- EcotoQ, Institut National de la Recherche Scientifique, Centre Eau Terre Environnement, Québec city, QC, Canada
Metal bioavailability in solution is mostly driven by two factors: complexation and competition. The first factor, complexation, contributes to decrease the overall reactivity of the metal by reducing the activity of the free metal ion, which is known as the common denominator of metal reactions involving either dissolved ligands or surface functional groups (abiotic or biotic). Ubiquitous in natural ecosystems, natural organic matter is, for several metals, the most important metal complexing ligand. The second factor, competition, contributes to decrease the availability of biotic ligands involved in the membrane transport of metals from the bulk solution to the intracellular medium. In freshwater systems, proton and hardness cation concentrations are the main parameters potentially modulating metal bioavailability. The above reflects the current accepted paradigm. In this paper, two knowledge gaps are identified: i) the role of natural organic matter other than metal complexation that may lead to an increase in metal bioavailability; and ii) the effects of multiple metals other than competition that may trigger biological feedback mechanisms which may, in turn, alter biotic ligand binding properties. More research efforts are needed to decipher the extent of these overlooked potential effects and to improve the predictability of metal bioavailability.
1 Introduction
One of the major breakthroughs in aquatic environmental toxicology of trace elements was the discovery of the predominant role of the free metal ion in our ability to predict metal bioavailability. More than 80 years ago, in a literature review, Nielsen and Massey (1940) stated: “The toxicity of silver is due to the presence in solution of free silver cations”. Back then, most reports like this one remained anecdotal in nature. The first hard evidence obtained that metal complexation decreased toxicity was through the work of Steeman Nielsen and Wium-Andersen (1970) who suggested that the free copper ion was a better predictor of toxicity to freshwater algae than dissolved copper. At the time, the interest in copper toxicity was fuelled by the inadvertent presence of copper in 14C ampoules used to determine carbon fixation rates by phytoplankton. Copper being toxic to algae, its presence generated a bias in productivity measurements. This observation triggered a spur of studies on metal bioavailability (Gächter et al., 1973; Manahan and Smith, 1973). Using thermodynamic equilibrium constants to calculate the metal ion concentrations, Sunda and Huntsman, (1976) presented one of the first graphs showing the link between free metal ion concentration and uptake/toxicity: in this pioneering work, copper uptake in the estuarine diatom Thalassiosira pseudonana and its subsequent effect on growth was related to the free cupric ion concentration in the exposure solution. Around that time, our capacity to predict metal complexation through thermodynamic modelling was developed, first by Perrin and Sayce (1967), followed by many others [e.g., see Figure 1 of Di Bonito et al. (2018)]. These models facilitated the search for links between the chemical speciation of a metal (the distribution of dissolved chemical species of a given metal and redox state) and its uptake/toxicity. Such models allowed Anderson et al. (1978) to demonstrate, this time from a nutritional perspective, that the growth of the coastal diatom Thalassiosira weissflogii was dependent on the free zinc ion activity rather than the total zinc concentration. Many others (e.g., Allen et al., 1980) then followed the path laid down by these pioneers and developed a large body of evidence over time supporting the early observations. The term “free ion-activity model” only appeared much later (Morel, 1983). As opposed to sea water, the ionic composition of fresh water can vary significantly depending on the nature of the bedrock of a given watershed. This led some scientists to also consider speciation, not only in the water column, but also at the interface between the bulk solution and the binding sites at the surface of aquatic organisms that were involved in metal uptake. The gill surface interaction model presented by Pagenkopf (1983) laid down the foundation of what is now known as the Biotic Ligand Model or BLM (Di Toro et al., 2001). This approach took into consideration both the role of metal complexation by dissolved ligands and competition among major cations (mainly Ca2+, Mg2+ and H+) and metals for binding with biotic ligands (Figure 1A).
