- 1Department of Chemistry, Université de Montréal, Montreal, QC, Canada
- 2Department of Civil, Geological and Mining Engineering, Montreal, QC, Canada
This study presents a comprehensive assessment of the performance of popular pitcher-type point-of-use (POU) water filters to remove PFAS contaminants from tap waters. The evaluated filters, Brita (Elite and Standard), ZeroWater, Aquagear, and ClearlyFiltered, were tested for their efficacy in removing 75 targeted PFAS, total organic carbon (TOC), total dissolved solids (TDS), chloride, and sulfate from two Canadian tap waters with background Σ75 PFAS concentrations of 13 and 56 ng/L. Overall, the performances of the filters varied depending on the structure of the filter media, the water source, and the specific contaminants present. ZeroWater was the top performer in the case of total PFAS removal. The volume-weighted average removal of total PFAS after 160 L of filtration using Saint-Donat tap water was 99% for ZeroWater, 99% for ClearlyFiltered, 77% for Aquagear, and 20% for Brita (Elite). In the case of Montreal tap water, which had different water characteristics and lower total PFAS levels, the volume-weighted average removal for PFAS was ≈100% for ZeroWater, 96% for ClearlyFiltered, 60% for Aquagear, 48% for Brita (Elite), and 38% for Brita (Standard). Both laboratory and home tests involving ZeroWater filters yielded similar high-performance results using Montreal tap water. Although ZeroWater exhibited high PFAS removal (99%) in Saint-Donat water, TDS and TOC desorption and a significant drop in pH were observed after 80 L, a phenomenon which was explained by the higher total concentration of anions in this water. In contrast, no desorption was observed in Montreal tap water for TDS and TOC due to the lower concentrations of anions. The Aquagear filter demonstrated an unusual increase in concentrations of sulfate after the initial 20 L, which needs further evaluation. This study discusses individual filter performance, the influence of tap water characteristics, and the potential to meet the new NSF guidelines, which provides valuable insights for consumers seeking to choose an appropriate easy-to-use water filtration system to ensure safe and clean drinking water in different regions.
1 Introduction
Contamination of water with emerging contaminants, especially per- and polyfluoroalkyl substances (PFAS), is a growing concern worldwide. These chemicals are used in a wide range of industrial applications, and their persistence in the environment, as well as potential health impacts, have led to increased efforts to eliminate them from water bodies (Teymourian et al., 2021). However, PFAS are not effectively removed by conventional drinking water treatment processes, which means that even if drinking water meets other regulatory standards, it may still contain PFAS at levels of concern (Teymoorian et al., 2023). As a result, when contamination of tap water is discovered, further residential treatment may be required to remove PFAS from tap water before the community system upgrade is put in place.
Point-of-use (POU) drinking water filters are popular due to their ease of use and capacity to reduce exposure to various impurities and contaminants in drinking water. A POU filter is any device designed to provide treatment for an individual tap rather than the entire home. POU filters can be designed to remove chemical or microbial pollutants or improve water aesthetics. POU devices may consist of activated carbon (AC) with or without surface modifications, varied combinations of string-wound sediment filters, ultraviolet lamps, reverse osmosis membranes, redox media, and ion exchange filters, depending on the types of pollutants targeted (Wu et al., 2021).
For PFAS removal applications, using sorbent filters is a common treatment option. However, the adsorbent features (porosity, functional group, particle size), the chemistry of the water (pH, inorganic ions, organic matter) and PFAS characteristics (length of the C-F chain, functional group) all have impacts on adsorption efficiency (He et al., 2022). Consequently, it is important to assess the performance of POU filters under a range of operating conditions.
Herkert et al. (2020) investigated the effectiveness of point-of-entry (POE) and POU residential filters for drinking water for the elimination of seven perfluoroalkyl carboxylic acids (PFCAs), three perfluoroalkyl sulfonic acids (PFSAs), and six per- and polyfluoroalkyl ether acids (PFEAs) in 12 homes in southeastern and central (n = 61) North Carolina (NC, USA) (Herkert et al., 2020). All under-sink two-stage systems tested (>74% - >99% for Southeastern NC, >92% - >99% for Central NC) and reverse osmosis filters tested (>75% - >99% for Southeastern NC, >88%–100% for Central NC) demonstrated great removal for monitored perfluoroalkyl acids (PFAAs) and PFEAs. However, all other activated carbon-containing filters showed variable PFAS removal. PFAS removal efficiency of these filters was dependent on the length of the PFAS chain. Generally, PFAS with long-chains were more easily removed than short-chain PFAS. They also evaluated pitcher filters (n = 13), but only in Central NC, and the removal percentage varied between 36% and 71% for the PFAS they investigated. However, Herkert et al.'s study primarily focused on a limited range of PFAS, and the study was observational.
Another research by Anumol et al. (2015) evaluated the removal efficiency for different organic contaminants (including PFOS and PFOA) of three commercial pitcher-type POU devices (Brita® Riviera eight-cup filter, ZeroWater® eight-cup filter, and PUR™ CR-6000 seven-cup filter) and two refrigerator POU filters (Whirlpool®W10295370 filter and GE® MSWF) (Anumol et al., 2015). Each filter device had an exhaustion time determined by the manufacturer and defined as the time of service or maximum volume of water passed through the filter, which is called the manufacturer’s expected lifetime (MEL). All filters had higher average removal for hydrophobic, nonionic compounds in comparison to hydrophilic compounds. Ionic compound removal (such as PFOS and PFOA) was improved by ion exchange resins in pitcher-type POU filters. The lifetime individual removal efficiencies of PFOS and PFOA were higher when using ZeroWater filter (96.7% and 97.5%, respectively) in comparison to other filters, such as PUR filter (∼85% and 79%, respectively) and Brita filter (57% and 52%, respectively). However, this research evaluated the performance of POU filters only for two targeted anionic PFOS and PFOA, not for the other types of PFAS with different lengths, charges, and functional groups, which can also affect the adsorption efficiency.
In another research in North Carolina (USA), 18 private well consumers were enlisted to evaluate the PFAS removal efficiency of under-sink, activated carbon block water filters. Sample collection was performed monthly for 8 months. Filters under investigation were certified for removing PFOS and PFOA under National Sanitation Foundation (NSF) certification P473, but they were not certified for short-chain PFAAs or PFEAs. A total of 17 PFAS were found in filter influent samples out of 47 targeted analytes (∑PFAS 4.7–131 ng/L). Overall, results showed that the filters effectively removed PFAS (97%–99%) in all influents, including short-chain PFEAs, for the total producer-recommended lifetime of the filters (Mulhern et al., 2021).
It has been reported that well water resources in the municipalities of Fountain, Security, and Widefield, (CO, USA) had PFOS and PFOA levels that exceeded the former U.S. Environmental Protection Agency (USEPA) health advisory level of 70 ng/L. Therefore, commercial POE/POU devices using granular activated carbon (GAC) adsorbents and reverse osmosis treatment were deployed to decrease PFAS exposure from household drinking water systems. All tested reverse osmosis systems were effective in removing PFAS from the influent water, lowering concentrations mostly under analytical detection levels (quantification level = 10 ng/L). The iSpring RCS5T and Flexeon LP-700 reverse osmosis filters did not exhibit any PFAS in the purified water; however, the HydroLogic Evolution™ RO1000 reverse osmosis filter exhibited low PFAS levels in the purified water after shutdown and startup (Patterson et al., 2019). No definite reason could be found to explain this observation.
