- 1Productive Coasts Lab, Climate Change Cluster, Faculty of Science, University of Technology Sydney, Sydney, NSW, Australia
- 2Estuaries and Catchments Team, Waters Wetland Coastal Science Branch, NSW Department of Planning Industry and Environment, Lidcombe, NSW, Australia
- 3Sydney Institute of Marine Science, Mosman, NSW, Australia
Constructed wetlands (CWs) are an effective means to treat nutrient and sediment pollution in urban stormwater runoff to minimise impact on receiving waterways. Maintenance of devices is recognised as a major contributing factor to performance. There is a lack of evidence-based guidance on maintenance activities to optimise treatment, due to a paucity of data from long-term field studies into CW performance before and after maintenance. In this study, the nutrient and sediment removal efficiency (% RE) of a CW was evaluated by calculating removal efficiencies of nitrogen (N), phosphorus (P) and total suspended sediment (TSS) following a long-term sampling program under baseflow and event flow conditions. Sampling was carried out before, during and after maintenance. Maintenance involved removing all aquatic vegetation and 200–300 mm of sediments over a 3-week period, aiming to improve the wetland’s performance. Assessment of dissolved and particulate nutrient fractions allowed a comprehensive investigation into drivers of nutrient removal efficiency. Under baseflow conditions differences in inflow and outflow pollutant concentrations were used to calculate removal efficiency and pollutant loads were used during event flow conditions. Before maintenance, during baseflow conditions the wetland was removing total N (36% RE) but exporting total P (-52% RE) and total sediment (-94% RE). During event-flow conditions all target pollutants were being removed (total N 63% RE, total P 25% RE and TSS 69% RE). phosphorusDuring maintenance, the device continued to remove total N (18% RE) but the physical disturbance of the maintenance resulted in mass export of total P (-120% RE) and total sediment (−2,000% RE) over a short time period, effectively undoing previous treatment. After maintenance, during baseflow conditions, the wetlands’ ability to treat total N decreased (28% RE), improved for total P (1% RE), and became a chronic source of suspended sediment (−127% RE). During event flow conditions, total N was no longer being treated (−19%) but total P and total suspended sediment were being retained (74%, 80% RE respectively). This study showed that the physical disturbance resulting from large-scale maintenance activities can potentially reverse years’ worth of treatment if not adequately planned and carried out with suitable controls.
Introduction
Diffuse sources of pollution such as urban stormwater runoff have been identified as some of the greatest threats to the health of waterways in Australia and around the world (Burton and Pitt, 2002; NRMMC, 2006; NRC, 2008; BMT WBM, 2017). Widespread urbanisation has had significant effects on runoff and the structure and function of aquatic environments (Walsh C. J. et al., 2005; Walsh et al., 2005; Lee et al., 2006; Wenger et al., 2009; Walsh et al., 2012). Urbanisation impacts water quality and ecosystem health of waterways by altering the hydrology of a natural catchment, increasing peak flow volumes, decreasing runoff response times and decreasing infiltration (Fletcher et al., 2004; Barbosa et al., 2012).
Urban stormwater typically contains relatively high concentrations, and large masses, of nutrients such as nitrogen (N) and phosphorus (P) and other potential contaminants (e.g. toxicants, microbes, litter, microplastics) (Taylor et al., 2005; Francey et al., 2010). Excessive nutrient loads from stormwater are linked with negative ecosystem impacts in downstream receiving waterways (Conley et al., 2009; Yang and Lusk, 2018). Nitrogen and P in urban stormwater exist in dissolved and particulate forms, including dissolved inorganic ions including ammonium (NH4+), nitrite (NO2−), and nitrate (NO3−) (nitrite and nitrate collectively referred to as NOx-N), and phosphate (PO43-), and organic molecules (i.e. dissolved organic N (DON) and P (DOP), and particulate organic N (PN) and P (PP) (Jani et al., 2020). Dissolved inorganic forms of nutrients are linked to negative impacts in downstream receiving waters, causing eutrophication, excessive algal growth and fluctuations in dissolved oxygen concentrations that potentially result in fish kills (Miller and Boulton, 2005; Taylor et al., 2005; Li and Davis, 2014; Lusk and Toor, 2014).