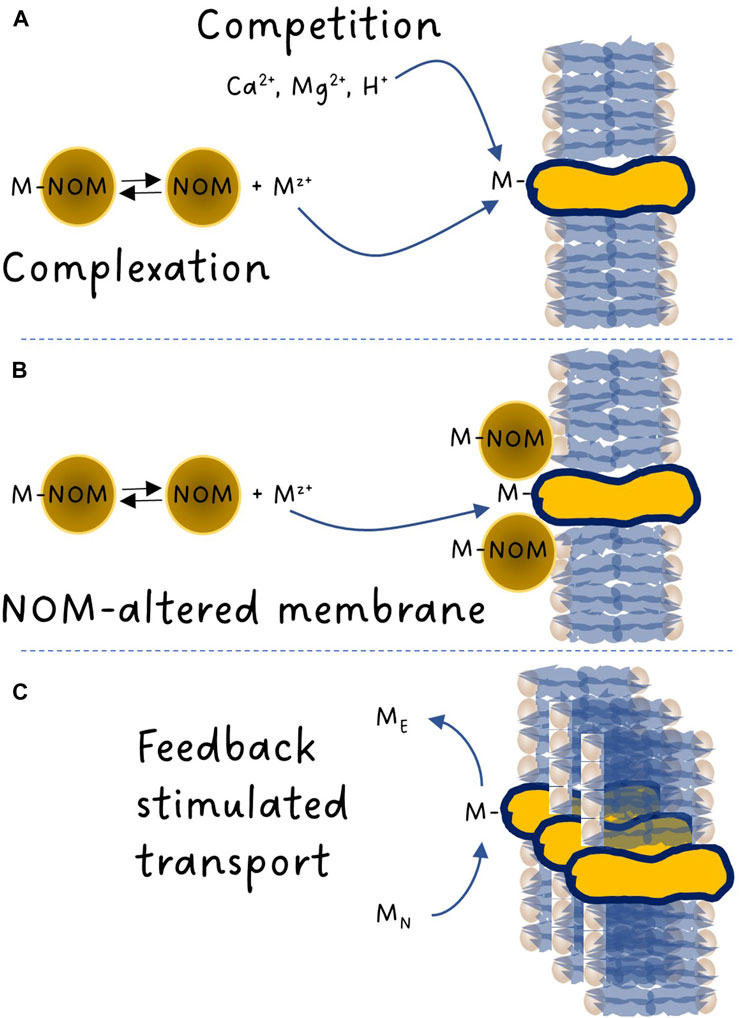
FIGURE 1. Schematic overview of the metal binding reactions involved with membrane transporters. (A) Metal complexation with natural organic matter (NOM) lowering the free metal-ion activity and competitive interactions between the metal, protons, calcium and magnesium ions. (B) NOM adsorption to membranes and alteration of membrane properties. (C) Competitive interactions between an essential (ME) and a nonessential metal (MN) resulting in a cellular deficiency in ME counter-balanced by the generation of additional transport sites by the cell through a feedback response.
The limits of these conceptual models have been described thoroughly by Campbell (1995) and exceptions were documented. These have been detailed in several publications (Zhao et al., 2016; Campbell et al., 2022) and can succinctly be broken down into three categories: i) The metal of interest forms lipophilic complexes that bypass ion transporters by crossing plasma membranes by simple passive diffusion (e.g., see Boullemant et al. (2011) and references therein for examples); ii) The metal binding ligand is assimilable and leads to “piggy-back” transport in which the metal is inadvertently taken up (e.g., Errécalde and Campbell, 2000; Fortin and Campbell, 2001); or iii) The metal internalization step is not the rate-determining step due either to very high uptake fluxes resulting in a diffusion limitation at the boundary layer of the membrane (e.g., Fortin and Campbell, 2000) or to slow dissociation kinetics (e.g., Hudson, 1998; Town and van Leeuwen, 2022).