He et al. (2022) collected 12 household water samples from Beijing (China), before and after POU filters. POU filters made with coconut shell activated carbon had removal percentages from 21% to 99% for 14 PFAS in tap water. Density functional theory (DFT) results demonstrated that the topological structures of PFAS and their hydrophobicity and electrostatic interactions between the adsorbent surface and the powerful electronegative F atoms are the most important factors that affect the adsorption of PFAS on the activated carbon (He et al., 2022). Competitive adsorption tests revealed that coconut shell AC had a higher affinity for PFSAs compared to PFCAs, and amongst the novel PFAS, 6:2 FTS and F-53B could be effectively eliminated from the water, but short-chain PFAAs and Gen-X were more challenging to remove. The FOSA energy of adsorption was higher than the same C8 compound, which can be explained by the molecular structure, including polarizable amide groups that increase the affinity with coconut shell AC.
In this study, we evaluated the effectiveness of different brands of pitcher-type POU filters with or without NSF/ANSI Standard certifications to remove a wider range of targeted PFAS (n = 75) with different functional groups and chain lengths (although not all were detected in the unfiltered tap waters). Additionally, the impact of filtration on other water quality parameters, such as total organic carbon (TOC), total dissolved solids (TDS), chloride, and sulfate were evaluated to provide a comprehensive assessment of the filters’ capabilities as well as gain insight into how the general quality of the tap water might influence PFAS removal. Each POU technology was tested using two different tap water sources from cities in Quebec province, Canada (Saint-Donat and Montreal) with distinct characteristics and PFAS levels. A recent study by Munoz et al. (2023) highlighted the presence of a high level of total PFAS in Saint-Donat’s tap water, ranging from 68 to 82 ng/L (Munoz et al., 2023). This concentration exceeds Health Canada’s newly proposed PFAS threshold of 30 ng/L (Health Canada, 2023). Hence, the results of this research can help the population of areas affected by PFAS contamination in their drinking water to make informed decisions when selecting the more suitable device to protect themselves against these harmful chemicals. The results also include a wide range of PFAS, including some PFAS that are not well studied and more difficult to remove using such filtration systems.
The selected pitcher filters are Brita® Elite™, Brita® Standard filter (model # OB03), ZeroWater (5-Stage), Aquagear, and ClearlyFiltered (Affinity® Filtration Technology). In this list, only ZeroWater and ClearlyFiltered currently hold NSF53 and Water Quality Association (WQA) certifications against PFOA/PFOS, respectively. To date, around 93 different sorbent filter products made by 15 manufacturers have been certified by NSF or the WQA to decrease PFOS and PFOA. However, PFAS are not limited to just these two compounds. There are around between 5,000 and more than 12,000 reported PFAS chemicals with various structures and chain lengths in the environment (Karamat et al., 2023; Sosnowska et al., 2023), which can remain in the water after filtration or may affect the adsorption of other PFAS due to competition effects. Given that previous studies evaluated POU filters with a limited number of targeted PFAS compounds, studies using the natural mixture of PFAS are of interest. Hence, we explored the effectiveness of these filters in removing up to 75 different targeted PFAS compounds using the un-spiked water matrices. It is important to mention that NSF certifications (previously as P473 and now categorized as 53 or 58) were originally based on a threshold of 70 ppt for PFOS and PFOA, the previous advisory limit set by the EPA. However, the NSF 53/58 is currently under review to include more stringent certification criteria for PFAS removal as the EPA has recently updated much lower enforceable levels, called Maximum Contaminant Levels (MCLs). These MCLs now stand at 4 ppt for PFOS and PFOA, alongside thresholds for PFHxS, PFNA, and Gen-X at 10 ppt (EPA, 2024).
The evaluation of pitcher-type POU filters for the removal of different contaminants is more important compared to other types of POU filters. Pitcher-type filters can be deployed more rapidly than plumbed-in systems following contamination and they offer an interesting interim option prior to the implementation of community treatment. These filters are also widely used due to their low initial purchase cost, high availability, and convenience in residential homes. Evaluating the performance of different types of pitcher filters can provide valuable information to consumers and policymakers to ensure that people have access to safe and clean drinking water, especially in areas with high detected and quantified PFAS in tap water. Dissolved ions such as natural organic matter (NOM), chloride, and sulfate can also impact the performance of POU filters, given that several treatment pitcher-filter technologies rely on ion exchange. NOM also competes with PFAS for adsorption onto activated carbon media. This can lead to decreased PFAS removal efficiency and a shorter filter lifetime by clogging the filter. Therefore, considering the impact of dissolved ions is also important when selecting and using POU filters.
2 Materials and methods
2.1 Chemicals and standards
Certified and surrogate PFAS standards were provided by different sources, including Wellington Laboratories (Guelph, ON, Canada), DuPont (Wilmington, DE, USA), Fluobon (Beijing, China), Apollo Scientific, and Synquest Laboratories. Details are provided in Supplementary Tables S1-S2. Methanol, HPLC grade water, HPLC grade water with 0.1% formic acid, and acetonitrile were provided by Fisher Scientific (ON, Canada). NH4OH was purchased from Sigma-Aldrich (MO, USA).
2.2 Sample collection
The tap waters of Saint-Donat, originating from a municipal system fed by untreated groundwater, and Montreal in Quebec, Canada (whose water is produced from the St-Lawrence River) were chosen to evaluate the performance of POU filters. Each device was evaluated with these two tap water sources in order to determine how well the filters perform under different water characteristics. Unfiltered tap waters were collected in multiple pre-cleaned high-density polyethylene (HDPE, 19 L) buckets, stored at 4°C, and analyzed within a week. The tap waters were used without spiking any PFAS. Therefore, the PFAS mixture was different for each tap water.
Before starting the analysis, each POU filter was preconditioned based on the manufacturer’s recommendations. The POU filters were kept at ambient temperature during the experiment (∼20 C°). Pre-cleaned HDPE bottles (500 mL) were used for the collection of filtered and unfiltered tap water at each level of sample collection. The bottles had been rinsed with deionized water, followed by 50:50 methanol/HPLC grade water and HPLC water prior to PFAS sampling. Filtered water samples in the lab batch experiment were collected directly under each POU filter after passing 20 L, 40 L, 80 L, and 160 L of tap water, ensuring no contact with the pitcher materials. The filter was then returned to the pitcher to proceed to the next sampling point. Controls (unfiltered samples) were collected at the same time as collecting the filtered samples to have an accurate removal percentage. In order to describe the average exposure of consumers using the filtration devices, the volume-weighted average removals were calculated for each POU device using the following equation:
n is the number of volumes tested (4), Ri is the removal measured after Vi, Vi is the volume of water filtered at step i (each i corresponds to a specific interval between two consecutive data points), and Vn is the total volume filtered = 160 L.
Each POU filter was operated for 8 h per day with a rest period of 16 h. Pitcher filters were gravity-fed using sequential batches of water (2 L) pumped in the top receptacle of the pitcher. All samples were kept at 4°C immediately after collection and tested within a month for PFAS analysis.
2.3 POU devices
This research evaluated the performance of five commercially available pitcher-type POU filters, including Brita® Elite™, Brita® Standard filter (model # OB03), ZeroWater (5-Stage), Aquagear, and ClearlyFiltered (Affinity® Filtration Technology) (Table 1). The choice of these pitcher filter devices is due to their small size, ease of use, cartridge replacement, and ease of transport in each area, and the choice of these brands is due to their availability in the North American area, which also has NSF/ANSI or similar certificates. NSF, as a non-profit organization, certifies a broad range of industrial and domestic products, such as home water purification systems. Each filter was tested in duplicate (except Brita Standard) but with different tap water sources. The selected POU filters all contain activated carbon and/or ion exchange resin media. The inside structure of each device can be seen in Supplementary Figures S1-S5.
