- 1College of Science and Engineering, James Cook University Townsville, Townsville, QLD Australia
- 2Australian Institute of Marine Science (AIMS), Townsville, QLD, Australia
Microplastics (MPs) have become ubiquitous in the marine environment, and are likely ingested by a broad cross-section of marine life. The extent to which marine organisms ingest MPs is uncertain due to limitations in analytical methods. Effective identification and analysis of ingested MPs is a precursor to understand their impact on marine organisms and their human consumers. This is particularly challenging for crustaceans, due to the chitin present in their exoskeleton and digestive systems, which is resistant to chemical degradation. This study presents a novel application that can efficiently break down the stable organic tissue of banana prawns (Penaeus merguiensis), and subsequently isolate putative MP polymers from the digestive tract without damaging their integrity. Five treatments were examined for their capacity to break down chitin from the prawn digestive system; namely acid, alkaline, oxidant, enzyme and microwave assisted oxidant digestion. Gravimetric and image analysis revealed that the organic tissue of the prawn gastrointestinal tract can be effectively removed by acid, oxidant, and microwave assisted oxidant digestion methods. However, testing on seven reference polymers (polyamide (PA), polyethylene (PE), polyester (PES), polypropylene (PP), polystyrene (PS), polyvinyl chloride (PVC), and rayon) revealed significant degradation when exposed to acid digestion. Overall, microwave assisted oxidant digestion achieved the best recovery rate of spiked MPs (
1 Introduction
Microplastics (MPs) are a heterogenous mixture of plastic polymers with a size less than 5 mm (Dawson et al., 2021), which have become ubiquitous in the marine environment due to extensive plastic pollution (Thompson et al., 2004). Presence of these particles in marine organisms raises increasing concerns over animal welfare and food safety, given that seafood compromises over 17% of animal protein consumption by humans globally (Murray and Cowie, 2011; FAO, 2018). Therefore, it is of extreme importance that the risk seafood presents to consumers, in terms of exposure and health effects, is accurately quantified. To do this comprehensively, not only should MP contamination be quantified across the breadth of globally consumed organisms, but the MP concentration in both the edible and discarded tissues should be quantified separately, so that the risk of human exposure to MP contaminated seafood can be accurately assessed (Dawson et al., 2021). At this stage, little is known about MPs in large decapod crustaceans, such as prawns and crabs (de Sá et al., 2018), in part, due to a lack of efficient protocols to extract ingested MPs from the structurally sophisticated digestive tract of decapods (Enders et al., 2017).
The digestive tract of decapods is composed of the oesophagus, foregut (also termed proventriculus), digestive gland (also termed hepatopancreas or midgut gland), the midgut, and the hindgut (rectum, anus) (Figure 1A) (Felgenhauer, 1992; Hill et al., 2004), where all regions except the midgut and digestive gland are coated by complexes of chitin and protein (Ceccaldi, 1989; Felgenhauer, 1992). Since chitin is resistant to degradation by many chemicals including water, organic solvents, mild acidic or basic solutions (Roy et al., 2017), its presence within the digestive tract of decapods has previously been suggested to inhibit the extraction of MP from organic tissue (Cole et al., 2014). For example, potassium hydroxide (KOH) is a commonly used solution which effectively dissolves the organic tissue of most taxa for the extraction of MP (Dawson et al., 2020). However, when applied to decapod intestinal tracts, KOH has been shown to be ineffective, with intact gut linings visible amongst the filter retentate (Figure 1B) (Hara et al., 2020). Thus, there is scope to explore suitable methodologies to extract MP from the digestive tracts of large crustaceans.
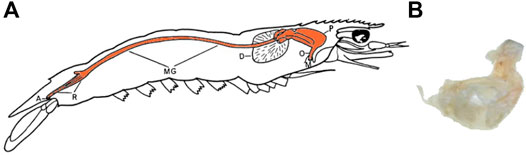
FIGURE 1. Prawn anatomy. (A) the digestive tract diagram of a penaeid prawn, adapted from Dall (1968), the orange shaded area is the digestive tract being preserved in all samples (A—anus, D—digestive gland or hepatopancreas, M—mouth, MG—midgut, O—oesophagus, P—proventriculus or foregut, R—rectum). (B) the remaining gut lining of the N. norvegicus after the application of the digestion protocol using potassium hydroxide (KOH) at 40°C (Hara et al., 2020).
Conventional MP extraction methodologies that could remove chitin should be considered in this particular scenario. Conventional treatments usually involve dissection alone or in combination with chemical (acids, oxidative, and alkaline reagents) or biological (enzyme) digestion (Thompson et al., 2004; Choy and Drazen, 2013; Lusher et al., 2017; Miller et al., 2017). Direct dissection (Welden and Cowie, 2016; Wójcik-Fudalewska et al., 2016) or acid digestion (Desforges et al., 2015; Devriese et al., 2015) have been effectively shown to remove chitin, allowing MP extraction. However, dissection without a tissue digestion step may miss MP, and acids are known to degrade some polymers. Further, specific chitinase enzymes are also available for chitin digestion (Roy et al., 2017). Microwave assisted digestion is used extensively in removal of organic matter to facilitate trace metal analysis (Kuss, 1992; Lamble and Hill, 1998; Ritter et al., 2004) and capable of degrading chitin to chitosan and glucosamine (Shao et al., 2003; Wojtasz-Paja̧k and Szumilewicz, 2007), but as of yet have not been used in MP research. However, the high temperature used in microwave ovens may be capable of degrading certain polymers, particularly when used in conjunction with harsh chemicals. (Castle et al., 1990; Sakurai et al., 2006; Karami et al., 2017). Few studies have validated MP extraction methods against reference polymers to ensure their integrity (Desforges et al., 2015; Abbasi et al., 2018; Cau et al., 2019; Hara et al., 2020). Even fewer have systematically tested the impact of digestion methods on MPs in large crustaceans.
Therefore, the goal of this study was to develop a suitable MP extraction method for decapod digestive tracts. Five treatment methods were adapted from the literature focusing on general organic tissue removal or chitin degradation (acid, base, oxidant, enzyme, and microwave assisted oxidant digestion) (Cole et al., 2014; Miller et al., 2017; Piarulli et al., 2019; Wojtasz-Paja̧k and Szumilewicz, 2007). The treatments were tested on the digestive tract of banana prawns (Penaeus merguiensis) since they are arguably the most commercially valuable species of penaeid prawns distributed accrosee tropical and sub-tropical regions. (Fischer and Bianchi, 1984; Göltenboth and Schoppe, 2006; Safaie, 2015; Vance and Rothlisberg, 2020). Specifically, this work will contribute to an efficient and effective digestion protocol for banana prawn digestive tracts by 1) comparing several common tissue digestion methods and assessing their ability to break down the chitinous tissue present within prawn digestive tract; and 2) evaluating the impacts of satisfactory treatments on the physical and chemical traits of known reference synthetic polymers.
