N. (2023). Comparison of the microalgae Phormidium tenue and Chlorella vulgaris as biosorbents of Cd and Zn from aqueous environments. Environ. Res. 235, 116675. doi:10.1016/j.envres.2023.116675
Almomani, F., and Bhosale, R. R. (2021). Bio-sorption of toxic metals from industrial wastewater by algae strains Spirulina platensis and Chlorella vulgaris: application of isotherm, kinetic models and process optimization. Sci. Total Environ. 755, 142654. doi:10.1016/j.scitotenv.2020.142654
Al-Saadi, A. A., Saleh, T. A., and Gupta, V. K. (2013). Spectroscopic and computational evaluation of cadmium adsorption using activated carbon produced from rubber tires. J. Mol. Liq. 188, 136–142. doi:10.1016/j.molliq.2013.09.036
Bakatula, E. N., Richard, D., Neculita, C. M., and Zagury, G. J. (2018). Determination of point of zero charge of natural organic materials. Environ. Sci. Pollut. Res. 25, 7823–7833. doi:10.1007/s11356-017-1115-7
Balali-Mood, M., Naseri, K., Tahergorabi, Z., Khazdair, M. R., and Sadeghi, M. (2021). Toxic mechanisms of five heavy metals: mercury, lead, chromium, cadmium, and arsenic. Front. Pharmacol. 12, 643972. doi:10.3389/fphar.2021.643972
Barchi, Jr J. J., and Strain, C. N. (2023). The effect of a methyl group on structure and function: serine vs. threonine glycosylation and phosphorylation. Front. Mol. Biosci. 10, 1117850. doi:10.3389/fmolb.2023.1117850
Barczak, M. (2019). Functionalization of mesoporous silica surface with carboxylic groups by Meldrum’s acid and its application for sorption of proteins. J. Porous Mater 26, 291–300. doi:10.1007/s10934-018-0655-7
Briffa, J., Sinagra, E., and Blundell, R. (2020). Heavy metal pollution in the environment and their toxicological effects on humans. Heliyon 6, e04691. doi:10.1016/j.heliyon.2020.e04691
Charazińska, S., Burszta-Adamiak, E., and Lochyński, P. (2021). Recent trends in Ni (II) sorption from aqueous solutions using natural materials. Rev. Environ. Sci. Biotechnol. 21, 105–138. doi:10.1007/s11157-021-09599-5
Chatterjee, A., and Abraham, J. (2019). Desorption of heavy metals from metal loaded sorbents and e-wastes: a review. Biotechnol. Lett. 41, 319–333. doi:10.1007/s10529-019-02650-0
Cherono, F., Mburu, N., and Kakoi, B. (2021). Adsorption of lead, copper, and zinc in a multi-metal aqueous solution by waste rubber tires for the design of a single batch adsorber. Heliyon 7, 1–12. doi:10.1016/j.heliyon.2021.e08254
Clean Water Team (CWT) (2020). “Electrical conductivity/salinity fact sheet, FS3.1.3.0(EC),” in The clean water Team guidance compendium for watershed monitoring and assessment, version 2.0. Division of water quality (California).
Department of Water Affairs and Forestry (1999). General authorisations in terms of section 39 of the National Water Act, 1998 (Act no. 36 of 1998). Government Gazette No. 20526, 8 October 1999, Government Notice. Available online at: https://faolex.fao.org/docs/pdf/saf47843.pdf
Department of Water Affairs and Forestry (1995). Water Research Commission WRC Report No TT 64/94. South African Water quality management series: procedures to assess effluent discharge impacts. Available online at: https://www.wrc.org.za/wp-content/uploads/mdocs/TT%2064-94.pdf
Depci, T., Kul, A. R., and Önal, Y. (2012). Competitive adsorption of lead and zinc from aqueous solution on activated carbon prepared from Van apple pulp: study in single-and multi-solute systems. Chem. Eng. J. 200–202, 224–236. doi:10.1016/j.cej.2012.06.077
El-Naggar, N. E. A., Hussein, M. H., Shaaban-Dessuuki, S. A., and Dalal, S. R. (2020). Production, extraction and characterization of Chlorella vulgaris soluble polysaccharides and their applications in AgNPs biosynthesis and biostimulation of plant growth. Sci. Rep. 10 (1), 3011. doi:10.1038/s41598-020-59945-w
Giarikos, D. G., Brown, J., Razeghifard, R., Vo, D., Castillo, A., Nagabandi, N., et al. (2021). Effects of nitrogen depletion on the biosorption capacities of Neochloris minuta and Neochloris alveolaris for five heavy metals. Appl. Water Sci. 11 (39), 39–15. doi:10.1007/s13201-021-01363-y
Gonte, R., and Balasubramanian, K. (2016). Heavy and toxic metal uptake by mesoporous hypercrosslinked SMA beads: isotherms and kinetics. J. Saudi Chem. Soc. 20, S579–S590. doi:10.1016/j.jscs.2013.04.003
Harris, E. H. (1989). The Chlamydomonas Sourcebook: a comprehensive guide to biology and laboratory use. Academic Press.