2.4 Analysis methods
Off-line automated solid phase extraction (SPE) (Thermo/Dionex Autotrace 280 system) was used for the extraction of PFAS from water using Strata X-AW, 200 mg/6 mL from Phenomenex as SPE cartridge. A 100 μL surrogate internal standards (IS) mixture (10 μg/L in MeOH) was spiked to each of the tap water samples before doing SPE, and the pH of the samples was adjusted to 6.5 with acetic acid to improve retention of short-chain PFAS. The cartridges were first conditioned with 0.2% (v/v) NH4OH (from 28% to 30% solution) in MeOH (2 × 4 mL) followed by 2 × 4 mL of HPLC-grade water. After loading the samples (flow rate of 10 mL/min), cartridges were dried using nitrogen flow for about 30 min. Finally, PFAS analytes were eluted with 2 × 4 mL of 0.2% (v/v) ammonium hydroxide in methanol. Further evaporation was done for all samples under mild nitrogen gas and temperature (40°C) to reach 250 μL. Extracted samples were injected into an ultra-high-performance liquid chromatograph coupled with a high-resolution Q Exactive Orbitrap mass spectrometry (UHPLC-HRMS) with negative and positive ionization modes (150–1,000 m/z, full scan mode) (Munoz et al., 2023). More information about the UHPLC-HRMS acquisition method is provided in (Supplementary Table S3).
The TOC of the influents and effluents was determined using a TOC analyzer (Sievers M5310 C, USA). Anions were measured with an ion chromatograph (ICS 5000 AS-DP DIONEX, USA) equipped with an AS18 column. The TDS of influent and effluent samples were also measured.
2.5 Quality assurance and quality control
Method blanks were used during each batch of SPE to check for any contamination that may have arisen during the analysis. After the initial calibration verification, continuing calibration verification was performed after every 10th water sample in LC-HRMS. Limits of detection (LODs) are presented in Supplementary Table S4. As HRMS yielded PFAS signals with low or unmeasurable background noise within the set mass tolerance threshold, the traditional signal-to-noise method used to derive LODs of LC-MS/MS methods could not be reproduced here. Instead, the LODs were calculated by multiplying the standard deviation of the blanks by the (n-1, 95) student coefficient (n is the number of replicate blanks), when applicable (Muir and Sverko, 2006). LODs were also calculated based on the peak intensity of low-end calibration curve levels or field samples with low concentrations (the LOD was derived using an absolute height of 1E4) (Kaboré et al., 2018). The reporting method LODs were then set at either the blank-derived or height-derived value, whichever was greater. We note that an alternative method described in EPA LC-MS/MS methods for PFAS in drinking water derives detection limits (DL) from the standard deviation of spike replicates (e.g., EPA method 537.1), which likely would be valid for LC-HRMS. Accuracy (Supplementary Table S5) was also assessed by ensuring that the mean accuracy values were within 70%–130% (for n = 3), with relative standard deviations mostly lower than 20% according to the US EPA method (Shoemaker and Tettenhorst, 2018; EPA, 2019; Goeury et al., 2022).
3 Results and discussion
Table 2 provides the water quality parameters for the two tap water sources. The turbidity of unfiltered tap water of Montreal and Saint-Donat was less than 1 NTU (0.143 and 0.085 NTU, respectively). Saint-Donat tap water generally exhibited higher levels of TDS and chloride compared to Montreal tap water. On the other hand, Montreal tap water showed higher levels of TOC and sulfate in contrast to Saint-Donat tap water.
The observed flow rate of each POU device was different for Saint-Donat and Montreal tap waters as the physicochemical characteristics of the water can affect the flow rate. The average flow rate of ZeroWater was 99 mL/min for Saint-Donat tap water; however, it was 127 mL/min for Montreal (+28%), even though Montreal tap water had slightly higher turbidity (0.143 vs. 0.085 NTU). Aquagear filter had a 70 mL/min average flow rate for Saint-Donat tap water, and for Montreal tap water, it was 89 mL/min (+27%). The average flow rate for the Brita (Elite) filter was 115 mL/min for Montreal but was not recorded for Saint-Donat tap water. ClearlyFiltered also had a +29% higher average flow rate (53 mL/min) for Montreal tap water compared to Saint-Donat (41 mL/min), and it was the product with the lowest flow rate among all investigated POU devices. As the flow rate is correlated with the contact time of water inside the filter, such variation in flow could also contribute to variability in sorption performance (Belkouteb et al., 2020).
The average total concentration of PFAS in the source water of Saint-Donat was also higher than in Montreal, with different contribution percentages of each PFAS (Figure 1). During the analysis of influent water samples from Saint-Donat, 27 out of the 75 targeted PFAS compounds were not detected. Similarly, 29 out of the 75 targeted PFAS compounds were also not detected in the Montreal influents. Generally, higher initial contaminants concentrations can result in a faster breakthrough, as the filter may become saturated sooner.
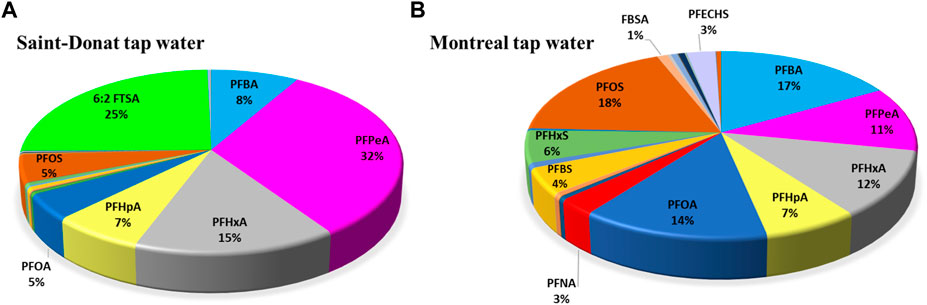
Figure 1. The average contribution percentages of each detected PFAS in the tap water of (A) Saint-Donat (Σ75 PFAS = 56 ng/L), (A) and Montreal (Σ75 PFAS = 13 ng/L).
ZeroWater performance: Following the implementation of the ZeroWater filter, a majority of the detected PFAS compounds in both sources of effluents were successfully reduced below the detection limit throughout the entire test period (160 L). This filter demonstrated superior PFAS reduction in the effluents with volume-weighted average removal after 160 L above 99% for Σ75 PFAS (as well as for PFOS + PFOA) for both sources of water (Figure 2) during water sampling volumes (20–160 L) compared to all other POU filters tested likely due to its five-stage filtration system, in which activated carbon and anionic and cationic ion exchangers (AIX and CIX) play a prominent role (Supplementary Figure S1). While this explanation offers insights, it is crucial to acknowledge that definitive conclusions would require further investigations, considering factors such as contact time and carbon selection, as well as other key design criteria that could influence the adsorption performance.