2 Materials and Equipment
Banana prawns (P. merguiensis) were obtained from commercial sources. The oesophagus, foregut and mid gut (Figure 1A) of prawns were carefully dissected from surrounding tissue, weighed, and stored at −20°C in glass containers if not used immediately. The digestive gland was excluded from analysis as it does not contain any chitinous lining. Furthermore, the particles able to pass through the filter-press from the foregut are usually less than 1 µm (Wade et al., 2018) which is currently below the size detection limits for most MP analysis methods.
Nitric acid (70% HNO3) (UN No. 2031), 30% hydrogen peroxide (H2O2) (CAS No. 7722-84-1), absolute ethanol (EtOH) AR grade (CAS No. 64-17-5) were all sourced from Thermo Fisher Scientific. 30% H2O2 and absolute EtOH were also diluted to 10% H2O2 and 70% EtOH with Milli-Q (Millipore) water (Milli-Q H2O). Potassium Hydroxide (KOH) pellets (Thermo Fisher Scientific UNILAB CAS No. 1310-58-3) were dissolved with Milli-Q (Millipore Merck) water to form a 10% w/v aqueous solution. Protease K (Bioline/Meridian Bioscience Cat. No. BIO-37039, 40.8 U mg−1, 20 mg ml−1) and Chitinase (entochitinase, EC 3.2.1.14, ASA Spezialenzyme GmbH, Germany, 100 U ml−1) was stored at −20°C as lyosphilized powder and stable solution with 50% glycerin respectively. Homogenization solution for protease K was prepared with 400 mM Tris-HCl buffer, 60 mM EDTA, 105 mM NaCl and 1% Sodium Dodecyl Sulfate (SDS) to achieve pH 8.0. Citric acid buffer was prepared using 0.1 M Sodium citrate and 0.1 M citric acid buffer to achieve pH 5.6 for chitinase. A total of seven different synthetic polymers were selected based on the previously reported particles or fibers in crustaceans (Murray and Cowie, 2011; Cole et al., 2014; Brennecke et al., 2015; Wójcik-Fudalewska et al., 2016; Abbasi et al., 2018; Cau et al., 2019). These were: polyamide (PA, 1–2 mm), polyethylene (PE,
3 Methods
3.1 Preventing Contamination
Strict procedures were followed in order to minimize MP contamination with the laboratory throughout analysis. A full-cotton (100%) lab coat dyed green was worn during all experimental procedures. All containers were made of glass and stainless steel and rinsed with tap water, followed by three rinses in reverse osmosis (RO) water. All equipment and samples were covered when not in use. The cover or caps of the containers were either aluminium foil or plastic with polytetrafluoroethylene (PTFE) lining depending on the compatibility of the chemicals used. Solutions and liquids were prefiltered to remove contaminants with PTFE filters (0.45 µm pore size, 47 mm Ø diameter, Millipore). A stainless steel (316 grade) filtration system was used with sizes (263, 77 and 26 µm) to obtain post treatment residual. Three pore sizes (263, 77 and 26 µm) of stainless steel filters (19 mm Ø) were punched from woven mesh. All samples were prepared in laminar flow and were rinsed thoroughly with Milli-Q water before and after dissection. Digestion and filtration was carried out in either a fume hood (acid digestion) or laminar flow (base, oxidant digestion).
3.2 Method Development
Following the stepwise approach to select an optimum extraction treatment in fish (Karami et al., 2017), the experimental design of this study was divided into three phases (Figure 2).
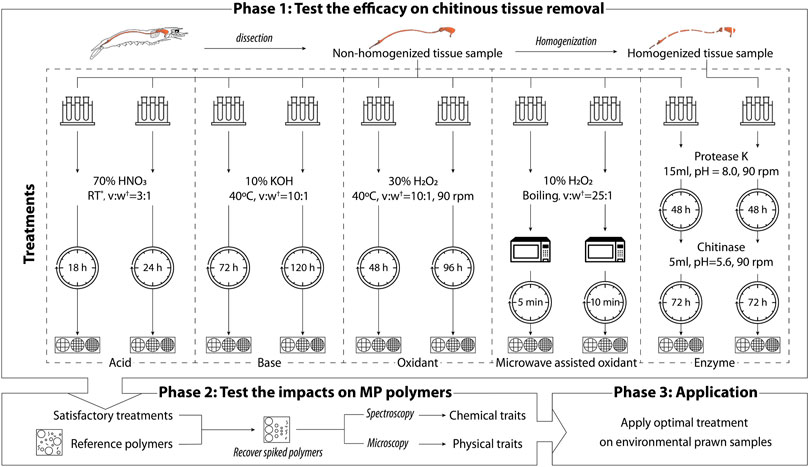
FIGURE 2. Schematic summary of the microplastic extraction treatments on prawn digestive tracts. * RT—room temperature (approx. 22–24°C). †v:w is the proportion between the volume of chemical added and the wet weight of the tissue sample.
Two variations (treatment duration or homogenization status of the tissue sample) were compared for each treatment according to preliminary visual assessments. The results of Phase 1 were assessed using gravimetric and image analysis to select satisfactory treatments. The satisfactory methods obtained in Phase 1 subsequently proceeded to Phase 2. Phase 2 evaluated the impact on reference polymers by analyzing the physical and chemical characteristics of polymers before and after treatment using microscopy image analysis and spectroscopy. Combining the result of Phase 1 and Phase 2, the optimal treatment is further applied to environmental sample captured in the wild. The results of Phase 3 are available in Dawson et al. (2022).
3.3 Tissue Sample Digestion Treatment in Phase 1
A total of five digestion methods with two variations of each were chosen based on preliminary tests via visual assessment of the remaining chitinous gut tissue after treatment (Figure 2). Each method was tested in triplicate following the protocols outlined below. All treated samples were filtered sequentially onto pre-weighed filters. These were then rinsed with Milli-Q water followed by 70% EtOH using a PTFE wash bottle, to reduce the possibility of particulates adhering to the sides of the container and to remove any chemical residue. Filters and the filtration system were flushed at least three times or until there were no particulates adhering to the system. Filters were dried overnight (approx. 18–24 h) at room temperature (approx. 22–24°C) and stored in a desiccator. All treatments except microwave assisted oxidant treatment were conducted at 40°C or below to achieve maximum enzyme activity and reduce MP degradation at high temperature, as previous studies have thoroughly demonstrated MP degradation above 50°C (Karami et al., 2017; ?). Once the treated samples were completely dry and re-weighed, the remaining residue was stained with lactophenol cotton blue, a commonly used staining agent demonstrating an affinity for chitin (Leck, 1999). The staining agent gave chitin a blue colour that was used to visualize the chitinous residues remaining on the filter after digestion.
3.3.1 Acid Treatment
70% HNO3 was added to the tissue samples at a ratio of 10:1 v:w, or until covering the sample. Samples were then covered with PTFE lined caps and stored at room temperature inside a fume hood during the treatment time (12 or 24 h). Before filtration, the solution was neutralized using 10% KOH in a ratio of 3:1 (v:v, 10% KOH: 70% HNO3).
