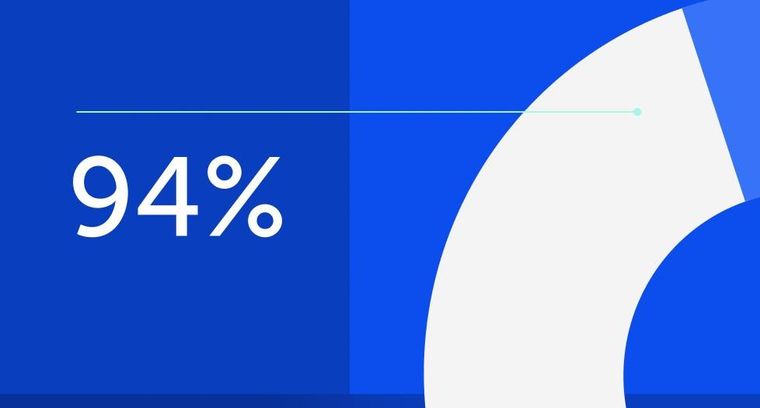
94% of researchers rate our articles as excellent or good
Learn more about the work of our research integrity team to safeguard the quality of each article we publish.
Find out more
ORIGINAL RESEARCH article
Front. Environ. Chem., 04 April 2025
Sec. Inorganic Pollutants
Volume 6 - 2025 | https://doi.org/10.3389/fenvc.2025.1505053
The atmospheric deposition surrounding the Horne Smelter, a major metal processor of electronic wastes in North America has been studied primarily for metals historically associated with local mining operations, but not for other inorganic contaminants (e.g., rare earth elements, REEs) likely related to increasing recycling activities. To address this issue, the present work assessed the atmospheric deposition of a wide range of trace elements (TEs) using complementary monitoring approaches: passive air samplers (PAS) equipped with polyurethane foam (PUF), lichens (Cladonia rangiferina) and spiders (Lycosidae). Sampling was conducted in forest ecosystems (up to 24 sites) along a south-east transect spanning 52 km from the Horne Smelter. Metal concentrations in monitors consistently confirm the deposition of various TE (e.g., As, Cd, Cu, Hg, Pb) associated with the long-term mining activities in the region. Additionally, Hg and Ag were the only two TEs negatively correlated (p < 0.05) with lichen abundance, suggesting a toxic effect. A significant exponential decay regression was observed between TE concentration in the indicators with the distance from the smelter for most metals. Such findings indicated that the Horne Smelter is the main source of TE emission in the area. We also observed a clear enrichment in the first 30 km closest to the smelter compared to farther locations, where similar spatial gradient ranges of TE concentration were reported in the PAS (from 376 to 2) and in lichen (from 297 to 4). We measured, for the first time, levels of REEs and other metals (e.g., V, Mo) in the smelter-impacted area of Rouyn-Noranda. REE data showed no anomalies in their distribution across the sampled sites, suggesting that their source is probably not related to specific enrichment in recycled new technologies. Since the transect spatial results were similar for the PUF-PAS (short-term monitor) and the lichens (longer-term monitor), no significant changes in deposition patterns have occurred in recent years. Further, TEs in spiders were more variable, suggesting that ecological processes may alter this spatial pattern. This study highlights the importance of expanding biomonitoring efforts to include a broader variety of inorganic contaminants for smelter operations of electronic wastes around the world.
Air pollution remains one of the major challenges facing modern societies, despite increased environmental regulation and enhanced public awareness. In the environment, the natural levels of trace elements (TEs) in a given area are generally described as baseline concentrations. Certain industrial activities, such as mining and smelting operations, release a variety of inorganic contaminants that are deposited in the surrounding environments, leading to increased concentrations above baseline levels that disrupt natural biogeochemical cycles (Dinis et al., 2021; Rauch and Pacyna, 2009; Savard et al., 2006; Kasongo et al., 2024). The atmospheric deposition of TEs released from smelting and mining activities impacts terrestrial ecosystems and poses a risk to environmental and public health (Ali and Khan, 2017; Lanier-Christensen, 2015). Since smelter emissions are notoriously subject to « batch processes » that vary over time, monitoring approaches must provide time-integrated information to avoid being merely an instantaneous snapshot.
Monitoring studies that combine the use of several tools reflecting environmental conditions found in different compartments have the potential to accurately gather valuable and time-integrated data on TE atmospheric deposition in adjacent environments (Cucu-man & Steinnes, 2013; Hussain et al., 2022; Gačnik et al., 2024). For example, passive air samplers (PAS), such as TE-200-PAS, collect pollutants by spontaneous adsorption within a polyurethane foam (PUF) filter that has a high retention capacity and low sensitivity to sudden fluctuations in pollutant concentrations (Chaemfa et al., 2009; Gaga et al., 2019). They do not require energy input, and are therefore relatively inexpensive, making them ideally suited for environmental monitoring on large spatial scales and for integrating TE atmospheric deposition over several months in remote sites (Li et al., 2018; Sawvel et al., 2015; Zhang et al., 2022). In addition, biomonitoring organisms are useful to measure levels of bioavailable TEs, i.e., those absorbed in tissues (Cecconi et al., 2021; Heikens et al., 2001; Loppi et al., 1997). Lichens in particular are well-known biomonitors because of their capacity to absorb airborne pollutants (Conti and Cecchetti, 2001; Kousehlar and Widom, 2020; Monaci et al., 2022). Due to their poikilohydric nature, they absorb water, minerals, and contaminants associated with atmospheric deposition (wet and dry) via their entire surface over longer time periods than PAS (Jeran et al., 2007; Loppi et al., 1997). They accumulate TEs in excess of their physiological requirements, making them of prime interest for biomonitoring of mining areas. Several lichen species such as Parmelia sulcata, Evernia mesomorpha, Usnea spp., and Cladonia rangiferina have already been used to assess atmospheric TE contamination in the boreal forest located in Québec, Canada (Carignan and Gariépy, 1995; Carignan et al., 2002; Darnajoux et al., 2015; Widory et al., 2018). To determine TE concentrations in the soil, some studies suggested the use of wolf spiders (Lycosidae) as biomonitors (Jung et al., 2005; Larsen et al., 1994; Ponton et al., 2018). Arthropod predators consume prey inhabiting the soil leading to bioaccumulation in their tissues, which can inform on TE bioavailability within the terrestrial food chain (Polis and Strong, 1996). Approaches based on multi-monitors can provide a better understanding of the contamination emanating from the smelter and mining activities as well as to reflect the level of TE exposure over long periods.
Over more than 90 years, the mining activities of the Horne Copper Smelter have released considerable amounts of elements such as Cd, Cu, Pb, Zn, and As, chronically affecting the terrestrial ecosystems nearby (Bonham-Carter et al., 2006; Darnajoux et al., 2015; Hou et al., 2006; Ministère de l'Environnement et lutte contre les Changements Climatiques, 2018; Telmer et al., 2004). Accumulation and effects of these non-essential and toxic elements were extensively studied in past decades (Couture and Pyle, 2008; Defo et al., 2018). In addition to these contaminants, other TEs have not been measured consistently in the area such as Ba, Cr, Li, Mo, Sb, Se, rare earth elements (REEs), and the platinum-group metals (PGM). Given the Horne Smelter is considered as the largest processor in North America of precious metal-rich materials (e.g., electronic circuit boards, computer parts, hazardous industrial waste, complex concentrates) (Aviseo Conseil, 2019), there is a knowledge gap regarding the emission of contaminants of emerging concern in the area. The effects of these contaminants including REEs have only recently attracted the attention of the scientific community because of their increasing concentrations in aquatic and terrestrial ecosystems (Brown et al., 2019; Khan et al., 2017; MacMillan et al., 2017; Yin et al., 2021) and the lack of current environmental regulation.
To address the knowledge gap regarding TEs released from the Horne Smelter’s recycling activities, we aimed to assess the environmental contamination of a wide range of TEs in terrestrial ecosystems near the Horne Smelter using complementary monitoring tools such as PAS, lichens and spiders. By making comparisons with TE concentrations documented in other regions, we also provided some context to the TE levels found in the most contaminated sites as well as the estimated “geochemical background” for the Rouyn-Noranda region. It was hypothesized that TE emissions from the Horne Smelter also release other TEs that are not included in current biomonitoring studies. These findings will help to better identify the tools needed to track contaminants of historic and emerging concern in the Rouyn-Noranda area during future environmental research activities. Such information can also provide new insights into the nature of environmental emissions derived from the electronic waste recycling activities (e.g., releasing of emerging contaminants), which will be applied not only in similar North American smelters, but also in ones located around the world.