Kalam, S., Abu-Khamsin, S. A., Kamal, M. S., and Patil, S. (2021). Surfactant adsorption isotherms: a review. ACS Omega 6 (48), 32342–32348. doi:10.1021/acsomega.1c04661
Kang, M., Tian, Y., Peng, S., and Wang, M. (2019). Effect of dissolved oxygen and nutrient levels on heavy metal contents and fractions in river surface sediments. Sci. Total Environ. 648, 861–870. doi:10.1016/j.scitotenv.2018.08.201
Kiruba, U. P., Kumar, P. S., Prabhakaran, C., and Aditya, V. (2014). Characteristics of thermodynamic, isotherm, kinetic, mechanism and design equations for the analysis of adsorption in Cd (II) ions-surface modified Eucalyptus seeds system. J. Taiwan Inst. Chem. Eng. 45 (6), 2957–2968. doi:10.1016/j.jtice.2014.08.016
Kordialik-Bogacka, E. (2011). Cadmium and lead recovery from yeast biomass. Cent. Eur. J. Chem. 9 (2), 320–325. doi:10.2478/s11532-011-0001-2
Kumar, M., Singh, A. K., and Sikandar, M. (2018). Study of sorption and desorption of Cd (II) from aqueous solution using isolated green algae Chlorella vulgaris. Appl. Water Sci. 8 (8), 225. doi:10.1007/s13201-018-0871-y
Kunene, P. N., and Mahlambi, P. N. (2019). Development and application of SPE-LC-PDA method for the determination of triazines in water and liquid sludge samples. J. Environ. Manage 249, 109415. doi:10.1016/j.jenvman.2019.109415
Li, H., Wang, J., Luo, Y., Bai, B., and Cao, F. (2022a). pH-responsive eco-friendly chitosan–chlorella hydrogel beads for water retention and controlled release of humic acid. Water 14 (8), 1190. doi:10.3390/w14081190
Li, N., Wang, P., Wang, S., Wang, C., Zhou, H., Kapur, S., et al. (2022b). Electrostatic charges on microalgae surface: mechanism and applications. J. Environ. Chem. Eng. 10 (3), 107516. doi:10.1016/j.jece.2022.107516
Li, Q., Song, W., Sun, M., Li, J., and Yu, Z. (2020). Response of Bacillus vallismortis sp. EPS to exogenous sulfur stress/induction and its adsorption performance on Cu(II). Chemosphere 251, 126343. doi:10.1016/j.chemosphere.2020.126343
Ma, R., Wang, B., Chua, E. T., Zhao, X., Lu, K., Ho, S. H., et al. (2020). Comprehensive utilization of marine microalgae for enhanced co-production of multiple compounds. Mar. Drugs 18 (9), 467. doi:10.3390/md18090467
Ma, X., and Jian, W. (2023). Growth conditions and growth kinetics of Chlorella vulgaris cultured in domestic sewage. Sustainability 15 (3), 2162. doi:10.3390/su15032162
Mandalam, R. K., and Palsson, B. (1998). Elemental balancing of biomass and medium composition enhances growth capacity in high-density Chlorella vulgaris cultures. Biotechnol. Bioeng. 59 (5), 605–611. doi:10.1002/(sici)1097-0290(19980905)59:5<605::aid-bit11>3.0.co;2-8
Martínez, R. J., Godínez, L. A., and Robles, I. (2023). “Waste resources utilization for biosorbent preparation, sorption studies, and electrocatalytic applications,” in Valorization of wastes for sustainable development (Elsevier), 395–418.