3.3.2 Base Treatment
10% KOH was added to the tissue samples at a ratio of 10:1 v:w or until the sample was submerged. Samples were incubated at 40°C in an oven during the treatment time (72 or 120 h).
3.3.3 Oxidant Treatment
30% H2O2 was added to the tissue samples at a ratio of 10:1 v:w or until the sample was submerged. Samples were incubated in a Thermoline incubator at 40°C and shaken at 90 rpm throughout the treatment time (48 or 96 h).
3.3.4 Enzyme Treatment
Each frozen tissue sample was homogenized using a glass rod. Both homogenized and non-homogenized tissue sample were digested in Proteinase-K in 15 ml homogenizing solution (pH = 8.0) for 48 h. Samples were then filtered and rinsed before adding chitinase with citric acid buffer solution (pH = 5.6) for a further 72 h. Protease digestion was maintained at 40°C and chitinase was maintained at 37°C in the incubator and shaken at 90 rpm throughout the treatment.
3.3.5 Microwave Assisted Oxidant Treatment
10% H2O2 was added to the tissue samples at a ratio of 25:1 v:w in a glass tube with a small glass beaker loosely covering the top to prevent a buildup of pressure within sample tubes. Samples were heated to the boiling state inside the microwave at half power (i.e., 550 W). Samples were closely monitored for throughout digestion, with the heating briefly paused periodically to avoid overflow of the reaction agent. The microwave time, 5 or 10 min (Figure 2) was the run time only, without taking the cooling-off time into account.
3.4 Sample Digestion in Phase 2 With Spiking Reference Polymers
Based on Phase 1, the three most satisfactory digestion methods were selected to evaluate their impacts on seven different types of polymers: polyamide (PA), polyethylene (PE), polyester (PES), polypropylene (PP), polystyrene (PS), polyvinylchloride (PVC), and rayon. Each sample was spiked with 10 replicates of each polymer type. Triplicates of spiked samples were subsequently digested using the same protocols outlined in Subsection 3.3.
3.5 Gravimetric Analysis (Phase 1)
Digestive efficiency is for each method was calculated as per Karami et al. (2017) using Equation (1). Treatments resulting greater than 95% average digestive efficiency among all three replicates were considered efficiently digested.
where Wi = Initial wet weight of tissue samples and spiked polymers; Wa = Weight of dry tissue and stainless steel filters after filtration; Wb = Weight of stainless steel filters before filtration.
3.6 Image Analysis (Phase 1 and 2)
Digestive efficiency alone has recently been suggested to be an inadequate metric to evaluate complete digestion (Dawson et al., 2020). Thus in Phase 1, image analysis was applied to examine the retention of chitinous tissue after treatment as visualized by lactophenol cotton blue stain: 1) overall digestion level, 2) normalized undigested residue coverage, and 3) average blue value. True colour, high resolution, RGB images were taken using a Leica Stereo microscope MZ16A. Overall digestion level was defined as a score (1–4, with higher scores relating to lower residual tissue) assigned to the status of remaining residues on 263 µm filters for each sample. The four digestion scores (Figure 3) corresponded to the following criteria:
• 1: very poor, i.e. the entire esophagus and proventriculus chambers intact;
• 2: poor, i.e. the esophagus and proventriculus chambers partially intact;
• 3: moderate, i.e. no obvious chitinous structure with many irregular particles;
• 4: good, i.e. no obvious chitinous structure with little irregular particles.
Both normalized undigested residue coverage and average blue value were computed using a computer vision image analysis algorithm (OpenCV library with Python 3.8) (Bradski, 2000). All images were taken under consistent brightness and nadir angle with a microscopic camera as 8-bit true colour RGB images with pixel having a value for the brightness of the red, blue, and green colour ranging from zero to 255 (with zero indicating no light and 255 indicating maximum light). The algorithm detected the area with remaining tissues and calculated the percentage coverage of undigested residual over the whole filter (Figure 4A) as well as the average value encoded in the blue channel of all pixels located in the detected area (Figure 4B). A black and white mask was created for both filter area and residue area ((Figure 4A) which were quantified by their pixel count, normalised by the wet weight of the tissue measured during the sample preparation stage. The undigested residual coverage measures how widely the residue was spread over the filter per unit weight. I.e., a high normalised undigested residual value implies the tissue sample had been broken down into small pieces that are able to spread over the filter. The last criteria, average blue value, was used as an indicator to show how well chitin has been broken down due to the binding specificity of the colouring agent. A higher the value suggests that less colouring agent has combined with the residue, thus, more chitin has been digested.
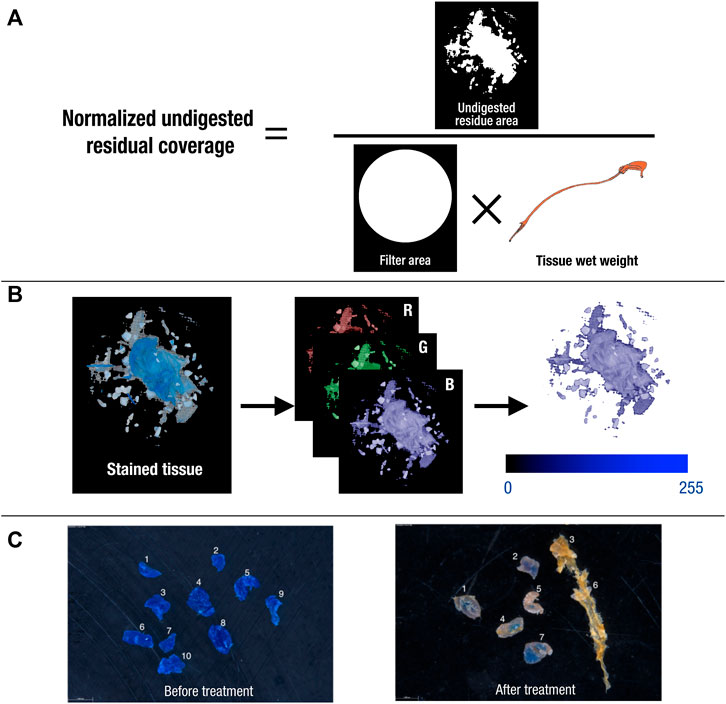
FIGURE 4. Illustration of image analysis protocol for (A) using masks of filter and residue and sample tissue wet weight to calculate “normalized undigested residue coverage”, (B) extracting blue channel from RGB images of stained tissue to calculate “average blue value”, and (C) an example of reference polymers (e.g. PE) comparison before and after treatment.
Treatments achieving an average value equal or greater than the value of third quartile (Q3), i.e. top 25% of the values in the dataset, for each assessment were considered acceptable in Phase 1.