The sampling sites are located near the Horne Smelter (Rouyn-Noranda, region of Abitibi-Témiscamingue, Québec, Canada) (Figure 1). Previous studies reported that the most contaminated sites were downwind of the prevailing winds (eastward elongation; Bonham-Carter et al. (2006)) and within the first 100 km from the smelter (Ettler, 2016; Telmer et al., 2004). Based on these results, sampling sites (n = 24) were selected along a 52 km transect starting from the Horne Smelter and oriented towards the southeast (Figure 1; Supplementary Table S1). All sites are far from other potential sources of TEs such as roads, landfills, settling ponds and mines. To minimize inter-site variability resulting from forest diversity near the Horne Smelter, sampling was only carried out in jack pine stands, an environment with rock outcrops where jack pine (Pinus banksiana), black spruce (Picea mariana), lowbush blueberry (Vaccinium augustifolium), and sheep laurel (Kalmia augustifolia) are commonly found (Kenkel, 1986). These conditions also meet the optimal ecological conditions for finding reindeer lichen C. rangiferina as well as wolf spiders Lycosidae (Koponen, 1987). In addition, the landscape topography of the specific sites is suitable for our purposes because they all have similar elevation (from 282 to 366 m) and they are directly exposed to the atmospheric flow carrying stack emissions loaded with TEs from the smelter (Aznar et al., 2008).
Figure 1. (A) Location of sampling sites (n = 24) along the south-east transect (≈50 km). Sites where passive atmospheric samplers (PAS) and biomonitors (Cladonia rangiferina, Lycosidae) were exposed or collected are indicated; (B) Location of the city of Rouyn-Noranda in the province of Québec, Canada; (C) Wind rose estimates from 1989 to 2019 provided by the Meteorological Service of Canada, climate ID 7086716 et 7086720 (ECCC, 2019). For more details, see Supplementary Table S1.
Passive air samplers (TE-200-PAS, Tisch Environmental) equipped with polyurethane foam (PUF) collection substrate (TE-1014, Tisch Environmental) were used to assess the relative atmospheric TE concentrations in 16 sites (Supplementary Table S1). To minimize background TE concentrations, PUF filters were pre-cleaned prior to deployment using the method optimized by Gaga et al. (2019). PUF filters were rinsed three times with milli-Q water before being ultrasonicated in 1% HNO3 (volume/volume, v/v) for 90 min, followed by a thorough rinse with milli-Q water to remove any residual acid. The pre-cleaning approach significantly reduced the background TE concentrations in the PUFs (Supplementary Table S2), achieving an 18%–92% reduction of TE concentrations, allowing several elements (i.e., As, Ni, V, Se, Ag, Cd, Sb) to fall below the method detection limit (Supplementary Table S2). The acid-washed PUF filters were carefully placed in the TE-200-PAS while wearing gloves. The TE-200-PAS were then fixed to trees approximately 4 m above the ground for 3 months (June to September 2021). The PUF filters were recovered after their 3-month deployment, placed in plastic bags, and stored at room temperature under dark conditions for preservation prior to TE measurements (Gaga et al., 2019).
To assess TE contamination in terrestrial ecosystems, two different biomonitors were used: the lichen C. rangiferina and spiders from the Lycosidae family. Lichens were identified as C. rangiferina based on the substrate, the growth form and the color using the flora guide Lichens of North America (Brodo et al., 2001). Samples (n = 4–5) were carefully collected from each sampling site (23 sites, Supplementary Table S1). To standardize samples and minimize contamination from soil, only the top 2.5 cm of the lichen thallus lobe tips were collected in the laboratory following indications described in Widory et al. (2018). In parallel, we visually estimated the abundance of C. rangiferina in terms of % ground cover within 1 m × 1 m quadrats (n = 5 per site) (Gagnon et al., 2021). Spiders were identified as Lycosidae based on morphology and other characteristics (arrangement of their eyes, ground-dwelling behavior). Individual spiders (n = 3–5) were gently hand-collected from populations at each sampling site (23 in total, Supplementary Table S1). Most Lycosidae sampled were adult females with an egg sac. To reduce differences in TE content due to size, only spiders weighing under 10 mg dw (dry weight) were analyzed. Samples of both biomonitors were then preserved in liquid nitrogen before being rinsed with an EDTA solution (3 mmol L−1) to remove adsorbed TE from their surface. Each sample was placed in 15 mL metal-free tubes for TE measurement.
All lab wares (i.e., tubes, microtubes, spatulas) were soaked in 15% (v/v) nitric acid and rinsed with milli-Q water several times to minimize contamination. For PUF filters and lichens, a microwave-based digestion was performed (Gaga et al., 2019; Charette et al., 2021), while for spiders, a less aggressive digestion using an incubator was applied (Rosabal et al., 2012). Each PUF filter was divided into thirds of ∼100 mg each for determination of total TE concentrations. Microwave digestion of these thirds was performed in a mixture of nitric acid (7.5 mL; 67%–69% HNO3, Optima grade, Fisher Scientific) and hydrogen peroxide (2.5 mL; 30% H2O2, Certified ACS, Fisher Scientific) for 20 min at 200°C in sealed polytetrafluoroethylene (PTFE) vessels (Multiwave 5000, Anton Paar) (Gaga et al., 2019). Ultrapure milli-Q water was then added to reach a total volume of 100 mL before measurements. C. rangiferina samples were freeze-dried for 24 h and homogenized (reduced to fine dust). Analysis of total mercury (THg) was performed on freeze-dried lichen samples using a direct mercury analyzer (DMA-80, Milestone Corp.). Digestion of lichen samples for determination of total TE concentrations was performed in a solution of hydrochloric acid (1 mL; 32%–35% HCl, Optima grade, Fisher Scientific), nitric acid (3 mL; 67%–69% HNO3, Optima grade, Fisher Scientific) and hydrogen peroxide (500 μL, 30%–32% H2O2, Optima grade, Fisher Scientific). To complete the digestion, samples were heated to 200°C for 20 min in closed pressurized vessels in the microwave oven (Multiwave 5000, Anton Paar) (Charette et al., 2021). Ultrapure milli-Q water was then added to reach a total volume of 15 mL. Lycosidae samples were freeze-dried for 24 h and homogenized before digestion in nitric acid (500 μL, 67%–69% HNO3, Optima grade, Fisher Scientific). They were heated at 65°C in an incubator for 4h. Once samples cooled, hydrogen peroxide (250 μL; 30%–32% H2O2) was added to oxidize organic matter (Rosabal et al., 2012). Ultrapure milli-Q water was then added to reach a total volume of 15 mL before TE measurement. All TE measurements were performed using an inductively coupled plasma-tandem mass spectrometer (ICP-QQQ, 8900 Triple Quadrupole, Agilent). Method detection limit for each studied TEs is shown in Supplementary Table S3.
To ensure the accuracy of measured concentrations and to assess the degree of TE extraction of our different digestion protocols, various certified reference materials were also analyzed. Reference materials NIST1573a (tomato leaves, National Institute of Standards and Technology, USA) and BCR-670 (Duck weed, Institute for Reference Materials and Measurements, European Commission) were used to assure quality measurement in lichens for conventional TEs and REEs, respectively. For Hg measurements, blanks were analyzed every 10 samples (No Hg contamination was observed), and certified reference materials (CRMs) TORT-3 (lobster hepatopancreas, National Research Council of Canada) and DORM-2 (fish protein, National Research Council of Canada) were used for quality control. Average Hg recoveries were 97.8% ± 0.3% (n = 3) for DORM-2 and 97.8% ± 2.4% (n = 9) for TORT-3. For spiders, the digestion was evaluated using DOLT-5 (dogfish liver, National Research Council of Canada) for conventional TEs and BCR-668 (mussel tissue, Institute for Reference Materials and Measurements, European Commission) for REEs as these CRMs have already been used for invertebrates (Marginson et al., 2023). The recovery percentages (Supplementary Table S3) for most TEs under study varied from 70% to 120%, with the notable exception of La in BCR-668 which did not meet our quality control requirements; La data were therefore removed from this data set. The detection limit for each TE measured is shown in Supplementary Table S3. NIST1573a, BCR-670 and BCR-668 were used because they are the only CRMs available for REEs in the analyzed matrices.