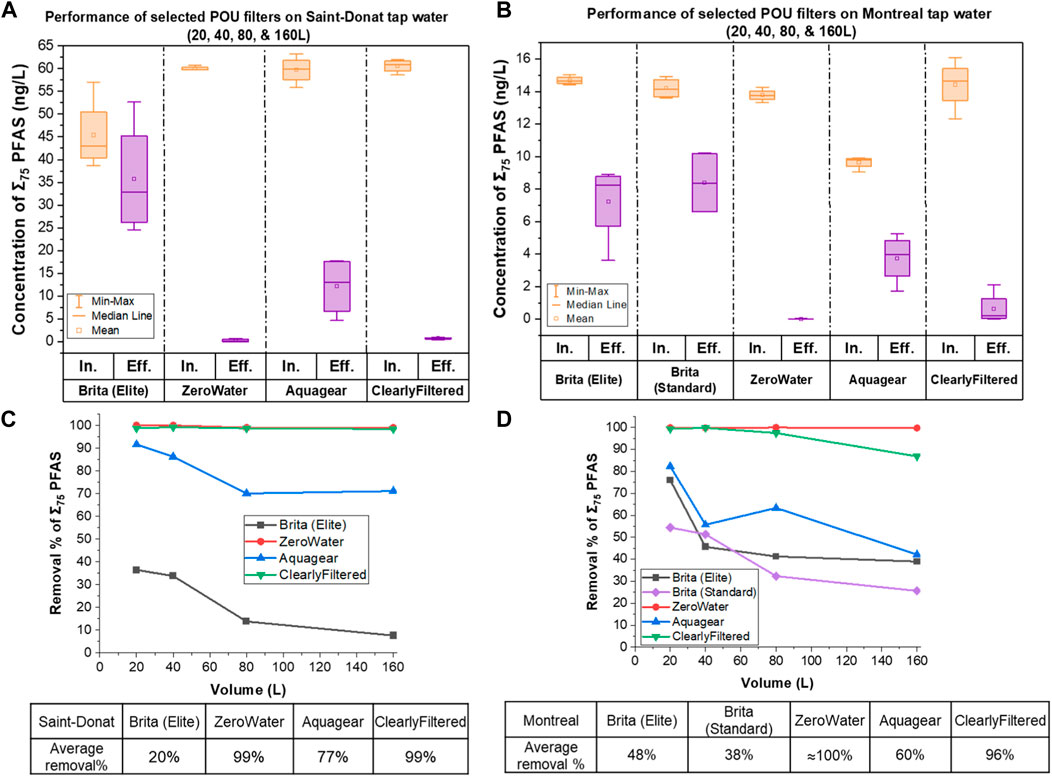
Figure 2. The removal performance of a Σ75 PFAS for selected POU filters with increasing filtered volumes (20, 40, 80, and 160 L). (A) and (B) show the changes in concentrations of the Σ75 PFAS in influents and effluents in Saint-Donat and Montreal tap water, respectively. Removal (in %) of Σ75 PFAS for each POU filter as well as volume-weighted average removal % for (C) Saint-Donat and (D) Montreal tap waters.
Figures 3, 4 also present the removal percentages of the most quantified PFAS compounds found in the tap water of Saint-Donat and Montreal, respectively (compounds that were not detected were considered as 0). ZeroWater consistently maintained high removal percentages, reaching mainly ∼100% for all chain lengths and different functional groups using Montreal tap water. However, in the case of PFBA in Saint-Donat waters, removal was slightly reduced at 94%, after 160 L, probably due to higher levels of total PFAS, chloride and TDS (Table 2) and the challenges in sorbing short-chain PFAS on activated carbon and AIX due to the more hydrophilic nature of shorter chain PFAS. Earlier studies have indicated that the primary mechanism responsible for removing short-chain PFAS is the electrostatic interaction with the adsorbent (Li et al., 2023). However, in the case of Saint-Donat water, this mechanism in ZeroWater may have been exhausted sooner compared to Montreal tap water. Although the PFAS removal was quite stable over 160 L of operation, large fluctuations in other monitored water quality parameters were observed, especially in Saint Donat waters.
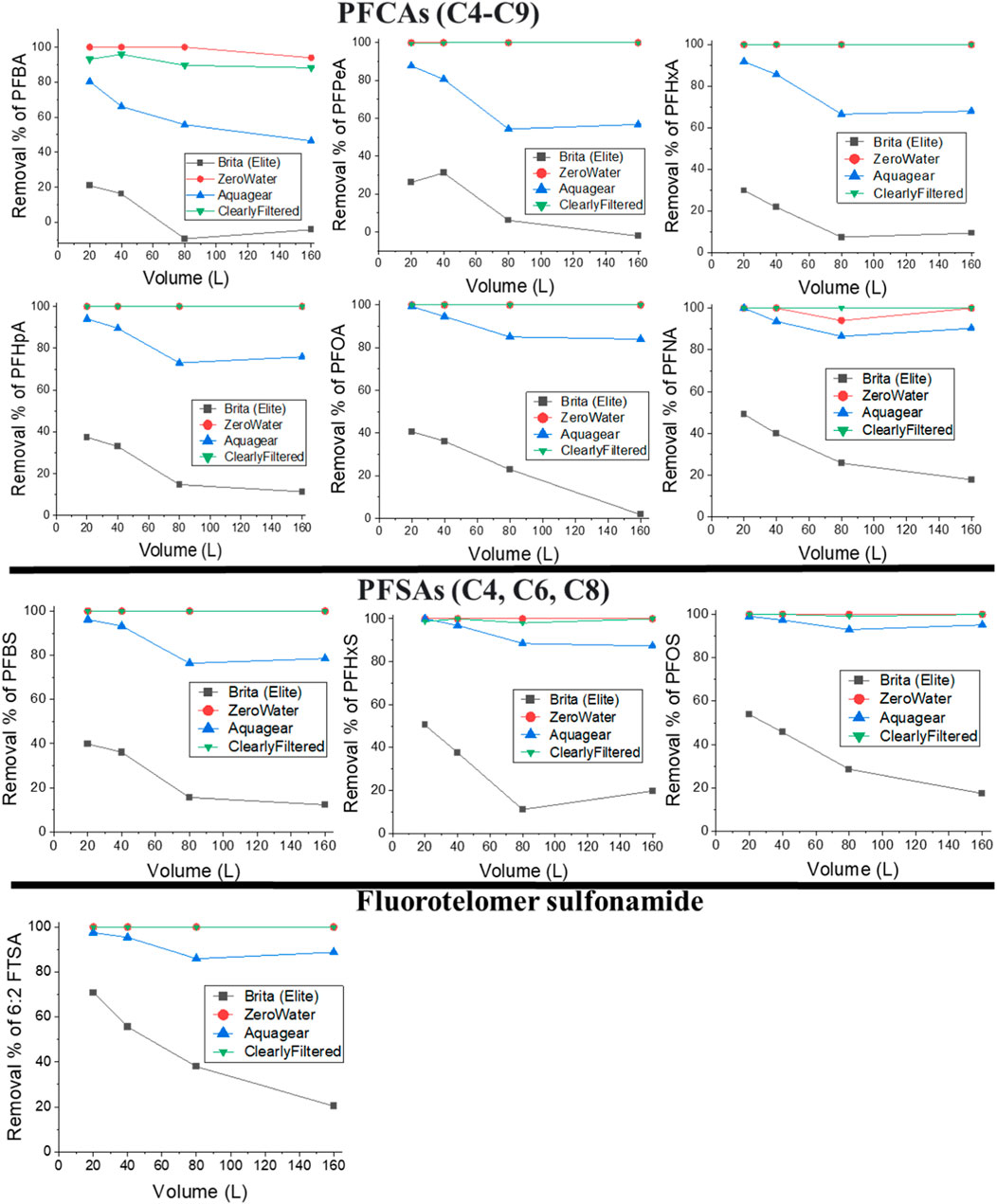
Figure 3. Removals (in %) of the highest quantified PFAS, categorized into three groups: PFCAs, PFSAs, and fluorotelomer sulfonamide in Saint-Donat tap water after filtration of 20–160 L.