Image analysis was also performed in Phase 2 to evaluate the degradation of each treatment on the physical properties of each polymer. The size of spiked reference polymers was determined by the length of fibres or the Feret’s diameter of particles, which were measured from microscopic images before the treatment (i.e. virgin polymers), and after the treatment (i.e. exposed polymers) in FIJI ImageJ (Schindelin et al., 2012) (Figure 4C). Polymer recovery rate was calculated using the equation below:
where Nv = Counts of virgin reference polymers before treatment; Ne = Counts of exposed reference polymers after treatment.
3.7 Spectroscopic Analysis (Phase 2)
Reference polymers also had their chemical characteristics analysed using Fourier transform infrared (FTIR) spectroscopy with a PerkinElmer Spectrum 100 Spectrometer (16 scans at 4 cm−1 resolution, wavenumber range = 4,000-600 cm−1). Spectra of virgin (n = 3) and exposed polymers (n = 9) were acquired measuring attenuated total reflectance (ATR-FTIR). Spectral signature of virgin and exposed polymers were correlated within the PerkinElmer Spectrum IR software, generating a coefficient of correlation (R) (Kroon et al., 2018). If exposed polymers obtained R that is greater than 0.9, they were considered as very similar to the virgin polymers.
3.8 Data Analysis
Tukey’s boxplots were adopted to visualize the distribution of all collected data (Tukey, 1977). Mann-Whitney test (RStudio 1.4.1106) was performed to check whether there was a significant difference between the size of reference polymers before and after treatment in Phase 2.
4 Results
4.1 Efficacy on the Chitinous Tissue Sample Removal
Acid, oxidant, and microwave assisted oxidant digestion methods resulted in digestive efficiency values greater than 95% (Figure 5A). Both variations of acid digestion resulted in digestive efficiency
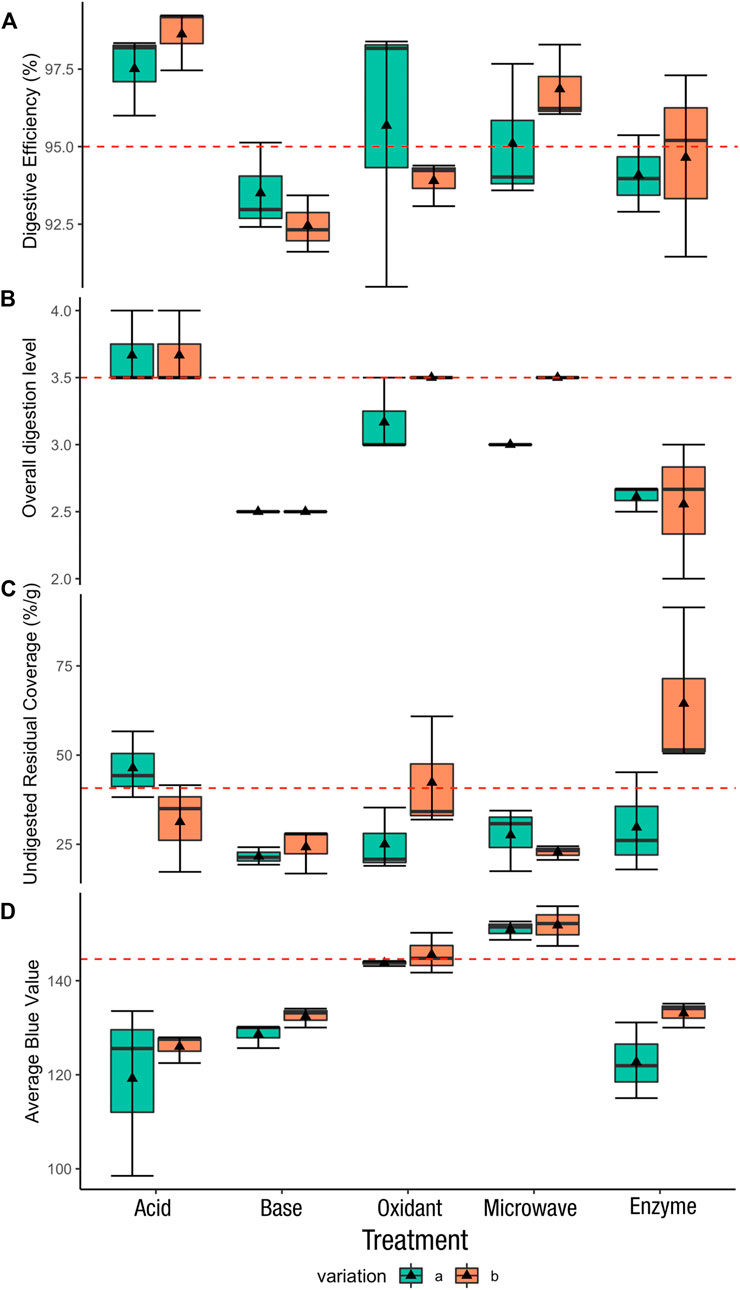
FIGURE 5. Boxplot for all evaluated aspects. The triangle marker is the mean value of each treatment. The red dash line is the cut-off line: (A) digestive efficiency—95% adopted from (Karami et al., 2017), (B) normalized overall digestion level—Q3 = 3.5, (C) undigested residue coverage—Q3 = 40.7%, (D) average blue value—Q3 = 145.
Overall, base digestion and enzyme digestion failed to satisfy most of the evaluation criteria (Table 1). Whereas acid, oxidant and microwave assisted oxidant digestion all had one variation each that met 3 of the 4 cut-off criteria. These were the 70% HNO3 for 24 h, 30% H2O2 for 96 h, and 10% H2O2 10 min microwave method. Thus, these three treatments proceeded to the next phase. The details of the performance of each treatment can be seen in supplementary material (Supplementary Table S2).

TABLE 1. Summary of gravimetric and image analysis results in an evaluation matrix, where a tick (✓) indicates that the cut-off criteria is met.
4.2 Impacts of Selected Treatments on Reference Polymers
Recovery rate, colour and spectra of MPs exposed acid, oxidant and microwave assisted treatments varied considerably, while, other than PA, the shape and size did not show significant changes for all recovered polymers. Rayon fibers were unable to be recovered in any treatment, and PVC fragments were fully recovered in all treatments. PE, PP, and PS were almost fully recovered in all treatments. PA and PES had very low recovery rate (0 and 36.7%, respectively) after being treated with 70% HNO3 for 24 h (Table 2). Moreover, the image analysis further showed that PE fragments and PES fibers were discoloured, and the surface of some polymers were smoothed after the treatment (Supplementary Figure S2). Minor color and morphological changes occurred during oxidant and microwave assisted oxidant treatments, however some remaining tissue residue adhered to the surface of the polymers (Supplementary Figures S3, S4). The size distribution of recovered exposed polymers was very similar compared to virgin polymers (Figure 6). The exposed PE, PES, PP, PS, and PVC polymers show no significant difference to the virgin polymers (Supplementary Table S3). Only PA recovered from microwave assistant treatment had significant changes in size (Mann-Whitney test, nv = 30, ne = 28, p = 0.0014). The average length of PA fragments before and after the treatment was 1.71 ± 0.05 mm and 1.54 ± 0.04 mm respectively. Notwithstanding these significant differences, the changes in size were small and the polymers had no obvious shape and colour changes.