2 Effects of ligands other than metal complexation
Aside from the notable exceptions mentioned above, there is an overwhelming literature available showing that metal complexation results in a decrease in overall metal bioavailability. This tenet has become largely accepted with time, particularly for simple monomeric ligands. These ligands can be of natural origin (e.g., chloride ions; amino acids) or synthetic in nature (e.g., EDTA or ethylenediaminetetraacetic acid). For many metals, the complex stability constants with these ligands are available in thermodynamic data libraries (Hummel et al., 2019) which allow anyone to calculate with confidence the metal species distribution in a given exposure medium. There are, however, two major remaining challenges in this area: i) Some thermodynamic data are missing for less encountered metals, many of which are listed as strategic and critical metals (Jabłońska-Czapla and Grygoyć, 2020; Tipping and Filella, 2020), and these data gaps hinder our capacity to examine links between these metals’ speciation and their bioavailability; and ii) The difficulty in determining (either analytically or through predictive modelling) the free metal ion concentration when the metal is in the presence of heterogeneous polyfunctional natural organic matter (NOM; also often referred to as humic and fulvic acids). Regarding the latter challenge, the protective role of NOM on metal bioavailability is usually tested by adding increasing amounts of a NOM to solutions containing a constant total metal concentration, resulting in a decrease in free metal ion concentration and, thus, a decrease in metal uptake and toxicity. This approach offers the advantage that the actual extent of the metal complexation by NOM does not need to be accurately known and a decrease in observed uptake and toxicity can be de facto attributed to a protective effect of NOM, presumably through metal binding. However, to be more quantitative, the extent of metal complexation needs to be determined and this remains an analytical challenge to this day. When an analytical technique is used to quantify the NOM complexation, the metal uptake/toxicity as a function of free metal can be compared with and without NOM. In these situations, occasionally, a greater-than-expected uptake/toxicity is observed in the presence of NOM. For example, such intriguing results for unicellular algae have been reported for Al, Cu, La, Pb, Pt and Zn (Parent et al., 1996; Lamelas et al., 2009; Worms et al., 2015; Hourtané et al., 2022; Barber-Lluch et al., 2023; Price et al., 2023; Zilber et al., 2024). Another potential aspect that has been overlooked is the direct effects of NOM on organisms (Campbell et al., 1997; Glover and Wood, 2005; Bittner et al., 2012) and how these could affect metal transport.
The mechanism(s) by which NOM can alter metal uptake fluxes remains to be fully elucidated (Figure 1B). Although the overall trend of NOM being a toxicity modifying factor for which an increasing presence of NOM will normally reduce bioavailability is rarely contested, knowledge of the exact conditions that may exceptionally lead to a higher toxic impact than anticipated is needed to improve metal risk assessment, especially in light of the ubiquitous importance of NOM in freshwater systems and the current global trend of increasing NOM inputs from terrestrial systems to surface waters (Monteith et al., 2007; Rodríguez-Cardona et al., 2023).
3 Effects of multiple metals other than competition
The other main building block of the BLM is the protective role of competing ions. For positively charged metals, these are typically hardness cations (Ca2+, Mg2+) but also protons (H+). From a chemist’s perspective, any cation in solution can potentially compete with a given metal. The empirical evidence that hardness cations are the main competing ions resulting in a protective effect of metal uptake and toxicity is most likely due to the fact that these cations are typically present at high background concentrations. Their high concentrations can thus overcome their low affinity for binding sites, while trace elements typically have low aqueous concentrations but a high affinity for biotic ligands. The same principle applies to protons. At acidic pH values, hydronium ions reach concentrations that can outcompete trace metals for biotic ligands. For example, Laderriere et al. (2021) observed that below a pH of 6, the accumulation of Cd, Cu and Ni by river biofilms decreased sharply. Note here that pH has dual and opposing effects (Campbell, 1995). A decrease in pH, for several metals (e.g., Cu, Pb, UO2), will result in an increase in the proportion of free metal in solution, thereby increasing overall bioavailability. On the other hand, a decrease in ambient pH also means more competition for biological binding sites by protons, which reduces metal uptake and toxicity. Which of the two variables will predominate is metal- and species-dependent and requires meticulous scrutiny, but, in general, at acidic pHs (<6), competition at the biological surface becomes more important than the pH-induced increase in the free metal ion concentration. Other major cations such as Na and K, two monovalent ions, are rarely involved in significant competitive effect unless the metal of interest is known to react with the transport sites of one of these cations [e.g., K+/Tl+ in algae (Hassler et al., 2007); Na+/Ag+ in fish (Morgan et al., 1997)].