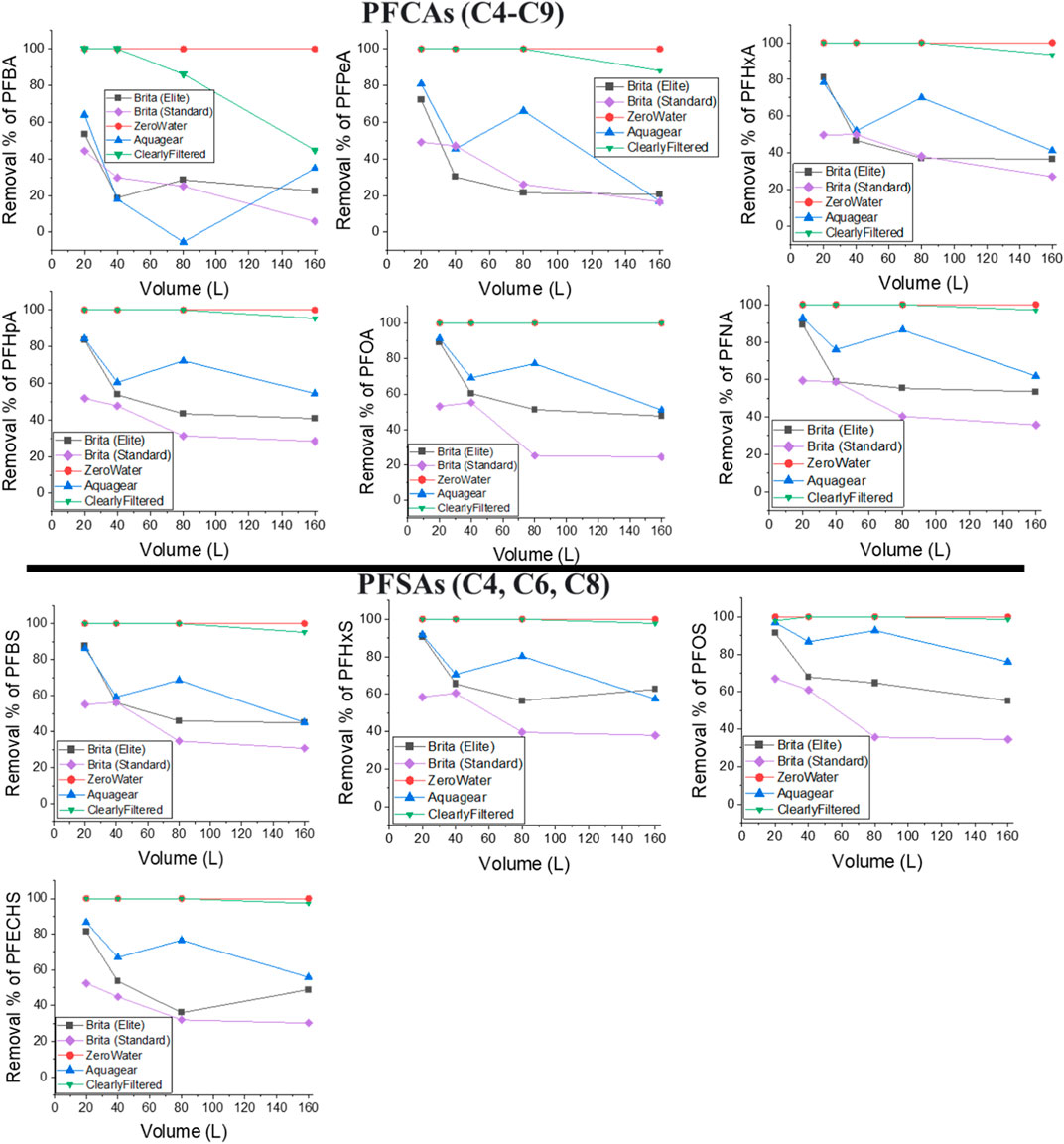
Figure 4. Removals (in %) of the highest quantified PFAS, categorized into two groups: PFCAs and PFSAs (linear and cyclic) in Montreal tap water after filtration of 20–160 L.
Supplementary Tables S6, S7 present pH variations of the Saint-Donat and Montreal influents and effluents, focusing on the performance of evaluated POU filters. ZeroWater exhibited a remarkable pH drop for Saint-Donat tap water after processing 80 L, decreasing from 6.03 to an acidic pH of 2.67, indicating highly acidic conditions in the filtered water. The ZeroWater instructions indicate that when the filtered water becomes acidic, the filter is exhausted and must be replaced. Finally, after filtering 160 L, the effluent pH slightly improved to 5.63, probably due to the exhaustion of the CIX resin. Such a sharp decrease in pH after 80 L suggests that the filter includes an AIX resin loaded with OH− which was exhausted before the H+ loaded CIX resin and, therefore, no longer able to neutralize the H+ released by the CIX. The results suggest that there may be more CIX than AIX resin in the filer (in terms of capacity, not necessarily volume), causing the AIX to become exhausted before the CIX. This imbalance leads to a rapid drop in pH, resulting in a lemon-like taste in the filtered water, which is unpalatable for drinking. This observation raises an intriguing possibility for future research on optimizing the resin composition in ZeroWater filters by introducing more AIX resin to balance it with CIX in the system to potentially enhance their lifespan.
Similar fluctuations were also observed for TOC and TDS after 80 L. TOC removal in the case of Saint-Donat tap water (initial concentration (n = 4) of 0.83 mg C/L) showed an efficiency of about 93% after 40 L, but after reaching 80 L, the removal decreased to −81%, i.e., organic matter actually desorbed from the filter media during the low pH event. Fortunately, PFAS removal was not impacted during this event. The filter freed up adsorption sites during the desorption event, resulting in approximately 25% TOC removal after 160 L of water sampling (Figure 5). A similar trend was also observed for TDS using Saint-Donat tap water (average in influents (n = 4): 191 mg/L), where ZeroWater achieved about 100% removal after 40 L, but the removal dropped to −54% after 80 L, indicating desorption of some TDS. Subsequently, with additional filtration (160 L), the removal efficiency increased to only 7%, presumably because of the same reason for TOC. IX is notorious for producing elution peaks in relation to the affinity of the contaminants (Liu et al., 2021).
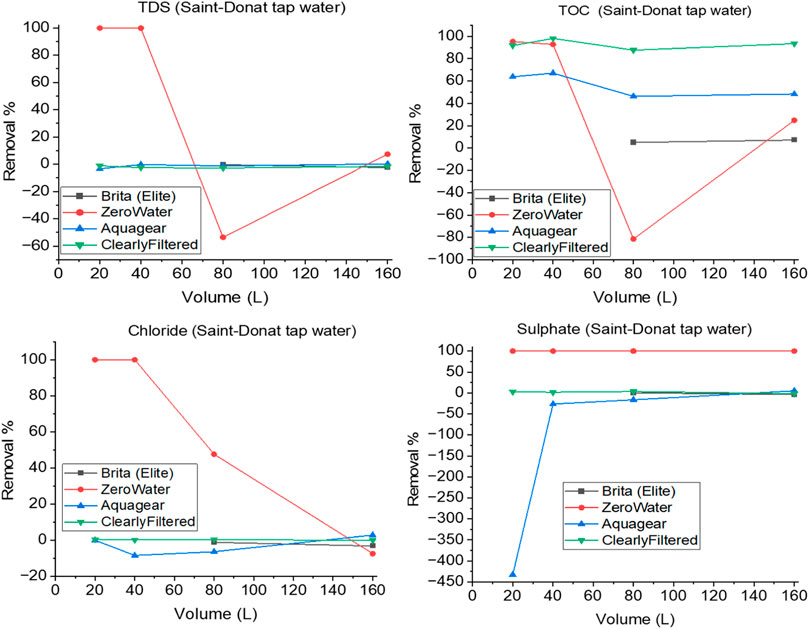
Figure 5. TDS, TOC, chloride, and sulfate removal % for selected POU filters using Saint-Donat tap water. Note: The data for the collected samples at 20 L and 40 L for Brita (Elite) is not available.
In contrast to Saint-Donat, ZeroWater exhibited better and more stable performance in Montreal tap water, likely due to lower levels of anions (2.7 vs. 4.9 mEq/L in Saint-Donat tap water Table 2), which may have resulted in less competition for adsorption on the filter structure. As a result, in Montreal tap water, ZeroWater achieved around 83% TOC removal after 80 L with an initial average concentration of 2.3 mg C/L (n = 4), which reached 53% at the end of water sampling (160 L). The high removal of TDS was also stable after 80 L, with a reduction to 93% after 160 L (Figure 6).