TABLE 2. Recovery rate of reference polymers under acid, oxidant and microwave assisted oxidant treatment (PA—polyamide, PE—polyethylene, PES—polyester, PP—polypropylene, PS—polystyrene, PVC—polyvinyl chloride).
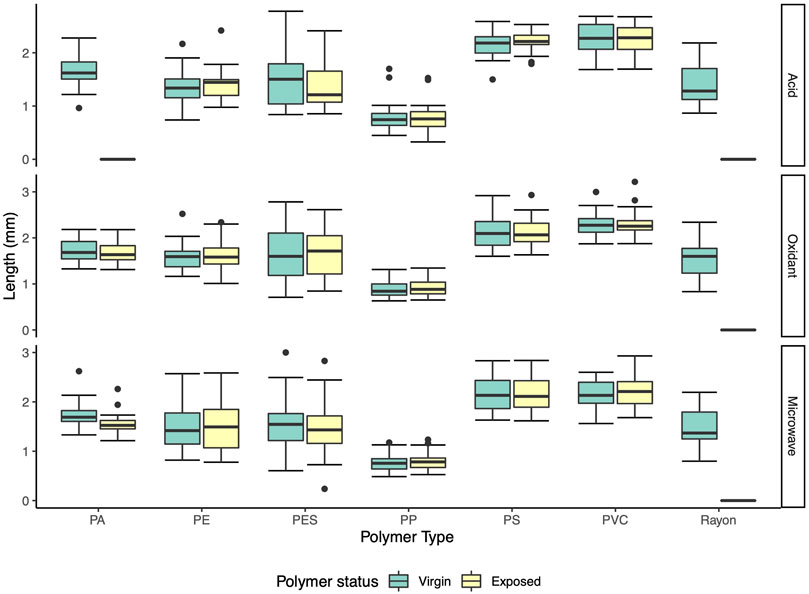
FIGURE 6. Size distribution of virgin and exposed polymers. PA—polyamide, PE—polyethylene, PES—polyester, PP—polypropylene, PS—polystyrene, PVC—polyvinyl chloride.
In terms of the chemical characteristic changes after each treatment, polymers recovered from microwave assisted oxidant digestion had very high FTIR spectra correlation scores (R > 0.95 for all nine polymer types on average) to their virgin polymer counterparts (Figure 7, Supplementary Table S4). After oxidant treatment, the correlation score of exposed polymers to the virgin polymer spectrum library showed that PA, PE, PES and PP had high average correlation scores for recovered polymers (R > 0.9) while PS (R = 0.81 ± 0.07) and PVC (R = 0.76 ± 0.06) had low scores. As for acid treatment, PA were unable to recovered with no correlation score available and the score of PVC polymer was the lowest (R = 0.75 ± 0.03) amongst all polymers recovered.
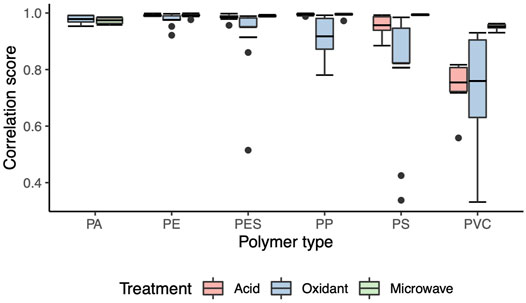
FIGURE 7. Correlation score distribution of all recovered polymers for each treatment. PA*—polyamide, PE—polyethylene, PES—polyester, PP—polypropylene, PS—polystyrene, PVC—polyvinyl chloride. * PA was not able to be recovered from the acid treatment and thus this data is absent.
5 Discussion
5.1 Optimal Tissue Removal and MP Extraction Approach for Prawns
The oxidant treatment successfully removed the chitinous lining of the prawn digestive tract, however the addition of microwave radiation to this treatment resulted in more complete and efficient digestion while maintaining the integrity of the polymer samples. Even though the solution was heated to the boiling point of the 10% H2O2 solution (approx. 100°C), the spectral signature of exposed polymers remained very similar to the virgin polymers. In contrast, colour change has previously been described in PA and PVC at temperatures of 50–60°C, which is likely a result of prolonged incubation (96 h) using traditional heating oven (Karami et al., 2017). Microwave heating converts electromagnetic energy to thermal energy by water molecule oscillation rather than relying on heat transfer from the ambient environment using ovens or incubators (Kuss, 1992; Sun et al., 2016). As a result, the materials in microwaves are heated with a uniform thermal field in much less time (Sun et al., 2016). The short reaction time may reduce the likelihood of MP degradation as the high temperature is only maintained very briefly compared to other digestion methods used in our study. Furthermore, the microwave energy creates a small but consistent vibration of the molecules throughout the solution. Mechanical movement facilitates the digestion process (Cole et al., 2011; Therien et al., 2019) making it likely that the molecular vibration imparted by microwaves would improve digestion of chitin. Therefore, in the absence of significant MP degradation, microwave assisted oxidant digestion presents a promising approach for a standardized method of MP extraction and quantification from crustacean gastrointestinal tracts. It is worth point out in this study that relatively large MPs were used for ease of manipulation and measurement. Although these particles are still within the size range of microplastics, further studies should verify that this method also applies to smaller MP (<1 mm). Recent method developments using very small immobilised MPs may be of use to validate this digestion method (?).