From a risk assessment perspective, it is clear that hardness cations and pH are toxicity modifying factors that can be quantified effectively. The remaining challenges are elsewhere. We currently assume that the only mechanism involved is competition (Figure 1A). However, several papers have identified changes in metal bioavailability with pH that could not be explained by changes in complexation and competition (Markich et al., 2000; Slaveykova and Wilkinson, 2003; Fortin et al., 2007; François et al., 2007; Crémazy et al., 2013). Similar questions have been raised regarding competing reactions among metals. Indeed, most of the research in the field has been performed using single metal exposures, while in a typical real-life exposure scenario (e.g., effluents from metal extraction and processing activities) a large array of elements is present. Whether two trace elements compete for binding to a given biotic ligand will depend not only on their respective concentrations, but also on the nature of the membrane transporters involved and their affinities for each metal. Many metals are essential for growth and cellular function. It follows that for these elements, acquisition strategies exist, and cells have the capacity to synthesize essential metal transporters to meet cellular requirements. No such transporters should exist for nonessential metals but they can sometimes use transporters that were designed for essential elements. Nevertheless, both essential and nonessential metals can be toxic if present at concentrations exceeding the organism’s capacity to regulate internal metal concentrations. If the ambient concentration of an essential metal ME is high enough to exert a toxic effect, the presence of a nonessential metal MN that can bind to the same metal transporter as ME is expected to contribute to decreasing the binding of essential metal ME, thereby resulting in a protective effect. What has been less considered so far is what happens when [MN] >> [ME]. In such a scenario, the cellular content of essential metal ME may become insufficient for metabolic needs. This situation typically triggers a feedback response from the organism to synthesize more metal ME transporters, further exacerbating the uptake and effect of metal MN (Figure 1C). On the contrary, if [ME] >> [MN], the cellular requirement in metal ME is largely met and the cell may opt to downregulate metal ME transporters, resulting in a decrease in the uptake of metal MN. When such physiological feedback mechanisms are operative, the organism’s response cannot be adequately predicted by mere competitive effects among metals. In other words, the current BLM approach only considers that organisms react with metals and does not consider that organisms react to metals. Examples of such interactions remain rare, mostly because they are poorly investigated. Again, studies performed on unicellular algae have identified such feedback interactions for several metals (Sunda and Huntsman, 2000; Lane et al., 2008; Lavoie et al., 2012; Sunda, 2012; Kochoni et al., 2022).
From a regulatory perspective, an empirical approach is often preferred over complex mechanistic models. The current popularity of multiple linear relationships over the BLM is a good example (Mebane, 2023). The above considerations may thus seem mostly to be of an academic nature. However, the development of robust mechanistic approaches allows for the confident use of simpler empirical models. There is thus a need to better understand the intricacies of metal trafficking at the organism interface (whether in air, water or soils) and within the organism to shed light on how cells react to metals. Among the challenges ahead, we need to identify the ion transporters used by non-essential metals, in order to anticipate which essential metals may be affected by the growing presence of nonessential metals.
4 Conclusion
Research over the past 50 years has mainly focused on the impact of high-volume base metals such as Fe, Zn, Cu, Ni and elements such as Cd and As that are present in mineral deposits. The growing world demand for rare earth elements and other technology critical elements reveals a large ecotoxicological data gap for these elements (Dang et al., 2021; Batley and Campbell, 2022). Moreover, further scrutiny of the role played by natural organic matter other than metal complexation and the effects of multiple metals other than competition are needed to provide the scientific knowledge required to build the next-generation of metal uptake/toxicity predictive models.