All numerical data are presented in dry weight and as the arithmetic mean (± standard deviation, SD). Statistical tests were performed using SigmaPlot (10.0) and R (3.6.1). Normality was confirmed using Q-Q plots and the Shapiro-Wilk test. Outliers were identified using Grubbs test and removed from the dataset (<1% of samples). Regression analyses were performed to explore the relationship between TE concentrations and distance from the Horne Smelter. Exponential decay relationship was explored for all metals and three parameters (y0, a, b) were determined (Equation 1) when significant regressions were obtained. The determination coefficient (R2), regression line and equation were reported when the model was significant (p < 0.05). The y0 parameter of Equation 1 was used to estimate TE baseline concentrations.
Spatial metal concentration gradients ([M]maximum/[M]minimum) for each monitor collected over 52 km were calculated using mean TE concentrations in each sampling site. To determine spatial differences compared to the site closest to the Horne Smelter (i.e., the presumed most contaminated site), a Kruskal-Wallis test followed by Dunn’s test (after Bonferroni correction) was applied for TE measurements in samples of PAS, lichen and spiders. The sum of REEs (ΣREE) included Y (and excluded La) as it was strongly correlated to the other REEs (Supplementary Table S4; R2 = 0.85–0.99). REE concentrations in lichen were normalized by the Post-Archean Australian Shale values (PAAS) to assess possible anomalies in their relative proportion, which were calculated using Equation 2 (example from Ce) (Dang et al., 2023; Pourmand et al., 2012).
Non-linear models were used to describe the relationship between C. rangiferina ground cover (%) and distance from the smelter Equation 3. Also, the exponential decay relationship (Equation 1) was explored to relate TE concentrations in lichens with the C. rangiferina ground cover (%).
The concentrations in deployed PUF-PAS showed significant exponential decay relationships with the distance from the Horne Smelter (p < 0.05) for a variety of TEs, including As, Ba, Cd, Cu, Fe, Mn, Mo, Pb, V, Zn and some REEs (Y, Pr and Nd). The negative relationship for Co was linear (p = 0.003) (Figure 2; Supplementary Figure S1). The exponential decay relationships observed between TE concentrations in filters and the distance from the smelter showed a high level of association (based on R2 values), varying from 0.50 (Zn, Y) to 0.97 (Pb, Se) (Figure 2; Supplementary Figure S1). High spatial concentration gradients (ratio: [M]maximum/[M]minimum) were observed along the 52 km transect sampled, where this ratio ranged from 1.7 (Co), 4.5 (Zn), 83.3 (As) to 375 (Cu) (Supplementary Table S5). For most metals, the PUF-PAS deployed after 28.7 km showed significant differences in TE concentration compared with the measurement in the PUF-PAS closest to the Horne Smelter at 1.4 km (i.e., the most contaminated site) (Supplementary Table S6).
Figure 2. Relationship between concentration (μmol g−1 dw) of As (n = 36), Pb (n = 48), Cu (n = 36), Y (n = 47), Pr (n = 47) and Nd (n = 47) measured in PUF-PAS and the distance from the Horne Smelter. Equation, coefficient of determination (R2) and p-value (p) are indicated in the panels.
Similar to the passive air sampler results, highly significant exponential decay relationships were observed between TE concentrations in biomonitor samples and distance from the Horne Smelter (Figures 3, 4, Supplementary Figures S2–S4). However, the decay relationship was much less steep compared to PUF-PAS. For lichens, this relationship was significant for a wide range of TEs (As, Ag, Ba, Pb, Se, Sb, Cu, Ti, Co, Cd, Hg, Ni, Zn, Fe, Mo, V, La, Ce, Pr, Nd, Sm, Gd, Y, Tb, Dy, Ho, Er, Yb) (Figures 3, 4;Supplementary Figures S1–S4), while for spiders, significant exponential decay relationships were observed only for As, Se, Ag and Pb (Figure 3). For Cu and Cd in spiders, a linear relationship with the distance from the Horne Smelter was observed. The spider samples showed TE concentrations that are, in general, considerably greater than the concentrations measured in lichens (more than 50 times higher for Cd). Based on concentrations in lichens and spiders, TE enrichment generally extended up to 28.7 km from the smelter (similar to PAS measurements), after which the bioaccumulation concentrations significantly differed from the TE concentrations of the more contaminated site located at 1.4 km (Supplementary Tables S7–S9). We observed high spatial bioaccumulation gradients for elements previously reported in the area (e.g., As, Cd, Cu, Zn, Pb) as well as for unstudied elements (e.g., Co, Mo, V, Ag, REEs) (Supplementary Table S10).
Figure 3. Relationship between concentration in biomonitor samples (μmol g−1 dw) of As, Se, Cu and Cd and the distance from the Horne Smelter. Lichens are in the left panels and spiders in the right panels. Equation, coefficient of determination (R2) and p-value (p) are given.
Figure 4. Relationship between concentration (μmol g−1 dw) of La (n = 114), Ce (n = 114), Pr (n = 114), Nd (n = 114), Sm (n = 99), Gd (n = 106), Er (n = 77) and ΣREE (n = 114) in lichens samples and the distance from Horne Smelter. Equation, coefficient of determination (R2) and p-value (p) are given.
Despite clear REE enrichment in lichens, where Nd showed the strongest spatial gradient among REE members (19.3), the REE patterns in lichen samples reflect their relative natural abundance in bedrock (Supplementary Figures S5, S6). The average REE concentrations in lichens display a log-linear pattern decreasing with atomic numbers, following the Oddo-Harkins rule, and is consistent regardless of REE concentration (Supplementary Figure S6A). The PAAS-normalized REE concentrations in lichens showed that heavy REEs (i.e., Tb, Dy, Ho, Er, Yb) are slightly enriched compared to light REEs (i.e., Ce, Pr, Nd, Sm) (Supplementary Figure S6B). The REE patterns in lichen samples also exhibited a slight positive Gd anomaly that is consistent regardless of REE concentration.
We recorded the presence of C. rangiferina at a minimum distance of 2.7 km from the smelter. The site closest to the smelter was also surveyed but C. rangiferina was absent. Expressing the impact of reported TE contamination on C. rangiferina abundance, we observed that vegetated ground cover increased with the distance from the Horne Smelter. In the first 5 km from the smelter, C. rangiferina presence was sparse, with ground cover <1%. The abundance of lichens is considerably greater beyond 10–20 km from the smelter, although there was important variability in lichen ground cover within sites (Figure 5A). The bioaccumulated concentrations of Hg (p: 0.05; R2 = 0.69) and Ag (p: 0.05; R2 = 0.58; data not shown) were correlated with lichen abundance in the field (Figure 5B).
Figure 5. Relationship between C. rangiferina ground cover (%, n = 4 per site) in 22 sampling sites and (A). The distance from Horne Smelter. (B) Mean concentration of Hg in C. rangiferina. Equation, coefficient of determination (R2) and p-value (p) are given.
Airborne particulates emitted from smelting operations are often associated with higher concentrations of TEs such as As, Cd, Cu and Pb in the surrounding environments than the natural background values (Kasongo et al., 2024). Smelter-derived particulate emissions from a point source have been shown to induce halo-like contamination patterns, resulting from differential settling due to particle size (Csavina et al., 2011; Csavina et al., 2014; Berryman et al., 2024). The PUF-PAS measurements in this study are consistent with emissions from a point source and showed a clear enrichment within the first 28.7 km of the smelter for most of the TEs studied, which could be related to airborne particles emitted from the smelter. The highest maximum/minimum concentration ratios estimated were for Cu (375), Pb (184), and As (83), respectively, which is comparable with reported stack emissions and field observations in the vicinity of other copper smelters (Fernández-Camacho et al., 2012; Svoboda et al., 2000; Zhang et al., 2022). In addition to Cu, Pb and As, there were also significant spatial concentration gradients for TEs previously unreported in this region such as Ba, Mo, Y, Nd and Pr. The ability of PUF-PAS to detect REEs (i.e., Y, Nd, and Pr) in ambient air is supported by previous studies where dispersion of REEs near smelting facilities was found to be primarily mediated by the atmospheric deposition of fine REE-laden particles (Brewer et al., 2022; Wang et al., 2014). These newly detected elements might be associated with the smelter’s recycling of e-wastes and hazardous materials, suggesting these recycling actions contribute to environmental TE contamination (Bi et al., 2010; Song and Li, 2014). As such activities are expected to increase in coming years, more environmental monitoring efforts should be performed in the future for these emerging TEs.