This study further confirmed that treating prawn digestive tracts with 70% HNO3 at room temperature for 24 h could remove the majority of the tissue samples with very high digestive efficiency (
In contrast to acid digestion, alkaline and enzyme treatments failed to break down the complex lining in the foregut, resulting in intact proventriculus in all replicates (Supplementary Figure S5). It is reasonable to suspect that any MP polymers present inside the proventriculus would not be isolated for further analysis, as isolation and identification of putative MP is based on visual inspection of the filter residue after digestion. KOH is one of the most widely used chemical digestion solutions for MP extraction, but extensive treatment with 10% KOH (40°C for 120 h) without degrading the lining could be a result of insolubility of chitin in mild basic solutions. The samples treated sequentially with protease and chitinase also failed to break down the tissue.This is consistent with a recent study by (Kallenbach et al., 2021), demonstrating that the chitinous exoskeleton of terrestrial isopods can resist broad-spectrum enzymatic digestion. A combination of pharmaceutical pancreatic enzyme mixes and chitinase was applied to the isopod for 48 h (24 h for pancreatic enzyme mix and 24 h for chitinase), yet the exoskeleton remained intact. On the other hand, the study claimed that a combination of 30% H2O2 and chitinase has removed the exoskeleton efficiently (Kallenbach et al., 2021). Since the experiment design did not compare this method with H2O2 treatment only, our current results suggest that the success of chitin degradation has little to do with chitinase, but mainly due to the addition of H2O2, which is same as the oxidant treatment used in this study. Chitinase is widely used to derive glucosamine from the α-chitin in shrimp and crab shells (Chen et al., 2010). Enzymatic hydrolysis the preferred method in industrial process due to its environmental friendliness comparing to other methods that tend to produce large quantities of chemical waste (Chen et al., 2010). Various chitinolytic enzymes exist in the wild including endochitinases (EC 3.2.1.14), exochitinases (EC 3.2.1.52), chitobiosidases (EC 3.2.1.30) and N-acetylglucosaminidases (NAGases) (EC 3.2.1.96) (Chen et al., 2010). This study tested endochitinases which would cleave chitin at internal sites on the glycosidic bonds randomly to generate the soluble oligosaccharides (Chen et al., 2010; Wang et al., 2019). There is little information about the chemical composition and structural difference amongst the chitinous tissues from the proventriculus and oesophagus, and that of the exoskeleton, which is widely used for glucosamine production. This knowledge gap may have hindered the use of targeted enzymes, thus leading to insufficient breakdown of the endogenous chitinous tissue in the prawns. It is also important to note that the aim of industrial chitinase utilization is yield of glucosamine rather than the complete removal of tissues to reveal their contents, so while an enzyme may be efficient for industrial aims, it is not applicable to MP research in this context.
5.2 Digestion Efficiency as an Indicator of Method Suitability
The five treatments were assessed by combining all the results into an evaluation matrix, in order to make an informed decision on the method suitability. In conventional MP extraction methodology development, the optimal treatment is typically selected based on one or more of the following criteria: digestive efficiency, weight changes of reference polymers, and FTIR spectral changes of the polymers (Cole et al., 2014; Desforges et al., 2015; Devriese et al., 2015; Karami et al., 2017; Hara et al., 2020). The results of this study clearly demonstrate that in cases involving crustacean digestive systems, digestive efficiency is not sufficient for method evaluation. For instance, the oxidant treatment (30% H2O2, 48 h) achieved a digestive efficiency of 95.68 ± 2.60%, but visual inspection showed that the undigested residuals were still clustering after filtration, making isolation of putative particles impossible (Supplementary Figure S6). The KOH treatment recommended by Hara et al. (2020) showed a similar pattern of results, with intact proventriculus material clearly visible despite a gravimetric digestive efficiency greater than 95% (Figure 1B). Furthermore, MPs can be too light to measure accurately, rendering gravimetric measures severely limited. Using larger particles that are able to be weighed is also problematic, as larger particles are more stable due to diminished surface to volume ratios. As demonstrated in this study, visual inspection of remaining residues could play a crucial role in verifying digestion methods.
It is worth noting that the evaluation matrix is not identical among all tissues. A selection of gravimetric analysis and image analysis will be case specific. In this study, digestive efficiency was the only conventional decision criterion in MP studies, while the three image analysis criteria were developed according to specific features of the prawn digestive tract. Overall digestion level is a straightforward visual cue to evaluate if the chitinous lining still exits after treatment, which may not apply to other biota or tissues that lack clear structures. Undigested residual coverage is highly related to the filtration technique. The rinsing and washing angle and force could influence the distribution of the particles. Thus, it is crucial to keep the operating procedure consistent throughout the experiment. It may also be desirable to increase the sample size in order to mitigate any potential artefacts. Additionally, the computer vision algorithm chosen to determine the particle distribution on filters also played an important role in the evaluation criteria. Since it is impossible to manually select all fine particles on the filters, the presence of distributed particles were approximately detected using the OpenCV library with the embedded Gaussian blur and binary threshold functions (Bradski, 2000). The optimization of the parameters for these functions could improve the detection accuracy in future research.
6 Conclusion
In conclusion, microwave assisted oxidant digestion outperformed the traditional acid, alkaline, oxidant and enzyme digestion treatments in MP extraction and quantification. The method achieved high digestion efficiency and satisfactory visual assessment. Thus, it was successfully applied to disintegrate the lining of P. merguiensis digestive tracts. Importantly, six out of seven types of reference polymers were recovered at a high rate with minimal shape, size, and spectral changes after treatment. This has highlighted a new method to efficiently remove difficult organic tissues and thereafter identify putative MP particles.
Data Availability Statement
The original contributions presented in the study are included in the article/Supplementary Materials, further inquiries can be directed to the corresponding author.
Author Contributions
JL conceptualized the study, acquired funding, carried out the laboratory analysis, analysed the data, and wrote the original draft. JL and AD acquired funding for the project. AD and LN contributed to the conceptualization methodology, reviewed and edited the manuscript.
Funding
This project was funded from the Key Program for International S\&T Cooperation Projects: Sino-Australian Center for Healthy Coasts (No. 2016YFE0101500), as well as the Australia China Science Research Fund grant ACSRF 48162, Australian Government Department of Industry, Innovation and Science, and the Queensland Government Department of Environment and Science. It was also supported by the AIMS@JCU Pilot Research grant and James Cook University SSA nominal fund from College of Science and Engineering. Funding bodies have had no input into study design; the collection, analysis and interpretation of data; writing of the report; or the decision to submit the article for publication.
Conflict of Interest
The authors declare that the research was conducted in the absence of any commercial or financial relationships that could be construed as a potential conflict of interest.
Publisher’s Note
All claims expressed in this article are solely those of the authors and do not necessarily represent those of their affiliated organizations, or those of the publisher, the editors and the reviewers. Any product that may be evaluated in this article, or claim that may be made by its manufacturer, is not guaranteed or endorsed by the publisher.
Acknowledgments
We would like to acknowledge the Bindal and Wulgarukaba people as the traditional owner and custodians of the land where the study is conducted. We thank the commercial and recreational fishers who provided prawn for this study. We also appreciate the help and feedback from AIMS Microplastic team.
Supplementary Material
The Supplementary Material for this article can be found online at: https://www.frontiersin.org/articles/10.3389/fenvc.2022.903314/full#supplementary-material
References
Abbasi, S., Soltani, N., Keshavarzi, B., Moore, F., Turner, A., and Hassanaghaei, M. (2018). Microplastics in Different Tissues of Fish and Prawn from the Musa Estuary, Persian Gulf. Chemosphere 205, 80–87. doi:10.1016/j.chemosphere.2018.04.076
Bradski, G. (2000). The OpenCV Library. Dr. Dobb’s Journal of Software Tools https://github.com/opencv/opencv/wiki/CiteOpenCV.