Stormwater control measures (SCMs) are structures designed to mitigate the hydrologic, water quality and ecological consequences associated with urbanisation (Collins et al., 2010; Jefferson et al., 2017; Gold et al., 2019). They are intended to intercept, treat and slowly release urban stormwater runoff; transforming and/or removing pollutants and contaminants by replicating or supplementing a variety of natural processes (Jacobsen et al., 2010; Yang and Toor, 2016; Lopez-Ponnada et al., 2020; Ahammed, 2017; Bell et al., 2017; Rivers et al., 2018).
Constructed wetlands (CWs) are SCMs designed to replicate processes that occur in natural wetlands to effectively remove various pollutants (Hatt et al., 2006; Vymazal, 2007; Lee et al., 2009; Hathaway and Hunt, 2011). CWs are extensively used in Australian and international settings to remove nutrients and sediments from urban stormwater runoff before it enters a receiving waterway (Jing et al., 2001; Greenaway and Woolley, 2003; Wu et al., 2014; Adyel et al., 2016; Adyel et al., 2017; Garcia-Chance et al., 2019).
The effectiveness of CWs at removing nutrient pollution is highly variable (Strecker et al., 2001; Collins et al., 2010; Hathaway and Hunt, 2011) with many contributing factors, including catchment characteristics, age of the urban development, sizing and design characteristics of the wetland and choice of plant species (Carleton et al., 2001; Fletcher et al., 2004; Hijosa-Valsero et al., 2012).
One factor that contributes to poor pollutant removal efficiency in CWs is excessive sediment and organic matter debris clogging outlets. This can artificially raise water levels, inundating vegetation and inhibiting biological and microbial processes that transform and remove nitrogen (Tao et al., 2006). Increased aquatic vegetation diversity has been shown to increase nitrogen removal in laboratory microcosm experiments (Geng et al., 2019), highlighting that if monospecific vegetation stands are allowed to develop in CWs, nutrient removal may be reduced (Maucieri et al., 2020). Decomposing plant and other organic matter debris can release dissolved nutrients back into the water column which is then exported to receiving waters (Chimney and Pietro, 2006). Further, unhindered vegetation growth can potentially cause other issues in CWs, from increased mosquito breeding rates (Greenway and Wooley, 2003) to organic matter export following vegetation die off (Vymazal, 2007).
Many of these issues can be addressed with a well-considered maintenance program. Above-ground plant biomass can accumulate up to 25% of the annual inflowing nitrogen in urban stormwater (Lenhart et al., 2012), highlighting harvesting and removal of vegetation may be an important maintenance consideration for permanent nitrogen removal (Blecken et al., 2017). Removing debris from outlet drains to prevent rising water levels may also maintain or improve treatment effectiveness (Hunt et al., 2011).
Appropriate maintenance of CWs and other SCMs is considered a key issue by waterway managers (e.g. CCC, 2007; NCC, 2012; LMCC, 2013; BCC, 2019; Stormwater NSW, 2020). Despite this, evidence-based guidance on the scale, frequency and type of maintenance operations to maintain optimal performance is lacking. Information about how the effectiveness of SCMs responds to different maintenance operations would enable environmental managers to make more informed decisions about timing, scale and types of maintenance operations required for different types of treatment infrastructure, leading to streamlined and more cost-effective maintenance strategies.
Typically, field-based SCM monitoring and assessment studies are carried out under one condition only, or, laboratory/mesocosm studies have been carried out to highlight differences in condition in controlled settings. Full scale field-based studies assessing CW pollutant removal efficiency over the long-term are uncommon (Vymazal et al., 2021). Furthermore, field-scale studies showing changes in efficiency in response to maintenance as an experimental intervention are rare but provide useful insight for waterway managers when developing maintenance strategies to optimise performance.
Our study addresses the question of optimal maintenance using a field-based case study to quantify the nutrient and sediment removal efficiency of a CW before, during and after large scale maintenance activities. The manager of the CW had concerns that the wetland may be performing poorly due to excessive sediment deposition and prolific plant and algal growth, and that this had implications for downstream receiving waters. Sediment build up in the inlet zone and decaying vegetation at the outlet impeded flow through the wetland and increased the water level, leading to inundation and water logging of emergent and riparian vegetation.