Data availability statement
The original contributions presented in the study are included in the article/supplementary material, further inquiries can be directed to the corresponding author.
Author contributions
CF: Writing–original draft, Writing–review and editing.
Funding
The author(s) declare that financial support was received for the research, authorship, and/or publication of this article. This work was partially supported by an NSERC Discovery Grant (grant no. RGPIN-2019-06823).
Acknowledgments
The author gratefully acknowledges the comments provided by Peter Campbell, Océane Hourtané and Sébastien Sauvé on an earlier version of the manuscript.
Conflict of interest
The author declares that the research was conducted in the absence of any commercial or financial relationships that could be construed as a potential conflict of interest.
The author(s) declared that they were an editorial board member of Frontiers, at the time of submission. This had no impact on the peer review process and the final decision.
Publisher’s note
All claims expressed in this article are solely those of the authors and do not necessarily represent those of their affiliated organizations, or those of the publisher, the editors and the reviewers. Any product that may be evaluated in this article, or claim that may be made by its manufacturer, is not guaranteed or endorsed by the publisher.
References
Allen, H. E., Hall, R. H., and Brisbin, T. D. (1980). Metal speciation - effects on aquatic toxicity. Environ. Sci. Technol. 14 (4), 441–443. doi:10.1021/es60164a002
Anderson, M. A., Morel, F. M. M., and Guillard, R. R. L (1978). Growth limitation of a coastal diatom by low zinc ion activity. Nature 276 (5683), 70–71. doi:10.1038/276070a0
Barber-Lluch, E., Nieto-Cid, M., Santos-Echeandia, J., and Sanchez-Marin, P. (2023). Effect of dissolved organic matter on copper bioavailability to a coastal dinoflagellate at environmentally relevant concentrations. Sci. Total Environ. 901, 165989. doi:10.1016/j.scitotenv.2023.165989
Batley, G. E., and Campbell, P. G. C. (2022). Metal contaminants of emerging concern in aquatic systems. Environ. Chem. 19 (1), 23–40. doi:10.1071/En22030
Bittner, M., Saul, N., and Steinberg, C. E. (2012). Antiandrogenic activity of humic substances. Sci. Total Environ. 432, 93–96. doi:10.1016/j.scitotenv.2012.05.056
Boullemant, A., Le Faucheur, S., Fortin, C., and Campbell, P. G. C. (2011). Uptake of lipophilic cadmium complexes by three green algae: influence of humic acid and its pH dependence. J. Phycol. 47 (4), 784–791. doi:10.1111/j.1529-8817.2011.01013.x
Campbell, P. G. C. (1995). “Interactions between trace metals and aquatic organisms: a critique of the free-ion activity model,” in Metal speciation and bioavailability in aquatic systems. Editors A. Tessier, and D. R. Turner (New York, NY, USA: John Wiley and Sons), 42, 45–102. doi:10.1139/f85-251
Campbell, P. G. C., Twiss, M. R., and Wilkinson, K. J. (1997). Accumulation of natural organic matter on the surfaces of living cells: implications for the interaction of toxic solutes with aquatic biota. Can. J. Fish. Aquat. Sci. 54 (11), 2543–2554. doi:10.1139/f97-161