Brennecke, D., Ferreira, E. C., Costa, T. M. M., Appel, D., da Gama, B. A. P., and Lenz, M. (2015). Ingested Microplastics (>100μm) Are Translocated to Organs of the Tropical Fiddler Crab Uca Rapax. Mar. Pollut. Bull. 96, 491–495. doi:10.1016/j.marpolbul.2015.05.001
Castle, L., Jickells, S. M., Gilbert, J., and Harrison, N. (1990). Migration Testing of Plastics and Microwave‐active Materials for High‐temperature Food‐use Applications. Food Addit. Contam. 7, 779–796. doi:10.1080/02652039009373940
Cau, A., Avio, C. G., Dessì, C., Follesa, M. C., Moccia, D., Regoli, F., et al. (2019). Microplastics in the Crustaceans Nephrops norvegicus and Aristeus Antennatus: Flagship Species for Deep-Sea Environments? Environ. Pollut. 255, 113107. doi:10.1016/j.envpol.2019.113107
Ceccaldi, H. (1989). “Anatomy and Physiology of Digestive Tract of Crustaceans Decapods Reared in Aquaculture,” in Actes de Colloques Ifremer (Tahiti, French Polynesia: AQUACOP IFREMER), N°9, 243
Chen, J.-K., Shen, C.-R., and Liu, C.-L. (2010). N-acetylglucosamine: Production and Applications. Mar. Drugs 8, 2493–2516. doi:10.3390/md8092493
Choy, C., and Drazen, J. (2013). Plastic for Dinner? Observations of Frequent Debris Ingestion by Pelagic Predatory Fishes from the Central North Pacific. Mar. Ecol. Prog. Ser. 485, 155–163. doi:10.3354/meps10342
Claessens, M., Van Cauwenberghe, L., Vandegehuchte, M. B., and Janssen, C. R. (2013). New Techniques for the Detection of Microplastics in Sediments and Field Collected Organisms. Mar. Pollut. Bull. 70, 227–233. doi:10.1016/j.marpolbul.2013.03.009
Cole, M., Lindeque, P., Halsband, C., and Galloway, T. S. (2011). Microplastics as Contaminants in the Marine Environment: A Review. Mar. Pollut. Bull. 62, 2588–2597. doi:10.1016/j.marpolbul.2011.09.025
Cole, M., Webb, H., Lindeque, P. K., Fileman, E. S., Halsband, C., and Galloway, T. S. (2014). Isolation of Microplastics in Biota-Rich Seawater Samples and Marine Organisms. Sci. Rep. 4, 4528. doi:10.1038/srep04528
Dall, W. (1968). Food and Feeding of Some Australian Penaeid Shrimp. FAO Fish. Rep. 2, 251–258. doi:10.1136/bmj.2.5600.301-c
Dawson, A. L., Li, J. Y. Q, and Kroon, F. J. (2022). Plastics for Dinner: Store-Bought Seafood, but Not Wild-Caught From the Great Barrier Reef, as a Source of Microplastics to Human Consumers. Environ. Adv. 8, 100249. doi:10.1016/j.envadv.2022.100249
Dawson, A. L., Motti, C. A., and Kroon, F. J. (2020). Solving a Sticky Situation: Microplastic Analysis of Lipid-Rich Tissue. Front. Environ. Sci. 8. doi:10.3389/fenvs.2020.563565
Dawson, A. L., Santana, M. F. M., Miller, M. E., and Kroon, F. J. (2021). Relevance and Reliability of Evidence for Microplastic Contamination in Seafood: A Critical Review Using Australian Consumption Patterns as a Case Study. Environ. Pollut. 276, 116684. doi:10.1016/j.envpol.2021.116684
de Sá, L. C., Oliveira, M., Ribeiro, F., Rocha, T. L., and Futter, M. N. (2018). Studies of the Effects of Microplastics on Aquatic Organisms: What Do We Know and where Should We Focus Our Efforts in the Future? Sci. Total Environ. 645, 1029–1039. doi:10.1016/j.scitotenv.2018.07.207
Desforges, J.-P. W., Galbraith, M., and Ross, P. S. (2015). Ingestion of Microplastics by Zooplankton in the Northeast Pacific Ocean. Arch. Environ. Contam. Toxicol. 69, 320–330. doi:10.1007/s00244-015-0172-5
Devriese, L. I., van der Meulen, M. D., Maes, T., Bekaert, K., Paul-Pont, I., Frère, L., et al. (2015). Microplastic Contamination in Brown Shrimp (Crangon crangon, Linnaeus 1758) from Coastal Waters of the Southern North Sea and Channel Area. Mar. Pollut. Bull. 98, 179–187. doi:10.1016/j.marpolbul.2015.06.051
Enders, K., Lenz, R., Beer, S., and Stedmon, C. A. (2017). Extraction of Microplastic from Biota: Recommended Acidic Digestion Destroys Common Plastic Polymers. ICES J. Mar. Sci. 74, 326–331. doi:10.1093/icesjms/fsw173
FAO (2018). The State Of World Fisheries And Aquaculture 2018: Meeting The Sustainable Development Goals. No. 2018 in the State of World Fisheries and Aquaculture (SOFIA). Rome, Italy: FAO.
Felgenhauer, B. E. (1992). Internal Anatomy of the Decapoda: An Overview. Microsc. Anat. Invertebr. 10, 45.
Fischer, W., and Bianchi, G. (1984). FAO Species Identification Sheets for Fishery Purposes: Western Indian Ocean (Fishing Area 51). Rome, Italy: Tech. rep. v. 1: Introductory Material. Bony Fishes, Families: Acanthuridae to Clupeidae.- v. 2: Bony Fishes, Families: Congiopodidae to Lophotidae.- v. 3: …Families: Lutjanidae to Scaridae.- v. 4: …Families: Scatophagidae to Trichiuridae.- v. 5: Bony Fishes, Families: Triglidae to Zeidae. Chimaeras. Sharks. Lobsters. Shrimps and Prawns. Sea Turtles. v. 6: Alphabetical Index of Scientific Names and Vernacular Names.