Musumba, G., Nakiguli, C., Lubanga, C., Mukasa, P., and Ntambi, E. (2020). Adsorption of lead (II) and copper (II) ions from mono synthetic aqueous solutions using bio-char from Ficus natalensis fruits. J. Encapsul Adsorpt. Sci. 10 (4), 71–84. doi:10.4236/jeas.2020.104004
Naicker, K., Mahlambi, P., and Mahlambi, M. (2023). Comparison of ultrasonic and microwave assisted digestion methods for the determination of heavy metals in soil and sediment: the effect of seasonal variations on metal concentrations and risk assessment. Soil Sediment. Contam. Int. J. 32 (3), 320–336. doi:10.1080/15320383.2022.2084032
Naicker, K., Mahlambi, P. N., Mahlambi, M. M., and Nocanda, X. (2024). Assessment of metals and anions in tap, river, wastewater, and sludge: comparison of hotplate- and microwave-assisted digestion. Water SA. 50 (3), 293–306. doi:10.17159/wsa/2024.v50.i3.4044
Nasir, N. M., Jusoh, A., Harun, R., Ibrahim, NNLN, Rasit, N., Ghani, WAWAK, et al. (2023). Nutrient consumption of green microalgae, Chlorella sp. during the bioremediation of shrimp aquaculture wastewater. Algal Res. 72, 103110. doi:10.1016/j.algal.2023.103110
Ni, B. J., Huang, Q. S., Wang, C., Ni, T. Y., Sun, J., and Wei, W. (2019). Competitive adsorption of heavy metals in aqueous solution onto biochar derived from anaerobically digested sludge. Chemosphere 219, 351–357. doi:10.1016/j.chemosphere.2018.12.053
Olabode, G. S., Olorundare, O. F., and Somerset, V. S. (2020). Physicochemical properties of wastewater effluent from two selected wastewater treatment plants (Cape Town) for water quality improvement. Int. J. Environ. Sci. Technol. 17, 4745–4758. doi:10.1007/s13762-020-02788-9
Pachouri, V., Chandramauli, A., Singh, R., Gehlot, A., Priyadarshi, N., and Twala, B. (2024). Removal of contaminants by Chlorella species: an effort towards sustainable remediation. Discov. Sustain. 5 (1), 19. doi:10.1007/s43621-024-00199-1
Pandey, S., and Kumari, N. (2023). “Impact assessment of heavy metal pollution in surface water bodies,” in Advances in environmental pollution research, metals in water. Editors S. K. Shukla, S. Kumar, S. Madhav, and P. K. Mishra (Elsevier), 129–154. ISBN 9780323959193. doi:10.1016/B978-0-323-95919-3.00004-5
Pfeifer, A., and Skerget, M. (2020). A review: a comparison of different adsorbents for removal of Cr (VI), Cd (II), and Ni (II). Turk J. Chem. 44 (4), 859–883. doi:10.3906/kim-2002-21
Piwowarska, D., Kiedrzyńska, E., and Jaszczyszyn, K. (2024). A global perspective on the nature and fate of heavy metals polluting water ecosystems, and their impact and remediation. Crit. Rev. Environ. Sci. Technol. 1, 1436–1458. doi:10.1080/10643389.2024.2317112
Prabakaran, S., and Rajan, M. (2021). “Biosynthesis of nanoparticles and their roles in numerous areas,” in Comprehensive analytical chemistry (Elsevier), 94, 1–47. doi:10.1016/bs.coac.2021.02.001
Qasem, N. A., Mohammed, R. H., and Lawal, D. U. (2021). Removal of heavy metal ions from wastewater: a comprehensive and critical review. NPJ Clean. Water 4 (1), 36–15. doi:10.1038/s41545-021-00127-0
Raji, Z., Karim, A., Karam, A., and Khalloufi, S. (2023). Adsorption of heavy metals: mechanisms, kinetics, and applications of various adsorbents in wastewater remediation—a review. Waste 1 (3), 775–805. doi:10.3390/waste1030046