Campbell, P. G. C., Welbourn, P. M., and Metcalfe, C. D. (2022) Metals and metalloids. In . (Eds PGC Campbell, PV hodson, PM welbourn, DA wright pp. 170–273. (Cambridge University Press: Cambridge) doi:10.1017/9781108819732.009
Crémazy, A., Campbell, P. G. C., and Fortin, C. (2013). The biotic ligand model can successfully predict the uptake of a trivalent ion by a unicellular alga below pH 6.50 but not above: possible role of hydroxo-species. Environ. Sci. Technol. 47 (5), 2408–2415. doi:10.1021/es3038388
Dang, D. H., Filella, M., and Omanović, D. (2021). Technology-critical elements: an emerging and vital resource that requires more in-depth investigation. Arch. Environ. Contam. Toxicol. 81 (4), 517–520. doi:10.1007/s00244-021-00892-6
Di Bonito, M., Lofts, S., and Groenenberg, J. E. (2018). “Models of geochemical speciation: structure and applications,” in Environmental geochemistry. Editors B. De Vivo, B. HE, and A. Lima Second Edition (China: Elsevier), 237–305. doi:10.1016/B978-0-444-63763-5.00012-4
Di Toro, D. M., Allen, H. E., Bergman, H. L., Meyer, J. S., Paquin, P. R., and Santore, R. C. (2001). Biotic ligand model of the acute toxicity of metals. 1. Technical basis. Environ. Toxicol. Chem. 20 (10), 2383–2396. doi:10.1002/etc.5620201034
Errécalde, O., and Campbell, P. G. C. (2000). Cadmium and zinc bioavailability to Selenastrum capricornutum (Chlorophyceae): accidental metal uptake and toxicity in the presence of citrate. J. Phycol. 36 (3), 473–483. doi:10.1046/j.1529-8817.2000.99103.x
Fortin, C., and Campbell, P. G. C. (2000). Silver uptake by the green alga Chlamydomonas reinhardtii in relation to chemical speciation: influence of chloride. Environ. Toxicol. Chem. 19 (11), 2769–2778. doi:10.1002/etc.5620191123
Fortin, C., and Campbell, P. G. C. (2001). Thiosulfate enhances silver uptake by a green alga: role of anion transporters in metal uptake. Environ. Sci. Technol. 35 (11), 2214–2218. doi:10.1021/es0017965
Fortin, C., Denison, F. H., and Garnier-Laplace, J. (2007). Metal-phytoplankton interactions: modeling the effect of competing ions (H+, Ca2+, and Mg2+) on uranium uptake. Environ. Toxicol. Chem. 26 (2), 242–248. doi:10.1897/06-298r.1
François, L., Fortin, C., and Campbell, P. G. C. (2007). pH modulates transport rates of manganese and cadmium in the green alga Chlamydomonas reinhardtii through non-competitive interactions: implications for an algal BLM. Aquat. Toxicol. 84 (2), 123–132. doi:10.1016/j.aquatox.2007.02.019
Gächter, R., Lum-Shue-Chan, K., and Chau, Y. K. (1973). Complexing capacity of the nutrient medium and its relation to inhibition of algal photosynthesis by copper. Schweiz Z Hydrol. 35 (2), 252–261. doi:10.1007/BF02502921
Glover, C. N., and Wood, C. M. (2005). The disruption of Daphnia magna sodium metabolism by humic substances: mechanism of action and effect of humic substance source. Physiol. Biochem. Zool. 78 (6), 1005–1016. doi:10.1086/432858
Hassler, C. S., Chafin, R. D., Klinger, M. B., and Twiss, M. R. (2007). Application of the biotic ligand model to explain potassium interaction with thallium uptake and toxicity to plankton. Environ. Toxicol. Chem. 26 (6), 1139–1145. doi:10.1897/06-315r.1