The objectives of this study were to compare pollutant removal efficiency of the CW: 1) when full of mature vegetation and sediments before the maintenance activity; 2) during the maintenance activity itself, and; 3) when the vegetation had been removed after the maintenance activity. We expected the nutrient and suspended sediment removal efficiency to vary greatly between sampling stages; before maintenance the CW would be most effective due to the role of plant biomass in assimilating dissolved inorganic nutrients and the organic matter enriched bed sediments facilitating removal of dissolved inorganic N through denitrification, and the complexity of the vegetated area to trap and store sediment; during the maintenance operations the physical disturbance of the activities would result in the unplanned export of sediment and organic matter, and; after maintenance the wetland would not effectively remove N or P, given the lack of plant biomass and enriched sediment available for dissolved nutrient uptake and denitrification, and sediment would be continually exported given the reduced complexity and the resuspension of bed sediments into the water column. Efficiency was assessed by quantifying changes in effluent nutrient and sediment concentrations, event mean concentrations and loads before and after removal of organic matter biomass from the wetland.
Materials and Methods
Study Site
The approximately 10-year-old, horizontal surface flow CW in this study is in Macquarie Hills, NSW, Australia (32.95o S, 151.65o W). The area has a warm temperate climate with annual precipitation averaging approximately 1,100 mm (Bureau of Meterology station 061393, 2022). The wetland is approximately 250 m long and receives rainfall-runoff from a well-established urban residential sub-catchment (65 hectare; 58% of the catchment area is impervious surface) via a single inlet drain. The device has no high-flow bypass and responds quickly to rainfall events, with runoff entering the device shortly after rainfall commences. Inflowing runoff enters the wetland system through two 900 mm concrete pipes discharging directly to a low flow sediment basin which is bypassed during large flows by a diverting weir. A trash rack removes gross pollutants before runoff enters a long, narrow, shallow, densely vegetated zone which then opens into a large and deep (1.5–2 m) open water area with dense fringing emergent and riparian vegetation, before flowing out to a major tributary of a downstream estuary (Lake Macquarie) through a 1800 mm × 1200 mm concrete box culvert with a second trash rack (Figure 1).
Experimental Design
The study used programmed maintenance activities as an experimental intervention to investigate effects of disturbance on nutrient removal efficiencies. Maintenance involved harvesting all aquatic vegetation and between 200 and 300 mm of bed sediments from the shallow vegetated zone and open water zone with an amphibious excavator (Truxor DM5000).
We examined measures of pollutant removal efficiency in 3 time periods;
1. before maintenance (February 2014–November 2014).
2. during maintenance (February 2015–austral summer).
3. after maintenance (March 2015–December 2015).
Flow Velocity Measurements
Pressure sensors installed in the fixed cross sections of the wetland inlet and outlet drain works were used to continuously measure stage height by barometric compensation. Flow velocity measurements were carried out opportunistically across a range of different sized rainfall events by deploying an acoustic doppler current meter (Micronics Stingray) during large rainfall events and a water velocity meter (Xylem Global Water Flow) during low flow conditions at both the inlet and outlet. Discharge was calculated using the velocity area equation (Q = AV) to establish contemporary stage-discharge rating tables at both the inlet and outlet, allowing for a continuous 15-min discharge timeseries. During dry weather the stage height was minimal to zero, below the limit of detection of the pressure sensors and flow velocity equipment meaning reliable stage and flow data could not be collected. During these conditions unreliable data was discarded, and discharge recorded as less than detection limits. This resulted in no discharge data being available during baseflow conditions.
Water Sample Collection
Before and After maintenance: During baseflow conditions (defined as 0 mm of rainfall for a minimum of 7 consecutive days), water samples were collected manually at the inlet and the outlet of the wetland at intervals of not more than 14 days. ISCO 6600 automated samplers were used to collect samples during events. These samplers had a liquid-level actuator to trigger sample collection and were fitted with 24 clean PET sample bottles. Samplers were installed at the inlet and outlet to collect samples as stage height increased throughout rainfall events. A pilot study was done to determine the most suitable sampling interval for the autosamplers to enable optimal data resolution for a typical event. Based on this, autosamplers were programmed to collect samples across the hydrograph at 15-min intervals once triggered.