Saalidong, B. M., Aram, S. A., Otu, S., and Lartey, P. O. (2022). Examining the dynamics of the relationship between water pH and other water quality parameters in ground and surface water systems. PLoS One 17 (1), e0262117. doi:10.1371/journal.pone.0262117
Saleh, T. A. (2022). “Kinetic models and thermodynamics of adsorption processes: classification,” in Interface science and technology (Elsevier), 34, 65–97. doi:10.1016/b978-0-12-849876-7.00003-8
Selahle, S. K., Mpupa, A., Nqombolo, A., and Nomngongo, P. N. (2022). A nanostructured o-hydroxyazobenzene porous organic polymer as an effective sorbent for the extraction and preconcentration of selected hormones and insecticides in river water. Microchem. J. 181, 107791. doi:10.1016/j.microc.2022.107791
Shi, W., Wang, Z., Li, F., Xu, Y., and Chen, X. (2023). Multilayer adsorption of lead (Pb) and fulvic acid by Chlorella pyrenoidosa: mechanism and impact of environmental factors. Chemosphere 329, 138596. doi:10.1016/j.chemosphere.2023.138596
Sikhakhane, S. S. (2001). Cost-benefit analysis of the environmental impacts of Darvill wastewater works, Pietermaritzburg, KwaZulu-natal. MSc dissertation. Pietermaritzburg: Department of Geography, Faculty of Science and Agriculture, University of Natal.
Simelane, L., Mahlambi, P., Rochat, S., and Baker, B. (2024). Removal of antiretroviral drugs from wastewater using activated macadamia nutshells: adsorption kinetics, adsorption isotherms, and thermodynamic studies. Water Environ. Res. 96 (4), e11020. doi:10.1002/wer.11020
Sočo, E., Papciak, D., Domoń, A., and Pająk, D. (2024). Modern treatment using powdered Chlorella vulgaris for adsorption of heavy metals from freshwater. Water 16 (17), 2388. doi:10.3390/w16172388
Soto-Ramírez, R., Tavernini, L., Lobos, M. G., Poirrier, P., and Chamy, R. (2023). Thermodynamic and kinetic insights into the adsorption mechanism of Cd (II) using Chlorella vulgaris cells with coverage modified by culture media engineering. Algal Res. 74, 103179. doi:10.1016/j.algal.2023.103179
Spain, O., Plöhn, M., and Funk, C. (2021). The cell wall of green microalgae and its role in heavy metal removal. Physiol. Plant 173 (2), 526–535. doi:10.1111/ppl.13405
Sultana, N., Hossain, S. Z., Mohammed, M. E., Irfan, M. F., Haq, B., Faruque, M. O., et al. (2020). Experimental study and parameters optimization of microalgae based heavy metals removal process using a hybrid response surface methodology-crow search algorithm. Sci. Rep. 10 (1), 15068. doi:10.1038/s41598-020-72236-8
Tang, R., Dai, C., Li, C., Liu, W., Gao, S., and Wang, C. (2017). Removal of methylene blue from aqueous solution using agricultural residue walnut shell: equilibrium, kinetic, and thermodynamic studies. J. Chem. 2017, 1–10. doi:10.1155/2017/8404965
Tattibayeva, Z., Tazhibayeva, S., Kujawski, W., Zayadan, B., and Musabekov, K. (2022). Peculiarities of adsorption of Cr (VI) ions on the surface of Chlorella vulgaris ZBS1 algae cells. Heliyon 8, e10468. doi:10.1016/j.heliyon.2022.e10468
Umgeni. Annual Report 2022/2023 (2023). Available online at: https://drive.google.com/file/d/1Jp2ZGeI5rgoqb7JCP8wNUJditL_kpoi5/view.