According to our passive samplers, sites located further away in forested ecosystems (more than 28.7 km from the smelter) showed a stable and relatively low concentration of TE-rich airborne particles, which contrasted with the deposition of TE-enriched smelter dust found up to 100 km away from the Horne Smelter in previous studies (Knight and Henderson, 2006; Telmer et al., 2006). The implementation of emission reduction measures taken in recent decades may partly explain why PUF-PAS measurements detected atmospheric TE enrichment at substantially shorter distances compared to previous studies (Crouse et al., 2019; Diener and Mudu, 2021; Wolf et al., 2020). However, the magnitude of enrichment measured by the PUF-PAS should be viewed with caution as they showed variations in their affinity for different TEs and particle sizes (Gaga et al., 2019; Li et al., 2018). Metals that tend to be found in very fine particles (Cu, Ni) were shown to have significantly higher absorption rates in the PUF than those that are bound to the coarse fraction. In addition, it has been shown that the “unfilterable” phase, comprising gaseous species and solutes in filter-penetrated droplets, accounts for a considerable proportion of the atmospheric TE content, leading to underestimation when considering only the particle collectors (Zhang et al., 2022). Despite the fact that smelter emissions are notoriously subject to batch processes (Ministère de l'Environnement et lutte contre les Changements Climatiques, 2018), the PUF-PAS devices used captured a 3-month snapshot of TE contamination in airborne particles. Passive samplers have the potential to provide low-cost alternatives to traditional air sampling techniques and here they demonstrated their potential to meet the broad sampling requirements posed by smelters, particularly in remote or logistically constrained environments (Kasongo, et al., 2024).
Similar to PUF-PAS measurements, regression analyses of TE concentrations in both biomonitoring organisms showed contamination patterns typical of a point source emission (Ettler, 2016; Fry et al., 2020; Stafilov et al., 2010). Numerous studies have reported analogous patterns using terrestrial biomonitors such as lichens (Bari et al., 2001; Cloquet et al., 2006; Fry et al., 2020; Grodzińska et al., 1999; Gačnik et al., 2024), but none have measured a range of TEs (including contaminants of emerging concern) as broad as in this study. We found a high TE concentration gradient for Pb, Cd, Ni, Sb, Zn, and Cu, which is comparable with previous reports of particulate contamination and likely reflects the major TEs emitted from the Horne Smelter. Consistent with the atmospheric particles analyzed from the PAS, various elements (e.g., Mo, REEs) were newly reported for this region, showing relatively slight TE gradients, but with significant spatial differences compared to the TE concentrations of the closest site to the Horne Smelter. These results reveal gaps in the monitoring of smelter-derived inorganic pollution in the Rouyn-Noranda region, particularly for emerging contaminants such as REEs, which are currently unregulated (Gwenzi et al., 2018). Since several TE measured in lichens are considered strategic and critical metals (e.g., Cu, Ni, Co, REEs, etc.) because of their increasing use in electronics, a link between their emission and the recycling of e-waste and hazardous materials at the smelter is suspected (Song and Li, 2014). Likewise, Sb, also found in the lichens in this study, has been shown to be directly related to emissions from e-waste recycling facilities (Bi et al., 2010). The presence of V in lichens suggests that some particles may be emitted from the sulfuric-acid plant adjacent to the smelter, where V2O5 is used as a catalyst to oxidize SO2 to SO3 (Samuel et al., 1989; Zdanowicz et al., 2006).
Lichen samples also showed TE enrichment extended to approximately 28.7 km from the smelter in the southeast direction, but still lower than the spatial TE deposition reported mostly 20 years before (Knight and Henderson, 2006; Telmer et al., 2006). Although PUF-PAS captures fine atmospheric particles and lichens are able to trap coarse particles within their medulla, both monitors were jointly able to detect significant metal concentration differences in the last sampled 35 km compared to the closest sites (i.e., 3-35 km) to the Horne Smelter. This consistency could reflect a high connection between atmospheric emissions and deposition of various TEs emitted from the Horne Smelter in sampled forest ecosystems. Interestingly, these spatial differences are not only observed for TEs previously found in the area (i.e., As, Cd, Cu, Pb) but also for newly detected TEs (REEs, Co, Mo). In addition, the fact that these two monitors, which provide different time-integrated information (for example, 3 months for PUF-PAS, some years for lichen), yielded similar transect spatial results, means no significant changes in TE deposition patterns occurred in recent years.
Regarding the magnitude of TE accumulation in the vicinity of a smelter, Grodzińska et al. (1999) used the lichen Cladonia stellaris to conduct a biomonitoring study near the Ni-Cu smelter complex in Monchegorsk, one of the most polluted cities in Russia. Compared to their results, in our study lichens sampled at the site closest to the Horne Smelter had similar TE concentrations for Fe, Al, Cu, Cd, Cr, Pb and Zn, but an order of magnitude lower for Co, Mn and Ni. Similarly, a study conducted near the Cu-Ni smelter in the Finnish city Harjavalta reported concentrations of Cu, Ni, Zn, Fe, Mn, Cd, and Pb in C. rangiferina that aligned closely with the maximum concentrations observed in our lichens (Salemaa et al., 2004) (Supplementary Table S11).
Metal concentrations for all studied TEs (except Cr) in areas farther from the smelter were estimated by exploiting the relationship between their concentration in lichens and the distance from an isolated point emission source (Supplementary Table S12). A large-scale study of the TE concentrations in peltigeralean lichens in northern Québec showed Al, Ti, V, Fe, Co, Ni, Cu, Zn, Mo, and Cd levels similar to those calculated from our regression-based estimates of the baseline values for C. rangiferina lichens (Darnajoux et al., 2015). However, our regression-based approach showed Mn concentrations that were an order of magnitude lower, and Pb levels that were 6 times higher than those from Darnajoux et al. (2015). Furthermore, estimated C. rangiferina Cd, Cu and Pb concentrations exceeded those found in terricolous lichen Peltigera canina from Yukon Territory, Canada (Pouillé et al. 2024). It is worth noting that comparisons between species of differing genera may be questionable due to the specific physiology and distinct morphologies of the species. Fortunately, a study conducted by Chiarenzelli et al. (2001) in a remote region of northern Canada (near Otter Lake, Nunavut) showed that concentrations obtained using C. rangiferina are of the same level as our measurements for most TEs, including As, Ag and REEs.
While metal quantification in C. rangiferina provided valuable data, it remains unclear to what extent the observed TE enrichment is due to current smelter operations and to what extent it is due to resuspension of soil particles containing historical TE deposits. Widory et al. (2018) used the relationship between 206Pb/204Pb isotope ratio and Pb concentration in C. rangiferina samples to determine its main atmospheric sources in the Rouyn-Noranda area. Their results showed that Pb enrichment east of the city is the result of binary mixing between emissions from the Horne Smelter and regional Pb background. Although a rigorous source apportionment analysis was not the focus of this study, the Pb enrichment in lichens showing a strong correlation (R2 ≥ 0.89) with the concentrations of Ni, V, Mo, Ag, Sb, Cu, As, Co, Se, Cd, Fe, Al, Zn, ΣREE across 2 orders of magnitude suggests a similarly proportioned mixed origin for these TEs (Supplementary Table S13). A slightly weaker correlation for Mn (R2 = 0.83) compared to the other TEs could be due to a relatively higher proportion of Mn from geogenic sources (Bargagli et al., 2002). As for Ba (R2 = 0.75), Ti (R2 = 0.63) and Cr (R2 = 0.51), the weaker association with Pb concentrations in lichens may be related to a more pronounced mixing with traffic-related emissions (Birmili et al., 2006; Sakata et al., 2021).