During Maintenance: All sampling equipment had to be removed during the maintenance period to avoid interference from the excavator. During the maintenance period, grab samples were collected manually from the inlet and outlet.
All sample collection times were recorded to the minute to allow for calculation of flow volume between sampling intervals, allowing calculation of flow-weighted data.
Sample Processing and Analytical Methods
Samples were retrieved from autosamplers within 16 h of a rainfall event and kept cool. Prior to processing, water level data were examined and the individual bottles that represented phases of the event hydrograph (first flush, peak flow, receding limb) were identified (e.g. the first x samples may have been during the “first flush” phase; and the next y during the “peak flow” etc.). Composite samples were made by combining the individual sample bottles that represent a phase of the event hydrograph in a prewashed stainless steel 10L bucket. This process was repeated in separate buckets for each phase. The resulting composite sample for a phase was sub-sampled for a range of variables. The steps of sub-sampling were to use a 60 ml disposable syringe to transfer 28 ml of unfiltered sample water into a clean vial for analysis of total nutrients; then two additional 28 ml water samples were passed through a 0.45 µm cellulose acetate syringe filter into vials for analysis of dissolved nutrients (dissolved inorganic, total dissolved). All nutrient samples were immediately frozen after collection (−20 C) until analysed. The composite sample was also subsampled for total suspended solids (TSS) by vigorously mixing the remaining sample and pouring into a 1L clean PET bottle which was stored in a refrigerator until analysis.
Relevant samples were analysed for oxidised N (NOx-N), ammonia N (NH4+), soluble reactive phosphate (SRP/PO43-), total dissolved P (TDP), total P (TP), total dissolved N (TDN), and total N (TN). Nutrient analyses were carried out by flow injection analysis (LachatTM QuikChem 8500 Flow Injection Analyser) following standard methods (APHA, 2005). Freshwater reference standards and internal standards were used for quality assurance. Oxidised N [NOx-N = Nitrate (NO3) + (NO2)] was determined using a filtered sample passed through a cadmium column to reduce nitrate to nitrite. The nitrite (originally in the sample and reduced nitrate) was determined by diazotizing with sulfanilamide and coupling with N-(1-naphthyl)-ethylenediamine dihydrochloride to form a highly coloured azo dye, which is measured by colorimetric method. For nitrite alone, the procedure is the same except the cadmium column was bypassed. Ammonia N (NH4+) was determined using the hypochlorite/phenate method and dissolved inorganic N (DIN) was estimated as the sum of NOx-N and NH4+. Soluble reactive phosphate (PO43-) was determined by ascorbic acid colorimetric method with FIA. Total and total dissolved nutrients were determined by dispensing samples into polycarbonate tubes dosed with alkaline persulfate solution, capped and digested in an autoclave at 121°C for 1 h. The alkaline persulfate digestion procedure oxidizes all forms of inorganic and organic-N to nitrate and hydrolyzes all forms of inorganic and organic-P to orthophosphate. The nitrate and orthophosphate in the digested samples were measured colorimetrically. Dissolved organic N (DON) was calculated as TDN minus DIN and dissolved organic P (DOP) was calculated as TDP minus phosphate.
In the laboratory, TSS samples were vacuum filtered through pre-washed, pre-ashed 47 mm GF/F filters at room temperature. Filters were dried at 105°C and weighed on a 4-decimal place Metler Toldeo level-balance. Sample salinity was measured before filtering, so that sample volume could be calculated based on difference in weight of sample bottles before and after filtering assuming water density of 1 g/ml.
Data Analysis
Where discharge data were available, flow-weighted event mean concentrations (EMC) of each pollutant were calculated by dividing the total pollutant mass load by the total event flow volume per rainfall event. Pollutant mass (load) was calculated using the measured interval pollutant concentrations and flow volumes within each rainfall event. Sum pollutant loads were then calculated for the inlet and outlet for each sampling period. Proportions of each dissolved and particulate fraction were determined for total N and total P. Removal efficiency calculation.