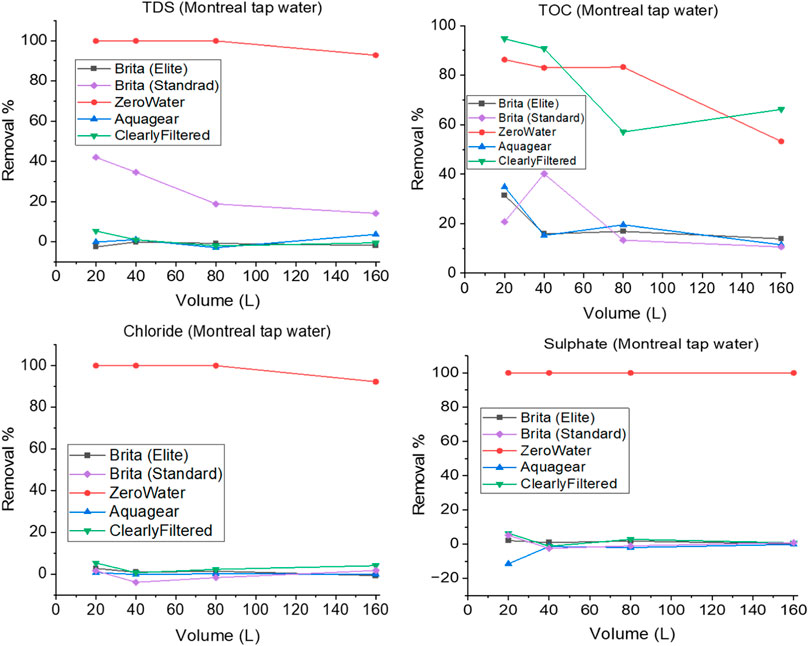
Figure 6. TDS, TOC, chloride, and sulfate removal % for selected POU filters using Montreal tap water.
Notably, chloride removal by ZeroWater showed differences between the two water sources (Figures 5, 6). For Montreal, ZeroWater, with an initial average concentration of 23 mg/L (n = 4), achieved a consistently high removal of about 100% up to 80 L (Figure 6), and this figure decreased slightly to approximately 92% after 160 L. However, in Saint-Donat tap water, which had a higher initial average chloride concentration (122 mg/L), the performance decreased to 48% after 80L, and after 160 L, the recorded removal was −7%, indicating that the filter had reached its saturation point for chloride and started to release previously exchanged chloride. On the other hand, sulfate removal percentages for ZeroWater were consistently high in both water sources, demonstrating almost 100% removal. Sulfate is known to exhibit a higher affinity than chloride for AIX resin, and the concentrations were less than chloride, especially at Saint Donat (Table 2). Therefore, monitoring chloride removal was a better predictor of PFAS removal.
ClearlyFiltered performance: ClearlyFiltered, as a PFAS reduction-certified product, had the second-best performance with ∼96% and ∼99% removal of total PFAS in Montreal and Saint-Donat tap water, respectively. This filter also had nearly 100% removal for PFOS + PFOA during the experiment for both tap waters. Specific details about the filter’s structure are not provided to explain the exact removal mechanisms. This filter is considered an advanced water filter with a higher price point and a significantly longer manufacturer-expected lifetime (approximately 2.5–5 times longer than ZeroWater). The filtration method employs a distinctive dual-technology approach to achieve this performance (carbon layers and proprietary composite layer).
As contaminants like PFAS pass through this filter constructed with Affinity® Filtration Technology, they undergo a complex pathway with multiple twists and turns, different from a direct trajectory, resulting in higher removal rates than simple filters, according to the manufacturer. This structure might also be the reason for its lower average flow rate compared to other evaluated POU filters (Supplementary Figure S2). ClearlyFiltered consistently maintained high removal percentages (near 100%) for most quantified PFAS in Saint-Donat water throughout the experiment for all chain lengths and functional groups (Figure 3). However, for PFBA, the volume-weighted average removal percentage over 160 L was 91%, while the specific removal after 160 L was 88%, attributed to the challenge in sorbing more hydrophilic short-chain PFAS in this adsorption-based filter. This filter also demonstrated high removal of PFAS in Montreal tap water but less than in Saint-Donat tap water when the filtration volume reached 160 L for PFCAs (C4-C7 &C9) and PFSAs (C4, C6, C8). The removal of PFBA changed from 100% to 45% after 160 L, probably due to the impacts of higher levels of TOC in Montreal tap water, which caused more competition from hydrophobic organic matter on adsorption sites, which also means ClearlyFiltered probably relies more on the hydrophobic effect of activated carbon for adsorption.
Other removal performances were also different using ClearlyFiltered in comparison with ZeroWater. The TOC volume-weighted average removal rate was 92%, with an average initial concentration of 0.8 mg/L in Saint-Donat influent water. However, in the case of Montreal tap water, which had a notably higher initial average TOC concentration of 2.2 mg/L, the removal dropped to 73%. This again suggests that the filter’s efficiency is influenced by the level of TOC in the water. In contrast with ZeroWater, ClearlyFiltered did not remove TDS, chloride, and sulfate, as shown in Figures 5, 6, which suggests that the filter does not rely on ion exchange as the primary removal mechanism.
Brita (Elite) performance: Brita filters are not NSF-53 certified for PFAS removal and lower performances were therefore expected. The Brita (Elite) filter showed a 36% removal for Σ75 PFAS after filtering 20 L of Saint-Donat tap water, which decreased to a mere 8% after filtering 160 L, with a volume-weighted average removal rate of 20%. Even though the TOC was higher in Montreal tap water, this filter exhibited better performance, achieving a 76% Σ75 PFAS removal after 20 L, which decreased to 39% after filtering 160 L, with a volume-weighted average removal rate of 48% after 160 L (vs. 20% in Saint-Donat). Volume-weighted average removal of the sum of PFOS and PFOA for this filter for Montreal tap water was relatively better (64%) than Saint-Donat tap water (29%). The type of PFAS present (Saint-Donat tap water includes higher levels of shorter chain PFAS with low AC affinity) is a potential explanation for this difference in performance.
These overall lower performances of the Brita (Elite) filter might be linked to its simpler filtration system (Supplementary Figure S3), which is not as effective in targeting PFAS contaminants. The main part of the structure of Brita that can help to reduce the PFAS is AC adsorption and hydrophobic interactions between the hydrophobic parts of the PFAS and the hydrophobic nature of the AC (Hakimabadi et al., 2023).
As can be seen in Figures 3, 4, the Brita (Elite) filter offered lower removal efficiencies of the shorter perfluorocarbon chains. As expected, the removal increased with the length of the PFAS chains. This filter also showed greater removal efficiencies for PFAS with sulfonate functional groups compared to carboxylate functional groups for both water sources, a common observation for AC sorption of PFAS. This difference can be attributed to the stronger negative inductive effect of the sulfonate functional group compared to carboxylate, primarily due to the presence of an additional oxygen atom in the sulfonate’s resonance structure (Park et al., 2020).
The 6:2 FTSA was also quantified (6.6–17.1 ng/L, mean = 14.0 ng/L) in all unfiltered Saint-Donat tap water samples (n = 16), which Brita (Elite) removed better than the total PFAS concentration, starting from 71% removal at 20 L to 20% after 160 L (with a volume-weighted average removal rate of 43%).
It must be mentioned that for Brita (Elite), the Saint-Donat raw waters were sampled over a period of about 2 weeks, which explains the higher influent variability (Figure 2A). This filter demonstrated low removal performance for TOC with a volume-weighted average removal rate of 19% and was ineffective in removing TDS, chloride, and sulfate. For this filter, the initial pH remained relatively stable throughout the filtration process for both water sources.