Hourtané, O., Rioux, G., Campbell, P. G. C., and Fortin, C. (2022). Algal bioaccumulation and toxicity of platinum are increased in the presence of humic acids. Environ. Chem. 19 (4), 144–155. doi:10.1071/EN22037
Hudson, R. J. M. (1998). Which aqueous species control the rates of trace metal uptake by aquatic biota? Observations and predictions of non-equilibrium effects. Sci. Total Environ. 219, 95–115. doi:10.1016/S0048-9697(98)00230-7
Hummel, W., Filella, M., and Rowland, D. (2019). Where to find equilibrium constants? Sci. Total Environ. 692, 49–59. doi:10.1016/j.scitotenv.2019.07.161
Jabłońska-Czapla, M., and Grygoyć, K. (2020). Speciation and fractionation of less-studied technology-critical elements (Nb, Ta, Ga, In, Ge, Tl, Te): a review. Pol. J. Environ. Stud. 30 (2), 1477–1486. doi:10.15244/pjoes/127281
Kochoni, E., Doose, C., Gonzalez, P., and Fortin, C. (2022). Role of iron in gene expression and in the modulation of copper uptake in a freshwater alga: insights on Cu and Fe assimilation pathways. Environ. Pollut. 305, 119311. doi:10.1016/j.envpol.2022.119311
Laderriere, V., Le Faucheur, S., and Fortin, C. (2021). Exploring the role of water chemistry on metal accumulation in biofilms from streams in mining areas. Sci. Total Environ. 784, 146986. doi:10.1016/j.scitotenv.2021.146986
Lamelas, C., Pinheiro, J. P., and Slaveykova, V. I. (2009). Effect of humic acid on Cd(II), Cu(II), and Pb(II) uptake by freshwater algae: kinetic and cell wall speciation considerations. Environ. Sci. Technol. 43 (3), 730–735. doi:10.1021/Es802557r
Lane, E. S., Jang, K., Cullen, J. T., and Maldonado, M. T. (2008). The interaction between inorganic iron and cadmium uptake in the marine diatom Thalassiosira oceanica. Limnol. Oceanogr. 53 (5), 1784–1789. doi:10.4319/lo.2008.53.5.1784
Lavoie, M., Campbell, P. G. C., and Fortin, C. (2012). Extending the biotic ligand model to account for positive and negative feedback interactions between cadmium and zinc in a freshwater alga. Environ. Sci. Technol. 46 (21), 12129–12136. doi:10.1021/Es302512r
Manahan, S. E., and Smith, M. J. (1973). Copper micronutrient requirement for algae. Environ. Sci. Technol. 7 (9), 829–833. doi:10.1021/es60081a013
Markich, S. J., Brown, P. L., Jeffree, R. A., and Lim, R. P. (2000). Valve movement responses of Velesunio angasi (Bivalvia: hyriidae) to manganese and uranium: an exception to the free ion activity model. Aquat. Toxicol. 51 (2), 155–175. doi:10.1016/s0166-445x(00)00114-4
Mebane, C. A. (2023). Bioavailability and toxicity models of copper to freshwater life: the state of regulatory science. Environ. Toxicol. Chem. 42, 2529–2563. doi:10.1002/etc.5736
Monteith, D. T., Stoddard, J. L., Evans, C. D., de Wit, H. A., Forsius, M., Hogasen, T., et al. (2007). Dissolved organic carbon trends resulting from changes in atmospheric deposition chemistry. Nature 450 (7169), 537–540. doi:10.1038/nature06316
Morgan, I. J., Henry, R. P., and Wood, C. M. (1997). The mechanism of acute silver nitrate toxicity in freshwater rainbow trout (Oncorhynchus mykiss) is inhibition of gill Na+ and Cl- transport. Aquat. Toxicol. 38 (1-3), 145–163. doi:10.1016/S0166-445X(96)00835-1
Nielsen, L. W., and Massey, L. M. (1940). “Silver as a fungicide,” in Silver in industry'. Editor L. Addicks (New York: Reinhold Publishing Corp), 431–450.