Overall removal efficiency of the CW was based on the percentage difference between pollutants flowing in and out of the wetland, in accordance with best practice guidance for assessing performance of SCMs in Australia (Stormwater Australia, 2018). Long term removal efficiency (RE%) (Equation 1) was calculated using average pollutant concentrations during baseflow conditions before, during and after maintenance, and pollutant loads during event conditions. Negative removal efficiency occurs when pollutant concentrations are greater at the outlet than the inlet, indicating net pollutant export from the CW and positive removal efficiency indicates net pollutant removal, treatment or retention by the CW.
Equation 1: Pollutant removal efficiency equation in % removal. RE% = removal efficiency. Calculated using pollutant concentrations during baseflow and pollutant loads during event flow.
Statistical Analysis
A one-way ANOVA was used to analyse whether the average pollutant concentrations were significantly different between the inlet and outlet during baseflow conditions, for each sampling period. Data were log transformed prior to all analyses to meet the assumptions of ANOVA. An analysis of the influence of season on RE% was not possible due to uneven data in different seasons.
Results
Baseflow Conditions
Nineteen samples were collected during baseflow conditions before maintenance. Dissolved inorganic N made up the greatest average proportion of the total N pool in the inflowing stormwater. The wetland was efficiently removing total N (36% RE) (Table 1). This was being driven by treatment of dissolved inorganic forms of N (NH4+ 51% RE, and NOx-N 94% RE). However organic and particulate forms of N were being exported (DON -11% RE and PN -70% RE), highlighting that before maintenance not all forms of N were being actively treated or retained (Figure 2). Refractory dissolved organic N was the dominant form of N in the outflow. The CW was a source of total P before maintenance (Table 1; Figure 3). There was a large removal of dissolved inorganic P (phosphate) (33% RE), but export of organic and particulate P (DOP -22% RE and particulate P -135% RE). Particulate P was the dominant form of the inflowing and outflowing total P, the ongoing export resulting in no effective treatment of total P (-52% RE). TSS was not being removed, instead the CW was a chronic source of suspended sediment to downstream receiving waters (-94% RE) (Table 1; Figure 4).
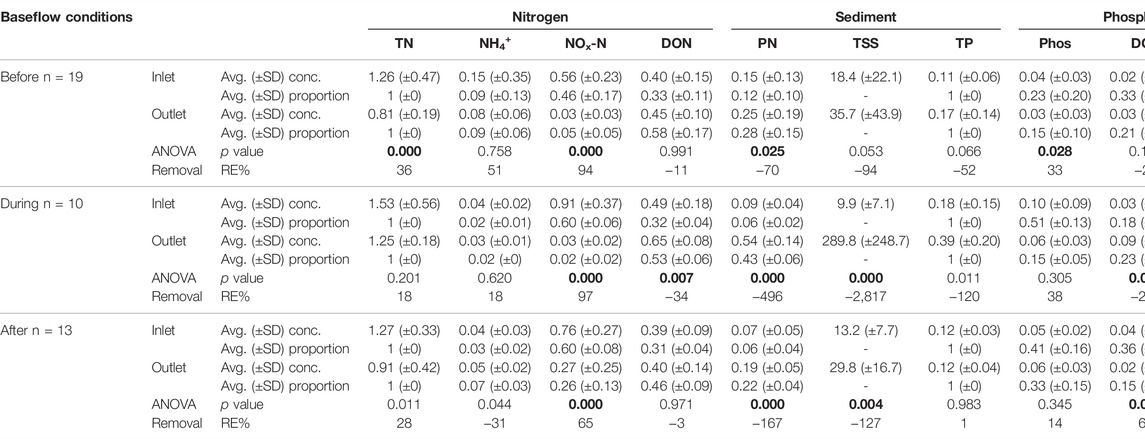
TABLE 1. Average (± standard deviation) pollutant concentrations (mg L−1) and average (± standard deviation) proportion of constituent fractions and overall removal efficiency (%) during baseflow conditions in the study CW. p value = probability level of 1-way ANOVA comparing average event mean concentrations between inlet and outlet. Significant differences in bold.