The REE log-linear decreasing pattern observed in lichen samples is similar to that previously observed in other sites (MacMillan et al., 2017; Picone et al., 2022) (Supplementary Figure S6A). However, PAAS-normalized concentrations in lichens typically show a clear downward slope, with higher light REEs enrichment relative to heavy REEs. Our results instead showed a somewhat small deviation from a horizontal line, with even a slight enrichment in heavy REEs (Supplementary Figure S6B). This trend should be interpreted with caution because the concentrations further from the suspected source were very low. Apart from the slight Gd anomaly, which may be associated with anthropogenic sources (Lafrenière et al., 2023), our results essentially indicate that the bioaccumulation patterns were relatively uniform across the REE group. This uniformity leads us to suggest that REE enrichment in the vicinity of the Horne Smelter is not particularly associated with e-waste and hazardous recycling activities, but rather to complex concentrate inputs to the smelter containing some amount of REEs. However, analyzes of representative samples of the smelter feed materials would be required to conclusively establish this, especially since no REE enrichment has ever been identified near a copper smelter with recycling activities (Parviainen et al., 2019).
Measurements of C. rangiferina ground cover shows that this biomonitor does not grow near the Horne Smelter. The same situation is observed near the Harjavalta smelter, where C. rangiferina is only present sparsely starting from 2 km away (Salemaa et al., 2004). The sigmoidal relationship between distance from the smelter and abundance of C. rangiferina at the sampling sites is consistent with what would be expected near a point contamination source. Significant correlations between the abundance of C. rangiferina in the field and its TE bioaccumulation were observed, particularly for Hg (R2 = 0.69) and, although to a lesser extent, for Ag (R2 = 0.58). These findings are consistent with the results of Puckett (1976) who reported that both elements are the most toxic metals to lichens, altering their cellular membrane and inhibiting photosynthesis.
With respect to spiders as biomonitors, spatial differences were observed only for As, Se, Cu, and Cd. Correlations have been shown between TE bioaccumulation in invertebrates and the soil TE content (Heikens et al., 2001), indicating the availability of these elements from smelter-contaminated soils. Mean concentrations of bioaccumulated Cu and Cd in spiders from the sites closer to the Vaudray-Joannès Lakes Biodiversity Reserve are at comparable levels, and of the same order of magnitude as studies in other part of the world using ground-dwelling spiders as biomonitors (Jung et al., 2005; Larsen et al., 1994). Previous research conducted near the Vaudray-Joannès Lakes Biodiversity Reserve showed limited inter-individual variability of TE concentrations in Lycosidae (Ponton et al., 2018), but our measurements were more variable within sites, limiting the observation of spatial trends along the studied transect for all TEs. The spider samples for this study were only standardized for weight, which may explain the amount of interindividual variation observed. It is well known that biotic factors such as diet, sex, and age structure can affect the bioaccumulation of TEs in organisms. Also, the lack of spatial differences for the other TEs studied compared to lichens (which is a primary producer) indicates that spider exposure to polymetallic contamination is likely associated, in part, with the dietary pathway as a consumer, rather than being solely atmospheric like lichens. Spiders are also able to reflect the TE deposition from the smelter, but some ecological processes may alter this spatial TE concentration patterns.
The present study used complementary monitoring approaches (based on PAS, lichen and spiders) to assess the atmospheric deposition of a wide range of TEs in the areas located in the southeast transect from the Horne Smelter. Data generated from these three environmental tools consistently demonstrated contamination patterns typical of point source emission for metals already studied in this area (e.g., Cd, As, Cu, Zn, Pb) as well as for newly reported TEs. These results illustrate the need to monitor a wider variety of TEs (including REEs, Mo, V, Ag, Co) in upcoming smelter biomonitoring efforts. In addition, significant spatial differences were observed between the closest sites to the smelter, which indicated a high TE concentrations, and further locations (more than 28.7 km), which showed stable and relatively low TE concentrations. Since the sites with the highest levels of atmospheric TEs in our study include primarily the urban and most densely populated sites in Rouyn-Noranda, more efforts should be made to reduce emissions at the source and to intensify research on the risks associated with exposure to such a complex mixture of TEs (Cao et al., 2017). Additionally, it would be worthwhile to investigate the source apportionment of different TEs using multi-isotope analysis to confirm that they are indeed co-released from the same source. Paleo-environmental studies using both biotic and abiotic archives for temporal reconstruction of TEs contamination from the smelter, particularly for the recently reported TEs, are also highly recommended. Our study provided concentrations for various TEs including those of emerging concern (REEs) with comparable levels to those obtained in other regions without any anthropogenic impact. These data will help developing better monitoring of polymetallic contamination associated with smelting operations and electronic waste recycling, which is of major importance in the area and is likely to continue growing in the near future. The combination of various monitors as strategy to tackle TE contamination proves to be an optimal way to provide time-integrated information and not an instantaneous snapshot of the environmental quality of monitored terrestrial environments.
The original contributions presented in the study are included in the article/Supplementary Material, further inquiries can be directed to the corresponding author.
The manuscript presents research on animals that do not require ethical approval for their study.
JD: Data curation, Formal Analysis, Investigation, Methodology, Validation, Conceptualization, Software, Visualization, Writing–original draft, Writing–review and editing. DP: Data curation, Formal Analysis, Investigation, Validation, Methodology, Writing–original draft. AM: Formal Analysis, Investigation, Validation, Data curation, Writing–original draft. NF: Conceptualization, Formal Analysis, Investigation, Methodology, Validation, Visualization, Writing–review and editing. MA: Conceptualization, Data curation, Formal Analysis, Funding acquisition, Investigation, Methodology, Project administration, Resources, Supervision, Validation, Visualization, Writing–original draft, Writing–review and editing. MR: Conceptualization, Data curation, Formal Analysis, Investigation, Methodology, Software, Validation, Visualization, Writing–original draft, Writing–review and editing, Funding acquisition, Project administration, Resources, Supervision.
The author(s) declare that financial support was received for the research, authorship, and/or publication of this article. This study was funded by Natural Sciences and Engineering Research Council of Canada (NSERC) through a scholarship (JD), Discovery grants (MR, MA) and by the Canada Research Chair Program (MA).
We are thankful for the laboratory and field assistance from Dominic Bélanger, Carlos Cerrejon, Julie Arseneault, Maria Chrifi Alaoui, Véronique Corriveau, Mélanie Primeau, Camille St-Arneault, and Virginie Ricard-Henderson. We acknowledge the members of the Comité Arrêt des Rejets et Émission Toxiques de Rouyn-Noranda (ARET) for accommodation, field assistance and help received during the campaigns. The authors thank Tom Harner (Environmental and Climate Change Canada) for graciously providing the passive air samplers.
The authors declare that the research was conducted in the absence of any commercial or financial relationships that could be construed as a potential conflict of interest.
The author(s) declare that no Generative AI was used in the creation of this manuscript.
All claims expressed in this article are solely those of the authors and do not necessarily represent those of their affiliated organizations, or those of the publisher, the editors and the reviewers. Any product that may be evaluated in this article, or claim that may be made by its manufacturer, is not guaranteed or endorsed by the publisher.
The Supplementary Material for this article can be found online at: https://www.frontiersin.org/articles/10.3389/fenvc.2025.1505053/full#supplementary-material
Ali, H., and Khan, E. (2017). Environmental chemistry in the twenty-first century. Environ. Chem. Lett. 15 (2), 329–346. doi:10.1007/s10311-016-0601-3
Aviseo Conseil (2019). Étude d’impacts économiques du secteur de la transformation du cuivre au Québec. Available at: https://bit.ly/3S6MXuA.