Pagenkopf, G. K. (1983). Gill surface interaction model for trace-metal toxicity to fishes: role of complexation, pH and water hardness. Environ. Sci. Technol. 17 (6), 342–347. doi:10.1021/es00112a007
Parent, L., Twiss, M. R., and Campbell, P. G. C. (1996). Influences of natural dissolved organic matter on the interaction of aluminum with the microalga Chlorella: a test of the free-ion model of trace metal toxicity. Environ. Sci. Technol. 30 (5), 1713–1720. doi:10.1021/ES950718S
Perrin, D. D., and Sayce, I. G. (1967). Computer calculation of equilibrium concentrations in mixtures of metal ions and complexing species. Talanta 14 7 (7), 833–842. doi:10.1016/0039-9140(67)80105-x
Price, G. A. V., Stauber, J. L., Jolley, D. F., Koppel, D. J., Van Genderen, E. J., Ryan, A. C., et al. (2023). Natural organic matter source, concentration, and pH influences the toxicity of zinc to a freshwater microalga. Environ. Pollut. 318, 120797. doi:10.1016/j.envpol.2022.120797
Rodríguez-Cardona, B. M., Houle, D., Couture, S., Lapierre, J.-F., and del Giorgio, P. A. (2023). Long-term trends in carbon and color signal uneven browning and terrestrialization of northern lakes. Commun. Earth Environ. 4 (1), 338. doi:10.1038/s43247-023-00999-9
Slaveykova, V. I., and Wilkinson, K. J. (2003). Effect of pH on Pb biouptake by the freshwater alga Chlorella kesslerii. Chlorella Kesslerii. Environ. Chem. Lett. 1 (3), 185–189. doi:10.1007/s10311-003-0041-8
Steeman Nielsen, E., and Wium-Andersen, S. (1970). Copper ions as poison in the sea and in freshwater. Mar. Biol. 6 (2), 93–97. doi:10.1007/BF00347237
Sunda, W. (2012). Feedback interactions between trace metal nutrients and phytoplankton in the ocean. Front. Microbiol. 3, 204. doi:10.3389/fmicb.2012.00204
Sunda, W. G., and Huntsman, S. A. (2000). Effect of Zn, Mn, and Fe on Cd accumulation in phytoplankton: implications for oceanic Cd cycling. Limnol. Oceanogr. 45 (7), 1501–1516. doi:10.4319/lo.2000.45.7.1501
Tipping, E., and Filella, M. (2020). Estimation of WHAM7 constants for GaIII, InIII, SbIII and BiIII from linear free energy relationships, and speciation calculations for natural waters. Environ. Chem. 17 (2), 140–147. doi:10.1071/EN19194
Town, R. M., and van Leeuwen, H. P. (2022). Chemodynamic features of nickel(II) and its complexes: implications for bioavailability in freshwaters. Ecotoxicol. Environ. Saf. 241, 113840. doi:10.1016/j.ecoenv.2022.113840
Worms, I. A. M., Slaveykova, V. I., and Wilkinson, K. J. (2015). Lead bioavailability to freshwater microalgae in the presence of dissolved organic matter: contrasting effect of model humic substances and marsh water fractions obtained by ultrafiltration. Aquat. Geochem 21 (2-4), 217–230. doi:10.1007/s10498-015-9256-0
Zhao, C. M., Campbell, P. G. C., and Wilkinson, K. J. (2016). When are metal complexes bioavailable? Environ. Chem. 13 (3), 425–433. doi:10.1071/En15205
Keywords: biotic ligand model, free-ion activity model, metal speciation, metal bioavailability, predictive modeling, metal uptake, metal toxicity
Citation: Fortin C (2024) Metal bioavailability in aquatic systems— beyond complexation and competition. Front. Environ. Chem. 5:1345484. doi: 10.3389/fenvc.2024.1345484
Received: 27 November 2023; Accepted: 13 February 2024;
Published: 23 February 2024.
Edited by:
Ying Ge, Nanjing Agricultural University, ChinaReviewed by:
Qiao-Guo Tan, Xiamen University, ChinaCopyright © 2024 Fortin. This is an open-access article distributed under the terms of the Creative Commons Attribution License (CC BY). The use, distribution or reproduction in other forums is permitted, provided the original author(s) and the copyright owner(s) are credited and that the original publication in this journal is cited, in accordance with accepted academic practice. No use, distribution or reproduction is permitted which does not comply with these terms.
*Correspondence: Claude Fortin, Y2xhdWRlLmZvcnRpbkBldGUuaW5ycy5jYQ==