Göltenboth, F., and Schoppe, S. (2006). “Mangroves,” in Ecology of Insular Southeast Asia (Elsevier), 187–214. doi:10.1016/b978-044452739-4/50011-5
Hara, J., Frias, J., and Nash, R. (2020). Quantification of Microplastic Ingestion by the Decapod Crustacean Nephrops norvegicus from Irish Waters. Mar. Pollut. Bull. 152, 110905. doi:10.1016/j.marpolbul.2020.110905
Hill, R. W., Wyse, G. A., and Anderson, M. (2004). Animal Physiology, 2. Sunderland, Massachusetts: Sinauer associates Massachusetts
Kallenbach, E. M. F., Hurley, R. R., Lusher, A., and Friberg, N. (2021). Chitinase Digestion for the Analysis of Microplastics in Chitinaceous Organisms Using the Terrestrial Isopod Oniscus asellus L. As a Model Organism. Sci. Total Environ. 786, 147455. doi:10.1016/j.scitotenv.2021.147455
Karami, A., Golieskardi, A., Choo, C. K., Romano, N., Ho, Y. B., and Salamatinia, B. (2017). A High-Performance Protocol for Extraction of Microplastics in Fish. Sci. Total Environ. 578, 485–494. doi:10.1016/j.scitotenv.2016.10.213
Kroon, F., Motti, C., Talbot, S., Sobral, P., and Puotinen, M. (2018). A Workflow for Improving Estimates of Microplastic Contamination in Marine Waters: A Case Study from North-Western Australia. Environ. Pollut. 238, 26–38. doi:10.1016/j.envpol.2018.03.010
Kuss, H.-M. (1992). Applications of Microwave Digestion Technique for Elemental Analyses. Fresenius J. Anal. Chem. 343, 788–793. doi:10.1007/BF00633568
Lamble, K. J., and Hill, S. J. (1998). Microwave Digestion Procedures for Environmental Matrices. Analyst 123, 103–133. doi:10.1039/A800776D
Lusher, A. L., Welden, N. A., Sobral, P., and Cole, M. (2017). Sampling, Isolating and Identifying Microplastics Ingested by Fish and Invertebrates. Anal. Methods 9, 1346–1360. doi:10.1039/C6AY02415G
Miller, M. E., Kroon, F. J., and Motti, C. A. (2017). Recovering Microplastics from Marine Samples: A Review of Current Practices. Mar. Pollut. Bull. 123, 6–18. doi:10.1016/j.marpolbul.2017.08.058
Murray, F., and Cowie, P. R. (2011). Plastic Contamination in the Decapod Crustacean Nephrops norvegicus (Linnaeus, 1758). Mar. Pollut. Bull. 62, 1207–1217. doi:10.1016/j.marpolbul.2011.03.032
Piarulli, S., Scapinello, S., Comandini, P., Magnusson, K., Granberg, M., Wong, J. X. W., et al. (2019). Microplastic in Wild Populations of the Omnivorous Crab Carcinus Aestuarii: A Review and a Regional-Scale Test of Extraction Methods, Including Microfibres. Environ. Pollut. 251, 117–127. doi:10.1016/j.envpol.2019.04.092
Ritter, A., Michel, E., Schmid, M., and Affolter, S. (2004). Interlaboratory Test on Polymers: Determination of Heavy Metals in Polymer Metrices. Polym. Test. 23, 467–474. doi:10.1016/j.polymertesting.2003.09.001
Roy, J. C., Salaün, F., Giraud, S., Ferri, A., Chen, G., and Guan, J. (2017). Solubility of Chitin: Solvents, Solution Behaviors and Their Related Mechanisms. London, United Kingdom: IntechOpen. doi:10.5772/intechopen.71385
Safaie, M. (2015). Population Dynamics for Banana Prawns,Penaeus Merguiensisde Man, 1888 in Coastal Waters off the Northern Part of the Persian Gulf, Iran. Trop. Zool. 28, 9–22. doi:10.1080/03946975.2015.1006459
Sakurai, H., Noro, J., Kawase, A., Fujinami, M., and Oguma, K. (2006). Digestion of Plastic Materials for the Determination of Toxic Metals with a Microwave Oven for Household Use. Anal. Sci. 22, 225–228. doi:10.2116/analsci.22.225
Schindelin, J., Arganda-Carreras, I., Frise, E., Kaynig, V., Longair, M., Pietzsch, T., et al. (2012). Fiji: An Open-Source Platform for Biological-Image Analysis. Nat. Methods 9, 676–682. doi:10.1038/nmeth.2019
Shao, J., Yang, Y., and Zhong, Q. (2003). Studies on Preparation of Oligoglucosamine by Oxidative Degradation under Microwave Irradiation. Polym. Degrad. Stab. 82, 395–398. doi:10.1016/S0141-3910(03)00177-0
Sun, J., Wang, W., and Yue, Q. (2016). Review on Microwave-Matter Interaction Fundamentals and Efficient Microwave-Associated Heating Strategies. Materials 9, 231. doi:10.3390/ma9040231
Therien, J. P. D., Hammerer, F., Friščić, T., and Auclair, K. (2019). Mechanoenzymatic Breakdown of Chitinous Material to N ‐Acetylglucosamine: The Benefits of a Solventless Environment. ChemSusChem 12, 3481–3490. doi:10.1002/cssc.201901310
Thompson, R. C., Olsen, Y., Mitchell, R. P., Davis, A., Rowland, S. J., John, A. W. G., et al. (2004). Lost at Sea: Where Is All the Plastic? Science 304, 838. doi:10.1126/science.1094559
Vance, D. J., and Rothlisberg, P. C. (2020). The biology and ecology of the banana prawns: Penaeus merguiensis de Man and P. indicus H. Milne Edwards. Adv. Mar. Biol. 86, 1–139. doi:10.1016/bs.amb.2020.04.001
Wade, N. M., Bourne, N., and Simon, C. J. (2018). Influence of Marker Particle Size on Nutrient Digestibility Measurements and Particle Movement through the Digestive System of Shrimp. Aquaculture 491, 273–280. doi:10.1016/j.aquaculture.2018.03.039
Wang, Y. J., Jiang, W. X., Zhang, Y. S., Cao, H. Y., Zhang, Y., Chen, X. L., et al. (2019). Structural Insight into Chitin Degradation and Thermostability of a Novel Endochitinase from the Glycoside Hydrolase Family 18. Front. Microbiol. 10, 2457. doi:10.3389/fmicb.2019.02457
Welden, N. A. C., and Cowie, P. R. (2016). Environment and Gut Morphology Influence Microplastic Retention in Langoustine, Nephrops norvegicus. Environ. Pollut. 214, 859–865. doi:10.1016/j.envpol.2016.03.067
Wójcik-Fudalewska, D., Normant-Saremba, M., and Anastácio, P. (2016). Occurrence of Plastic Debris in the Stomach of the Invasive Crab Eriocheir Sinensis. Mar. Pollut. Bull. 113, 306–311. doi:10.1016/j.marpolbul.2016.09.059
Keywords: crustacean, plastic, microwave ovens, seafood, chitin
Citation: Li JYQ, Nankervis L and Dawson AL (2022) Digesting the Indigestible: Microplastic Extraction From Prawn Digestive Tracts. Front. Environ. Chem. 3:903314. doi: 10.3389/fenvc.2022.903314
Received: 24 March 2022; Accepted: 19 May 2022;
Published: 30 June 2022.
Edited by:
Karin Mattsson, University of Gothenburg, SwedenReviewed by:
Kai Zhang, Macau University of Science and Technology, Macao SAR, ChinaMarkus Sillanpää, Finnish Environment Institute (SYKE), Finland
Copyright © 2022 Li, Nankervis and Dawson. This is an open-access article distributed under the terms of the Creative Commons Attribution License (CC BY). The use, distribution or reproduction in other forums is permitted, provided the original author(s) and the copyright owner(s) are credited and that the original publication in this journal is cited, in accordance with accepted academic practice. No use, distribution or reproduction is permitted which does not comply with these terms.
*Correspondence: Joan Y. Q. Li, am9hbi5saUBteS5qY3UuZWR1LmF1