Aznar, J. C., Richer-Laflèche, M., and Cluis, D. (2008). Metal contamination in the lichen Alectoria sarmentosa near the copper smelter of Murdochville, Québec. Environ. Pollut. 156 (1), 76–81. doi:10.1016/j.envpol.2007.12.037
Bargagli, R., Monaci, F., Borghini, F., Bravi, F., and Agnorelli, C. (2002). Mosses and lichens as biomonitors of trace metals. A comparison study on Hypnum cupressiforme and Parmelia caperata in a former mining district in Italy. Environ. Pollut. 116 (2), 279–287. doi:10.1016/s0269-7491(01)00125-7
Bari, A., Rosso, A., Minciardi, M. R., Troiani, F., and Piervittori, R. (2001). Analysis of heavy metals in atmospheric particulates in relation to their bioaccumulation in explanted Pseudevernia furfuracea thalli. Environ. Monit. Assess. 69 (3), 205–220. doi:10.1023/A:1010757924363
Berryman, E. J., Cleaver, A., Martineau, C., Fenton, N. J., Zagrtdenov, N. R., and Huntsman, P. (2024). Capture and characterization of fugitive mine dust around an open pit gold mine in Québec, Canada. Appli. Geochemi. 171, 106099. doi:10.1016/j.apgeochem.2024.106099
Bi, X., Simoneit, B. R. T., Wang, Z., Wang, X., Sheng, G., and Fu, J. (2010). The major components of particles emitted during recycling of waste printed circuit boards in a typical e-waste workshop of South China. Atmos. Environ. 44 (35), 4440–4445. doi:10.1016/j.atmosenv.2010.07.040
Birmili, W., Allen, A. G., Bary, F., and Harrison, R. M. (2006). Trace metal concentrations and water solubility in size-fractionated atmospheric particles and influence of road traffic. Environ. Sci. and Technol. 40 (4), 1144–1153. doi:10.1021/es0486925
Bonham-Carter, G. F., Henderson, P. J., Kliza, D. A., and Kettles, I. M. (2006). Comparison of metal distributions in snow, peat, lakes and humus around a Cu smelter in western Québec, Canada. Geochem. Explor. Environ. Anal. 6 (2-3), 215–228. doi:10.1144/1467-7873/05-090
Brewer, A., Dror, I., and Berkowitz, B. (2022). Electronic waste as a source of rare earth element pollution: leaching, transport in porous media, and the effects of nanoparticles. Chemosphere 287 (Pt 2), 132217. doi:10.1016/j.chemosphere.2021.132217
Brodo, I. M., Sharnoff, S., and Sharnoff, S. D. (2001). Lichens of North America. New Haven: Yale University Press.
Brown, L., Rosabal, M., Sorais, M., Poirier, A., Widory, D., and Verreault, J. (2019). Habitat use strategy influences the tissue signature of trace elements including rare earth elements in an urban-adapted omnivorous bird. Environ. Res. 168, 261–269. doi:10.1016/j.envres.2018.10.004
Cao, S., Duan, X., Ma, Y., Zhao, X., Qin, Y., Liu, Y., et al. (2017). Health benefit from decreasing exposure to heavy metals and metalloid after strict pollution control measures near a typical river basin area in China. Chemosphere 184, 866–878. doi:10.1016/j.chemosphere.2017.06.052
Carignan, J., and Gariépy, C. (1995). Isotopic composition of epiphytic lichens as a tracer of the sources of atmospheric lead emissions in southern Québec, Canada. Geochimica Cosmochimica Acta 59 (21), 4427–4433. doi:10.1016/0016-7037(95)00302-G
Carignan, J., Simonetti, A., and Gariépy, C. (2002). Dispersal of atmospheric lead in northeastern North America as recorded by epiphytic lichens. Atmos. Environ. 36 (23), 3759–3766. doi:10.1016/S1352-2310(02)00294-7
Cecconi, E., Fortuna, L., Peplis, M., and Tretiach, M. (2021). Element accumulation performance of living and dead lichens in a large-scale transplant application. Environ. Sci. Pollut. Res. 28 (13), 16214–16226. doi:10.1007/s11356-020-11797-7
Chaemfa, C., Barber, J. L., Moeckel, C., Gocht, T., Harner, T., Holoubek, I., et al. (2009). Field calibration of polyurethane foam disk passive air samplers for PBDEs. J. Environ. Monit. 11 (10), 1859–1865. doi:10.1039/B903152A
Charette, T., Rosabal, M., and Amyot, M. (2021). Mapping metal (Hg, As, Se), lipid and protein levels within fish muscular system in two fish species (Striped Bass and Northern Pike). Chemosphere 265, 129036. doi:10.1016/j.chemosphere.2020.129036
Chiarenzelli, J., Aspler, L., Dunn, C., Cousens, B., Ozarko, D., and Powis, K. (2001). Multi-element and rare earth element composition of lichens, mosses, and vascular plants from the Central Barrenlands, Nunavut, Canada. Appl. Geochem. 16 (2), 245–270. doi:10.1016/S0883-2927(00)00027-5
Cloquet, C., Carignan, J., and Libourel, G. (2006). Atmospheric pollutant dispersion around an urban area using trace metal concentrations and Pb isotopic compositions in epiphytic lichens. Atmos. Environ. 40 (3), 574–587. doi:10.1016/j.atmosenv.2005.09.073
Conti, M. E., and Cecchetti, G. (2001). Biological monitoring: lichens as bioindicators of air pollution assessment — a review. Environ. Pollut. 114 (3), 471–492. doi:10.1016/S0269-7491(00)00224-4
Couture, P., and Pyle, G. (2008). Live fast and die young: Metal effects on condition and physiology of wild yellow perch from along two metal contamination gradients. Hum. Ecol. Risk Assess. 14 (1), 73–96. doi:10.1080/10807030701790322
Crouse, D. L., Pinault, L., Balram, A., Brauer, M., Burnett, R. T., Martin, R. V., et al. (2019). Complex relationships between greenness, air pollution, and mortality in a population-based Canadian cohort. Environ. Int. 128, 292–300. doi:10.1016/j.envint.2019.04.047
Csavina, J., Field, J., Taylor, M. P., Gao, S., Landázuri, A., Betterton, E. A., et al. (2012). A review on the importance of metals and metalloids in atmospheric dust and aerosol from mining operations. Sci. Total Environ. 433, 58–73. doi:10.1016/j.scitotenv.2012.06.013
Csavina, J., Landázuri, A., Wonaschütz, A., Rine, K., Rheinheimer, P., Barbaris, B., et al. (2011). Metal and metalloid contaminants in atmospheric aerosols from mining operations. Water Air Soil Pollut. 221 (1-4), 145–157. doi:10.1007/s11270-011-0777-x
Csavina, J., Taylor, M. P., Félix, O., Rine, K. P., Eduardo Sáez, A., and Betterton, E. A. (2014). Size-resolved dust and aerosol contaminants associated with copper and lead smelting emissions: implications for emission management and human health. Sci. Total Environ. 493, 750–756. doi:10.1016/j.scitotenv.2014.06.031
Cucu-Man, S.-., and Steinnes, E. (2013). Analysis of selected biomonitors to evaluate the suitability for their complementary use in monitoring trace element atmospheric deposition. Environ. Monit. Assess. 185 (9), 7775–7791. doi:10.1007/s10661-013-3135-1
Dang, D. H., Wang, W., Winkler, G., and Chatzis, A. (2023). Rare earth element uptake mechanisms in plankton in the Estuary and Gulf of St. Lawrence. Sci. Total Environ. 860, 160394. doi:10.1016/j.scitotenv.2022.160394
Darnajoux, R., Lutzoni, F., Miadlikowska, J., and Bellenger, J.-P. (2015). Determination of elemental baseline using peltigeralean lichens from Northeastern Canada (Québec): initial data collection for long term monitoring of the impact of global climate change on boreal and subarctic area in Canada. Sci. Total Environ. 533, 1–7. doi:10.1016/j.scitotenv.2015.06.030
Defo, M. A., Bernatchez, L., Campbell, P. G. C., and Couture, P. (2018). Temporal variations in kidney metal concentrations and their implications for retinoid metabolism and oxidative stress response in wild yellow perch (Perca flavescens). Aquat. Toxicol. 202, 26–35. doi:10.1016/j.aquatox.2018.06.007
Diener, A., and Mudu, P. (2021). How can vegetation protect us from air pollution? A critical review on green spaces' mitigation abilities for air-borne particles from a public health perspective - with implications for urban planning. Sci. Total Environ. 796, 148605. doi:10.1016/j.scitotenv.2021.148605
Dinis, L., Bégin, C., Savard, M. M., and Parent, M. (2021). Impacts of smelter atmospheric emissions on forest nutrient cycles: evidence from soils and tree rings. Sci. Total Environ. 751, 141427. doi:10.1016/j.scitotenv.2020.141427
ECCC (2019). Station results - historical data. Available at: https://climate.weather.gc.ca/historical_data/search_historic_data_stations_e.html?searchType=stnName&timeframe=1&txtStationName=Rouyn&searchMethod=contains&optLimit=yearRange&StartYear=1840&EndYear=2021&Year=2021&Month=8&Day=8&selRowPerPage=2.