Brita (Standard) performance: The performance of the Brita (Standard) filter in Montreal tap water was also low, with a low volume-weighted average removal rate of 38% for Σ75 PFAS with about 42% for PFOS + PFOA. Therefore, it was not evaluated for Saint-Donat tap water. Similar to Brita (Elite), this filter also had better removal performance with increasing chain length. For instance, PFBA (C4) had a volume-weighted average removal rate of 25%, and this rate increased for PFNA (C9) to 46% during the experiment. Additionally, the removal efficiencies of PFSAs were greater than PFCAs but with less magnitude compared to the Brita (Elite) (Figure 4). The structure of this filter is presented in Supplementary Figure S4.
Aquagear performance: Aquagear is not NSF-certified for PFAS removal, but the manufacturer provides third-party performance data on its website. Aquagear’s performance also varied depending on the water source. It showed better removal efficiency for PFAS in Saint-Donat, with a volume-weighted average removal rate of 77% in comparison to Montreal tap water, with a volume-weighted average removal rate of 60%. The same removal trends were also observed for PFOS + PFOA, with volume-weighted average removals of 93% and 79% for Saint-Donat and Montreal tap waters, respectively. It means that despite Montreal tap water having lower PFAS levels, higher levels of other parameters in the water seemed to affect the PFAS adsorption on this filter, which is made from activated carbon and ion exchange media (Supplementary Figure S5). Aquagear also offered improved PFAS removals for longer chain lengths and sulfonated functional groups, as can be seen in Figures 3, 4. This removal trend suggests that activated carbon likely has a more important role in this filter, which is more effective in capturing longer-chain PFAS compounds.
The pH was not significantly impacted after the filtration of both tap waters. TOC removal by Aquagear was higher in Saint-Donat tap water, starting from 64% after 20 L and declining to 48% after 160 L with a volume-weighted average removal rate of 54% and in the case of Montreal tap water, ranging from 35% after 20 L to 11% after 160 L with a volume-weighted average of 20%.
This filter was also ineffective in removing TDS, chloride, and sulfate, suggesting that adsorption relies mostly on hydrophobicity. Notably, for sulfate, there was a significant release from 10 to 55 mg/L (removal rate −438%) after 20 L in Saint-Donat tap water. The negative removal rate continued although in smaller rates, until volume of 80 L (−16 removal % was recorded after reaching 80 L). A similar trend was also seen for Montreal tap water but with a smaller magnitude. After 20 L, the removal rate of sulfate was −11% but was insignificant after 160L. Further investigations would be needed to understand the exact source of this release as IX can be operated in sulfate mode. It is crucial to note that these sulfate leachings, though observed, remained below the EPA’s secondary maximum contaminant level (SMCL) of 250 mg/L (EPA, 2022).
Testing ZeroWater in domestic settings: As ZeroWater demonstrated the best performance in removing PFAS compared to other filters, further evaluation was done in a home setting using Montreal tap water to assess its PFAS removal performance under normal use. Since the house residents prefer to drink cold water, the filter was kept in the refrigerator (4°C) between use. The results are illustrated in Figure 7, where the volume-weighted average removal percentage for Σ75 PFAS reached 94%, while it was up to 100% for the laboratory test. Due to the low flow rate resulting from filter clogging, the residents faced challenges in reaching the 160-L volume needed to compare with lab tests. Consequently, data points for 160 L are missing in the home batch results, and the last volume recorded was 140 L. Globally, the home test provided performance data coherent with the laboratory test, given the numerous factors that differed in both tests.
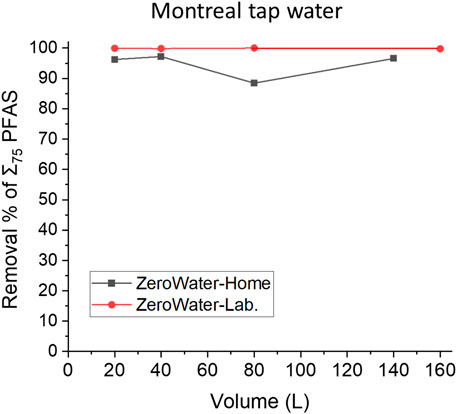
Figure 7. Comparison of Σ75PFAS removal in the home versus laboratory experiment using Montreal tap water.
4 Conclusion
The study compared the performance of various POU water filters, including Brita (Elite and Standard), ZeroWater, Aquagear, and ClearlyFiltered, in removing ambient PFAS concentrations from two different tap waters from Saint-Donat and Montreal, Canada. The findings revealed that the efficiency of the filters varied depending on their structure, water source, and the specific PFAS present.
The two NSF or WQA certified filters with advanced and multi-stage filtration systems, named ZeroWater and ClearlyFiltered filters, demonstrated the highest PFAS removal performances. Initial water quality, such as TOC, TDS, and anions levels can significantly influence PFAS filtration outcomes. Volume-weighted average removal of total PFAS over 160 L were 99%, 99%, 77%, and 20% for ZeroWater, ClearlyFiltered, Aquagear, and Brita (Elite), respectively, using Saint-Donat tap water. In the case of Montreal tap water with different water characteristics and lower total PFAS level, the volume-weighted average removal of PFAS were ∼100%, 96%, 60%, 48%, and 38% for ZeroWater, ClearlyFiltered, Aquagear, Brita (Elite) and Brita (Standard) respectively. Laboratory and home tests performed using ZeroWater filters provided similar performances.
ZeroWater demonstrated a noticeable pH drop after filtering 80 L in Saint-Donat tap water. Although desorption of organic matter and anions was noted during this event, PFAS removal was not impacted. Nevertheless, ZeroWater indicates that the acidic filtered water means the filter is exhausted and must be replaced. Aquagear also demonstrated an unusual sulfate release during the early sampling taken after 20 L, which needs further evaluation.
The findings of this study can provide guidance, especially for communities, particularly those residing in regions with notably elevated PFAS levels in tap water, as well as those relying on private wells as their source of drinking water facing PFAS contamination issues. This information aids them in selecting the most effective POU filter to protect them against PFAS that meets their specific water quality needs.
While this study aimed to assess the competitive PFAS removal efficiency of selected filters by considering some important water parameters, future investigations are required to evaluate their PFAS removal performance alongside a broader range of emerging contaminants such as pesticides, pharmaceuticals, or microplastics, especially for well-sourced tap water.
Furthermore, investigations on the environmental implications of POU filters are also important, including their material composition, disposal processes for used cartridges, and recyclability assessments to better clarify the overall sustainability of these filtration systems.
Data availability statement
The original contributions presented in the study are included in the article/Supplementary Material, further inquiries can be directed to the corresponding author.
Author contributions
TT: Conceptualization, Formal Analysis, Investigation, Methodology, Writing–original draft. QD: Investigation, Validation, Writing–review and editing, Methodology. BB: Conceptualization, Supervision, Writing–review and editing, Formal Analysis, Investigation, Validation. SS: Conceptualization, Funding acquisition, Methodology, Project administration, Supervision, Writing–review and editing.
Funding
The author(s) declare financial support was received for the research, authorship, and/or publication of this article. We want to thank the Natural Sciences and Engineering Research Council, the Canada Foundation for Innovation and PURE CREATE for funding this research.
Conflict of interest
The authors declare that the research was conducted in the absence of any commercial or financial relationships that could be construed as a potential conflict of interest.
Publisher’s note
All claims expressed in this article are solely those of the authors and do not necessarily represent those of their affiliated organizations, or those of the publisher, the editors and the reviewers. Any product that may be evaluated in this article, or claim that may be made by its manufacturer, is not guaranteed or endorsed by the publisher.