Wang, J., and Guo, X. (2023). Adsorption kinetics and isotherm models of heavy metals by various adsorbents: an overview. Crit. Rev. Environ. Sci. Technol. 53 (21), 1837–1865. doi:10.1080/10643389.2023.2221157
Wohlert, M., Benselfelt, T., Wågberg, L., Furó, I., Berglund, L. A., and Wohlert, J. (2022). Cellulose and the role of hydrogen bonds: not in charge of everything. Cellulose 29, 1–23. doi:10.1007/s10570-021-04325-4
Wu, H., Yao, S., Wang, Y., and Song, H. (2015). “Adsorption of ionic liquids from cellulosic hydrolysate by ion-exchange resin,” in 2015 asia-pacific energy equipment engineering research conference (China Atlantis Press), 261–264.
Yaakob, M. A., Mohamed, RMSR, Al-Gheethi, A., Aswathnarayana Gokare, R., and Ambati, R. R. (2021). Influence of nitrogen and phosphorus on microalgal growth, biomass, lipid, and fatty acid production: an overview. Cells 10 (2), 393. doi:10.3390/cells10020393
Yadav, M., Rani, K., Sandal, N., and Chauhan, M. K. (2022). An approach towards safe and sustainable use of the green alga Chlorella for removal of radionuclides and heavy metal ions. J. Appl. Phycol. 34 (4), 2117–2133. doi:10.1007/s10811-022-02771-6
Yadav, M., Soni, R., Chauhan, M. K., and Sandal, N. (2021). Cellular and physiological approaches to evaluate the chelating effect of Chlorella on metal ion stressed lymphocytes. Biometals 34, 351–363. doi:10.1007/s10534-021-00285-1
Yang, X., Wan, Y., Zheng, Y., He, F., Yu, Z., Huang, J., et al. (2019). Surface functional groups of carbon-based adsorbents and their roles in the removal of heavy metals from aqueous solutions: a critical review. Chem. Eng. J. 366, 608–621. doi:10.1016/j.cej.2019.02.119
Zamora-Ledezma, C., Negrete-Bolagay, D., Figueroa, F., Zamora-Ledezma, E., Ni, M., Alexis, F., et al. (2021). Heavy metal water pollution: a fresh look about hazards, novel and conventional remediation methods. Environ. Technol. Innov. 22, 101504. doi:10.1016/j.eti.2021.101504
Zhang, D., Jiang, W., Liu, L., Yu, K., Hong, M., and Tong, J. (2019). The molar surface Gibbs energy and polarity of ether-functionalized ionic liquids. J. Chem. Thermodyn. 138, 313–320. doi:10.1016/j.jct.2019.06.027
Zhang, K., Dai, Z., Zhang, W., Gao, Q., Dai, Y., Xia, F., et al. (2021a). EDTA-based adsorbents for the removal of metal ions in wastewater. Coord. Chem. Rev. 434, 213809. doi:10.1016/j.ccr.2021.213809
Zhang, K., Dai, Z., Zhang, W., Gao, Q., Dai, Y., Xia, F., et al. (2021b). EDTA-based adsorbents for the removal of metal ions in wastewater. Coord. Chem. Rev. 434, 213809. doi:10.1016/j.ccr.2021.213809
Keywords: biosorption efficiency, adsorption isotherms, kinetic modeling, thermodynamics, metal contamination
Citation: Tenza NP, Schmidt S and Mahlambi PN (2025) Unlocking the potential of Chlorella sp. biomass: an effective adsorbent for heavy metals removal from wastewater. Front. Environ. Chem. 6:1531726. doi: 10.3389/fenvc.2025.1531726
Received: 20 November 2024; Accepted: 18 March 2025;
Published: 11 April 2025.
Edited by:
Yawei Shi, Dalian Maritime University, ChinaReviewed by:
Khuong Nguyen Quoc, Can Tho University, VietnamMasud Hassan, The University of Newcastle, Australia
Copyright © 2025 Tenza, Schmidt and Mahlambi. This is an open-access article distributed under the terms of the Creative Commons Attribution License (CC BY). The use, distribution or reproduction in other forums is permitted, provided the original author(s) and the copyright owner(s) are credited and that the original publication in this journal is cited, in accordance with accepted academic practice. No use, distribution or reproduction is permitted which does not comply with these terms.
*Correspondence: Precious Nokwethemba Mahlambi, bWFobGFtYmlwQHVrem4uYWMuemE=