Ettler, V. (2016). Soil contamination near non-ferrous metal smelters: a review. Appl. Geochem. 64, 56–74. doi:10.1016/j.apgeochem.2015.09.020
Fernández-Camacho, R., Rodríguez, S., de la Rosa, J., Sánchez de la Campa, A. M., Alastuey, A., Querol, X., et al. (2012). Ultrafine particle and fine trace metal (As, Cd, Cu, Pb and Zn) pollution episodes induced by industrial emissions in Huelva, SW Spain. Atmos. Environ. 61, 507–517. doi:10.1016/j.atmosenv.2012.08.003
Fry, K. L., Wheeler, C. A., Gillings, M. M., Flegal, A. R., and Taylor, M. P. (2020). Anthropogenic contamination of residential environments from smelter As, Cu and Pb emissions: implications for human health. Environ. Pollut. 262, 114235. doi:10.1016/j.envpol.2020.114235
Gačnik, J., Ž ivković, I., Kotnik, J., Bož ič, D., Tassone, A., Naccarato, A., et al. (2024). Comparison of active measurements, lichen biomonitoring, and passive sampling for atmospheric mercury monitoring. Environ. Sci. Pollu. Rese. 31, 35800–35810. doi:10.1007/s11356-024-33582-6
Gaga, E. O., Harner, T., Dabek-Zlotorzynska, E., Celo, V., Evans, G., Jeong, C.-H., et al. (2019). Polyurethane foam (PUF) disk samplers for measuring trace metals in ambient air. Environ. Sci. and Technol. Lett. 6 (9), 545–550. doi:10.1021/acs.estlett.9b00420
Gagnon, A., Fenton, N. J., Sirois, P., and Boucher, J.-F. (2021). Plant community diversity at two reclaimed mine tailing storage facilities in Québec, Canada. Land 10 (11), 1191. doi:10.3390/land10111191Available at: https://www.mdpi.com/2073-445X/10/11/1191.
Grodzińska, K., Godzik, B., and Bieńkowski, P. (1999). Cladina stellaris (Opiz) brodo as a bioindicator of atmospheric deposition on the kola peninsula, Russia. Polar Res. 18 (1), 105–110. doi:10.3402/polar.v18i1.6560
Gwenzi, W., Mangori, L., Danha, C., Chaukura, N., Dunjana, N., and Sanganyado, E. (2018). Sources, behaviour, and environmental and human health risks of high-technology rare earth elements as emerging contaminants. Sci. Total Environ. 636, 299–313. doi:10.1016/j.scitotenv.2018.04.235
Heikens, A., Peijnenburg, W. J. G. M., and Hendriks, A. J. (2001). Bioaccumulation of heavy metals in terrestrial invertebrates. Environ. Pollut. 113 (3), 385–393. doi:10.1016/S0269-7491(00)00179-2
Hou, X., Parent, M., Savard, M. M., Tassé, N., Bégin, C., and Marion, J. (2006). Lead concentrations and isotope ratios in the exchangeable fraction: tracing soil contamination near a copper smelter. Geochem. Explor. Environ. Anal. 6 (2-3), 229–236. doi:10.1144/1467-7873/05-092
Hussain, S., Bharali, P., Koushik, B., and Hoque, R. R. (2022). “Bioaccumulation of metals in lichens and mosses understanding atmospheric deposition, metal-induced modifications and their suitability as biomonitors and bioremediators,” in Bioremediation of toxic metal(loid)s, 57–80. doi:10.1201/9781003229940-4
Jeran, Z., Mrak, T., Jaćimović, R., Batic, F., Kastelec, D., Mavsar, R., et al. (2007). Epiphytic lichens as biomonitors of atmospheric pollution in Slovenian forests. Environ. Pollut. 146 (2), 324–331. doi:10.1016/j.envpol.2006.03.032
Jung, C.-S., Lee, S. B., Jung, M.-P., Lee, J.-H., Lee, S., and Lee, S. H. (2005). Accumulated heavy metal content in wolf spider, Pardosa astrigera (Araneae: Lycosidae), as a bioindicator of exposure. J. Asia-Pacific Entomology 8 (2), 185–192. doi:10.1016/S1226-8615(08)60090-4
Kasongo, J., Alleman, L. Y., Kanda, J.-M., Kaniki, A., and Riffault, V. (2024). Metal-bearing airborne particles from mining activities: A review on their characteristics, impacts and research perspectives. Sci. Total. Envir. 951, 175426. doi:10.1016/j.scitotenv.2024.175426
Kenkel, N. C. (1986). Structure and dynamics of jack pine stands near Elk Lake, Ontario: a multivariate approach. Can. J. Bot. 64 (3), 486–497. doi:10.1139/b86-063
Khan, A. M., Bakar, N. K. A., Bakar, A. F. A., and Ashraf, M. A. (2017). Chemical speciation and bioavailability of rare earth elements (REEs) in the ecosystem: a review. Environ. Sci. Pollut. Res. 24 (29), 22764–22789. doi:10.1007/s11356-016-7427-1
Knight, R. D., and Henderson, P. J. (2006). Smelter dust in humus around Rouyn-Noranda, Québec. Geochem. Explor. Environ. Anal. 6 (2-3), 203–214. doi:10.1144/1467-7873/05-087
Koponen, S. (1987). Communities of ground-living spiders in six habitats on a mountain in Quebec, Canada. Ecography 10 (4), 278–285. doi:10.1111/j.1600-0587.1987.tb00769.x
Kousehlar, M., and Widom, E. (2020). Identifying the sources of air pollution in an urban-industrial setting by lichen biomonitoring - a multi-tracer approach. Appl. Geochem. 121, 104695. doi:10.1016/j.apgeochem.2020.104695
Lafrenière, M. C., Lapierre, J. F., Ponton, D. E., Guillemette, F., and Amyot, M. (2023). Rare earth elements (REEs) behavior in a large river across a geological and anthropogenic gradient. Geochi. Cosmoch. Acta. 353, 129–141. doi:10.1016/j.gca.2023.05.019
Lanier-Christensen, C. (2015). Tainted earth: smelters, public health, and the environment. Glob. Public Health 10 (2), 275–277. doi:10.1080/17441692.2014.986160
Larsen, K. J., Brewer, S. R., and Taylor, D. H. (1994). Differential accumulation of heavy metals by web spiders and ground spiders in an old-field. Environ. Toxicol. Chem. 13 (3), 503–508. doi:10.1002/etc.5620130321
Li, Q., Yang, K., Li, J., Zeng, X., Yu, Z., and Zhang, G. (2018). An assessment of polyurethane foam passive samplers for atmospheric metals compared with active samplers. Environ. Pollut. 236, 498–504. doi:10.1016/j.envpol.2018.01.043
Loppi, S., Nelli, L., Ancora, S., and Bargagli, R. (1997). Passive monitoring of trace elements by means of tree leaves, epiphytic lichens and bark substrate. Environ. Monit. Assess. 45 (1), 81–88. doi:10.1023/A:1005770126624
MacMillan, G. A., Chételat, J., Heath, J. P., Mickpegak, R., and Amyot, M. (2017). Rare earth elements in freshwater, marine, and terrestrial ecosystems in the eastern Canadian Arctic. Environ. Sci. Process. Impacts 19 (10), 1336–1345. doi:10.1039/c7em00082k
Marginson, H., MacMillan, G. A., Grant, E., Gérin-Lajoie, J., and Amyot, M. (2023). Rare earth element bioaccumulation and cerium anomalies in biota from the Eastern Canadian subarctic (Nunavik). Sci. Total. Environ. 879, 163024. doi:10.1016/j.scitotenv.2023.163024
Ministère de l’Environnement et lutte contre les Changements Climatiques (2018). Orientations et références techniques pour la deuxième attestation d’assainissement – Fonderie de cuivre. Available at: http://www.environnement.gouv.qc.ca/programmes/prri/orientations-ref-tech-fonderie-cuivre.pdf.57
Monaci, F., Ancora, S., Paoli, L., Loppi, S., and Wania, F. (2022). Lichen transplants as indicators of gaseous elemental mercury concentrations. Environ. Pollut. 313, 120189. doi:10.1016/j.envpol.2022.120189
Parviainen, A., Casares-Porcel, M., Marchesi, C., and Garrido, C. J. (2019). Lichens as a spatial record of metal air pollution in the industrialized city of Huelva (SW Spain). Environ. Pollut. 253, 918–929. doi:10.1016/j.envpol.2019.07.086
Picone, M., Distefano, G. G., Corami, F., Franzoi, P., Redolfi Bristol, S., Basso, M., et al. (2022). Occurrence of rare earth elements in fledgelings of Thalasseus sandvicensis. Environ. Res. 204 (Pt B), 112152. doi:10.1016/j.envres.2021.112152
Polis, G. A., and Strong, D. R. (1996). Food web complexity and community dynamics. Am. Nat. 147 (5), 813–846. doi:10.1086/285880Available at: http://www.jstor.org.proxy.bibliotheques.uqam.ca/stable/2463091.