Supplementary material
The Supplementary Material for this article can be found online at: https://www.frontiersin.org/articles/10.3389/fenvc.2024.1376079/full#supplementary-material
References
Anumol, T., Clarke, B. O., Merel, S., and Snyder, S. A. (2015). Point-of-Use devices for attenuation of trace organic compounds in water. Journal-American Water Works Assoc. 107, E474–E485. doi:10.5942/jawwa.2015.107.0129
Belkouteb, N., Franke, V., Mccleaf, P., Köhler, S., and Ahrens, L. (2020). Removal of per-and polyfluoroalkyl substances (PFASs) in a full-scale drinking water treatment plant: long-term performance of granular activated carbon (GAC) and influence of flow-rate. Water Res. 182, 115913. doi:10.1016/j.watres.2020.115913
EPA (2019). Method 533: determination of per- and polyfluoroalkyl substances in drinking water by isotope dilution anion exchange solid phase extraction and liquid chromatography/tandem mass spectrometry. Available at: https://www.epa.gov/dwanalyticalmethods/method-533-determination-and-polyfluoroalkyl-substances-drinking-water-isotope (Accessed November 07, 2023).
EPA (2022). Sulfate in drinking water. Available at: https://archive.epa.gov/water/archive/web/html/sulfate.html#:∼:text=Sulfate%20in%20drinking%20water%20currently,i.e.%2C%20taste%20and%20odor (Accessed December 12, 2023).
EPA (2024). Per- and polyfluoroalkyl substances (PFAS)-Final PFAS national primary drinking water regulation. Available at: https://www.epa.gov/sdwa/and-polyfluoroalkyl-substances-pfas (Accessed May 6, 2024)
Goeury, K., Duy, S. V., Munoz, G., Prévost, M., and Sauvé, S. (2022). Assessment of automated off-line solid-phase extraction LC-MS/MS to monitor EPA priority endocrine disruptors in tap water, surface water, and wastewater. Talanta 241, 123216. doi:10.1016/j.talanta.2022.123216
Hakimabadi, S. G., Taylor, A., and Pham, A. L.-T. (2023). Factors affecting the adsorption of per-and polyfluoroalkyl substances (PFAS) by colloidal activated carbon. Water Res. 242, 120212. doi:10.1016/j.watres.2023.120212
He, A., Lu, Y., Chen, F., Li, F., Lv, K., Cao, H., et al. (2022). Exploring the origin of efficient adsorption of poly-and perfluoroalkyl substances in household point-of-use water purifiers: deep insights from a joint experimental and computational study. Sci. Total Environ. 831, 154988. doi:10.1016/j.scitotenv.2022.154988
HEALTH-CANADA (2023) Draft objective for per- and polyfluoroalkyl substances in Canadian drinking water: overview.
Herkert, N. J., Merrill, J., Peters, C., Bollinger, D., Zhang, S., Hoffman, K., et al. (2020). Assessing the effectiveness of point-of-use residential drinking water filters for perfluoroalkyl substances (PFASs). Environ. Sci. Technol. Lett. 7, 178–184. doi:10.1021/acs.estlett.0c00004
Kaboré, H. A., Duy, S. V., Munoz, G., Méité, L., Desrosiers, M., Liu, J., et al. (2018). Worldwide drinking water occurrence and levels of newly-identified perfluoroalkyl and polyfluoroalkyl substances. Sci. total Environ. 616, 1089–1100. doi:10.1016/j.scitotenv.2017.10.210
Karamat, A., Tehrani, R., Foster, G. D., and VAN Aken, B. (2023). Plant responses to per-and polyfluoroalkyl substances (PFAS): a molecular perspective. Int. J. Phytoremediation 26, 219–227. doi:10.1080/15226514.2023.2232874
Li, D., Lee, C.-S., Zhang, Y., Das, R., Akter, F., Venkatesan, A. K., et al. (2023). Efficient removal of short-chain and long-chain PFAS by cationic nanocellulose. J. Mater. Chem. A 11, 9868–9883. doi:10.1039/d3ta01851b
Liu, Z., Papineau, I., Mohseni, M., Peldszus, S., Bérubé, P. R., Sauvé, S., et al. (2021). Operating bicarbonate-form versus chloride-form ion exchange resins without regeneration for natural organic matter removal. ACS ES&T Water 1, 1456–1463. doi:10.1021/acsestwater.1c00040
Muir, D., and Sverko, E. (2006). Analytical methods for PCBs and organochlorine pesticides in environmental monitoring and surveillance: a critical appraisal. Anal. Bioanal. Chem. 386, 769–789. doi:10.1007/s00216-006-0765-y
Mulhern, R., Bynum, N., Liyanapatirana, C., Destefano, N. J., Knappe, D. R., and Macdonald Gibson, J. (2021). Longitudinal assessment of point-of-use carbon filters for removal of per-and polyfluoroalkyl substances from private well water. AWWA Water Sci. 3, e1262. doi:10.1002/aws2.1262
Munoz, G., Liu, M., Duy, S. V., Liu, J., and Sauvé, S. (2023). Target and nontarget screening of PFAS in drinking water for a large-scale survey of urban and rural communities in Québec, Canada. Water Res. 233, 119750. doi:10.1016/j.watres.2023.119750
Park, M., Daniels, K. D., Wu, S., Ziska, A. D., and Snyder, S. A. (2020). Magnetic ion-exchange (MIEX) resin for perfluorinated alkylsubstance (PFAS) removal in groundwater: roles of atomic charges for adsorption. Water Res. 181, 115897. doi:10.1016/j.watres.2020.115897
Patterson, C., Burkhardt, J., Schupp, D., Krishnan, E. R., Dyment, S., Merritt, S., et al. (2019). Effectiveness of point-of-use/point-of-entry systems to remove per-and polyfluoroalkyl substances from drinking water. AWWA water Sci. 1, 11311–e1212. doi:10.1002/aws2.1131
Shoemaker, J., and Tettenhorst, D. (2018) EPA Method 537.1: determination of selected per-and polyfluorinated alkyl substances in drinking water by solid phase extraction and liquid chromatography/tandem mass spectrometry (LC/MS/MS). Washington, DC: National Center for Environmental Assessment.
Sosnowska, A., Bulawska, N., Kowalska, D., and Puzyn, T. (2023). Towards higher scientific validity and regulatory acceptance of predictive models for PFAS. Green Chem. 25, 1261–1275. doi:10.1039/d2gc04341f
Teymoorian, T., Munoz, G., Vo Duy, S., Liu, J., and Sauvé, S. (2023). Tracking PFAS in drinking water: a review of analytical methods and worldwide occurrence trends in tap water and bottled water. ACS ES&T Water 3, 246–261. doi:10.1021/acsestwater.2c00387
Teymourian, T., Teymoorian, T., Kowsari, E., and Ramakrishna, S. (2021). A review of emerging PFAS contaminants: sources, fate, health risks, and a comprehensive assortment of recent sorbents for PFAS treatment by evaluating their mechanism. Res. Chem. Intermed. 47, 4879–4914. doi:10.1007/s11164-021-04603-7
Keywords: PFAS removal, point-of-use filters, drinking water, water quality, water treatment, environmental pollution, LC-HRMS
Citation: Teymoorian T, Dinh QT, Barbeau B and Sauvé S (2024) Performance of pitcher-type POU filters for the removal of 75 PFAS from drinking water: comparing different water sources. Front. Environ. Chem. 5:1376079. doi: 10.3389/fenvc.2024.1376079
Received: 24 January 2024; Accepted: 22 April 2024;
Published: 04 June 2024.
Edited by:
Gaurav Pant, Graphic Era University, IndiaReviewed by:
Krishna Kumar Pandey, Banaras Hindu University, IndiaAjay Harit, Mahatma Gandhi University, India
Copyright © 2024 Teymoorian, Dinh, Barbeau and Sauvé. This is an open-access article distributed under the terms of the Creative Commons Attribution License (CC BY). The use, distribution or reproduction in other forums is permitted, provided the original author(s) and the copyright owner(s) are credited and that the original publication in this journal is cited, in accordance with accepted academic practice. No use, distribution or reproduction is permitted which does not comply with these terms.
*Correspondence: Sébastien Sauvé, c2ViYXN0aWVuLnNhdXZlQHVtb250cmVhbC5jYQ==