Ponton, D. E., Baillargeon, C., and Amyot, M. (2018). Évaluation de la biodiversité benthique et de la contamination récente en éléments traces dans la réserve de la biodiversité des Lacs-Vaudray-Joannès, Rouyn-Noranda, Québec. Rapp. présenté au MELCCFP.
Pouillé, S., Talbot, J., Tamalavage, A. E., Kessler-Nadeau, M. E., and King, J. (2024). Impacts of mineral dust on trace element concentrations (As, Cd, Cu, Ni and Pb) in lichens and soils at Lhù’ààn Mân’ (Yukon Territory, Canada). J. Geophy. Rese. Biogeosci. 129, e2023JG007927. doi:10.1029/2023JG007927
Pourmand, A., Dauphas, N., and Ireland, T. J. (2012). A novel extraction chromatography and MC-ICP-MS technique for rapid analysis of REE, Sc and Y: revising CI-chondrite and Post-Archean Australian Shale (PAAS) abundances. Chem. Geol. 291, 38–54. doi:10.1016/j.chemgeo.2011.08.011
Puckett, K. J. (1976). The effect of heavy metals on some aspects of lichen physiology. Can. J. Bot. 54 (23), 2695–2703. doi:10.1139/b76-290
Rauch, J. N., and Pacyna, J. M. (2009). Earth's global Ag, Al, Cr, Cu, Fe, Ni, Pb, and Zn cycles. Glob. Biogeochem. Cycles 23. doi:10.1029/2008gb003376
Rosabal, M., Hare, L., and Campbell, P. G. C. (2012). Subcellular metal partitioning in larvae of the insect Chaoborus collected along an environmental metal exposure gradient (Cd, Cu, Ni and Zn). Aquat. Toxicol. 120-121, 67–78. doi:10.1016/j.aquatox.2012.05.001
Sakata, K., Takahashi, Y., Takano, S., Matsuki, A., Sakaguchi, A., and Tanimoto, H. (2021). First X-ray spectroscopic observations of atmospheric titanium species: size dependence and the emission source. Environ. Sci. and Technol. 55 (16), 10975–10986. doi:10.1021/acs.est.1c02000
Salemaa, M., Derome, J., Helmisaari, H. S., Nieminen, T., and Vanha-Majamaa, I. (2004). Element accumulation in boreal bryophytes, lichens and vascular plants exposed to heavy metal and sulfur deposition in Finland. Sci. Total Environ. 324 (1-3), 141–160. doi:10.1016/j.scitotenv.2003.10.025
Samuel, V., St-Armand, M., and Arcouette, J. (1989). “Design of the gas cooling and cleaning section of the Horne acid plant,” in Process gas handling and cleaning. New-York: Pergamon Press, 65–77.
Savard, M. M., Bégin, C., Parent, M., Marion, J., and Smirnoff, A. (2006). Dendrogeochemical distinction between geogenic and anthropogenic emissions of metals and gases near a copper smelter. Geochem. Explor. Environ. Anal. 6 (2-3), 237–247. doi:10.1144/1467-7873/05-096
Sawvel, E. J., Willis, R., West, R. R., Casuccio, G. S., Norris, G., Kumar, N., et al. (2015). Passive sampling to capture the spatial variability of coarse particles by composition in Cleveland, OH. Atmos. Environ. 105, 61–69. doi:10.1016/j.atmosenv.2015.01.030
Song, Q., and Li, J. (2014). Environmental effects of heavy metals derived from the e-waste recycling activities in China: a systematic review. Waste Manag. 34 (12), 2587–2594. doi:10.1016/j.wasman.2014.08.012
Stafilov, T., Sajn, R., Pancevski, Z., Boev, B., Frontasyeva, M. V., and Strelkova, L. P. (2010). Heavy metal contamination of topsoils around a lead and zinc smelter in the Republic of Macedonia. J. Hazard. Mater. 175 (1-3), 896–914. doi:10.1016/j.jhazmat.2009.10.094
Svoboda, L., Zimmermannová, K., and Kalac, P. (2000). Concentrations of mercury, cadmium, lead and copper in fruiting bodies of edible mushrooms in an emission area of a copper smelter and a mercury smelter. Sci. Total Environ. 246 (1), 61–67. doi:10.1016/s0048-9697(99)00411-8
Telmer, K., Bonham-Carter, G. F., Kliza, D. A., and Hall, G. E. M. (2004). The atmospheric transport and deposition of smelter emissions: evidence from the multi-element geochemistry of snow, Quebec, Canada. Geochimica Cosmochimica Acta 68 (14), 2961–2980. doi:10.1016/j.gca.2003.12.022
Telmer, K. H., Daneshfar, B., Sanborn, M. S., Kliza-Petelle, D., and Rancourt, D. G. (2006). The role of smelter emissions and element remobilization in the sediment chemistry of 99 lakes around the Horne smelter, Québec. Geochem. Explor. Environ. Anal. 6 (2-3), 187–202. doi:10.1144/1467-7873/05-099
Wang, L., Liang, T., Zhang, Q., and Li, K. (2014). Rare earth element components in atmospheric particulates in the Bayan Obo mine region. Environ. Res. 131, 64–70. doi:10.1016/j.envres.2014.02.006
Widory, D., Vautour, G., and Poirier, A. (2018). Atmospheric dispersion of trace metals between two smelters: an approach coupling lead, strontium and osmium isotopes from bioindicators. Ecol. Indic. 84, 497–506. doi:10.1016/j.ecolind.2017.09.003
Wolf, K. L., Lam, S. T., McKeen, J. K., Richardson, G. R. A., van den Bosch, M., and Bardekjian, A. C. (2020). Urban trees and human health: a scoping review. Int. J. Environ. Res. Public Health 17 (12), 4371. doi:10.3390/ijerph17124371Available at: https://www.mdpi.com/1660-4601/17/12/4371.
Yin, X., Martineau, C., Demers, I., Basiliko, N., and Fenton, N. J. (2021). The potential environmental risks associated with the development of rare earth element production in Canada. Environ. Rev. 29 (3), 354–377. doi:10.1139/er-2020-0115
Zdanowicz, C. M., Banic, C. M., Paktunc, D. A., and Kliza-Petelle, D. A. (2006). Metal emissions from a Cu smelter, Rouyn-Noranda, Quebec: characterization of particles sampled in air and snow. Geochemistry-Exploration Environ. Anal. 6, 147–162. doi:10.1144/1467-7873/05-089
Keywords: environmental monitoring, lichen, passive air samplers, spiders, rare earth elements
Citation: Dupont J, Ponton DE, Marois A, Fenton NJ, Amyot M and Rosabal M (2025) Elemental atmospheric deposition around North America’s largest metal processor of electronic waste (Horne Smelter, Canada). Front. Environ. Chem. 6:1505053. doi: 10.3389/fenvc.2025.1505053
Received: 02 October 2024; Accepted: 17 February 2025;
Published: 04 April 2025.
Edited by:
Robert Peter Mason, University of Connecticut, United StatesReviewed by:
Diego De Souza Sardinha, Federal University of Alfenas, BrazilCopyright © 2025 Dupont, Ponton, Marois, Fenton, Amyot and Rosabal. This is an open-access article distributed under the terms of the Creative Commons Attribution License (CC BY). The use, distribution or reproduction in other forums is permitted, provided the original author(s) and the copyright owner(s) are credited and that the original publication in this journal is cited, in accordance with accepted academic practice. No use, distribution or reproduction is permitted which does not comply with these terms.
*Correspondence: Maikel Rosabal, cm9zYWJhbC5tYWlrZWxAdXFhbS5jYQ==
Disclaimer: All claims expressed in this article are solely those of the authors and do not necessarily represent those of their affiliated organizations, or those of the publisher, the editors and the reviewers. Any product that may be evaluated in this article or claim that may be made by its manufacturer is not guaranteed or endorsed by the publisher.
Research integrity at Frontiers
Learn more about the work of our research integrity team to safeguard the quality of each article we publish.