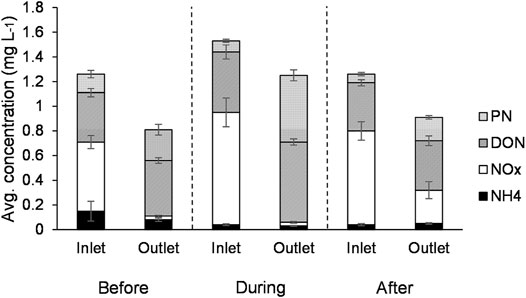
FIGURE 2. Baseflow average (SE) nitrogen concentration at the CW inlet and outlet, before, during and after maintenance.
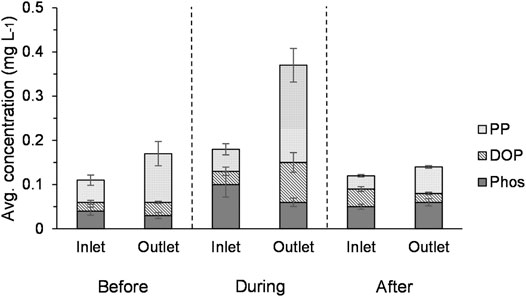
FIGURE 3. Baseflow average (SE) phosphorous concentration at the CW inlet and outlet, before, during and after maintenance.
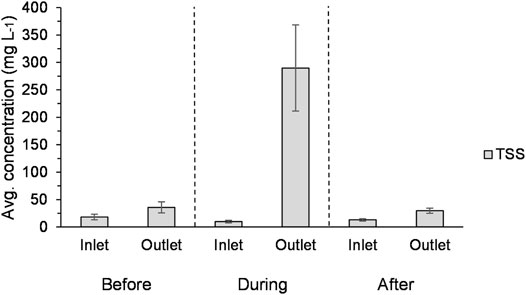
FIGURE 4. Baseflow average (SE) TSS concentration at the CW inlet and outlet, before, during and after maintenance.
During the vegetation and associated sediment removal, ten samples were collected a minimum of 2 days apart to obtain independent samples. Dissolved inorganic N (NOx-N) was the dominant form of N in the inflow, and dissolved inorganic P was the dominant P form. The treatment of N and P was similar to before maintenance (Table 1); total N was being removed (18% RE), driven by dissolved inorganic N removal (NH4+ 18% RE, and NOx-N 97% RE) but organic and particulate forms were being exported (DON -34% RE and particulate N -496% RE) (Figure 2). Total P was being exported (-120% RE) as the effective removal of dissolved inorganic P (phosphate) (38% RE) was countered by mass export of organic and particulate P (DOP -212% RE and particulate P -336% RE) (Table 1; Figure 3). Dissolved organic N was the dominant form of N and particulate P was the dominant P form in the outflow. There was nearly a 30-fold increase in average TSS concentration in the outflow from the wetland during the maintenance, yielding a removal efficiency of −2,817% (Table 1; Figure 4). This indicates the disturbance of the vegetation and bed sediment removal resulted in massive export of long-stored sediment, organic material and particulate nutrients into the receiving waterway downstream. Export concentrations of this magnitude were not observed during the much longer sampling periods before and after maintenance, indicating a disproportionately large export of material in a much shorter timeframe during the maintenance operation.
Sampling recommenced in mid-March 2015 14 days after the maintenance was completed. Thirteen samples were collected during baseflow conditions after maintenance. In this period, dissolved inorganic N (NOx-N) and P (phosphate) again comprised the greatest proportion of total N and total P respectively. After maintenance with almost all plant biomass and 200–300 mm of organic rich bed sediments removed, the treatment of total N decreased slightly from 36 to 28% RE (Table 1). There were larger scale changesin the dissolved and particulate fractions, driving the overall N removal efficiency (Figure 2). Removal of NOx-N decreased from 94 to 65% RE, likely due to the lack of plants and bed sediments facilitating various pathways of removal and transformation (e.g., assimilation, denitrification in anaerobic sediments, provision of root and rhizome substrate for microbial processing). NH4+ was being exported from the wetland (−31% RE), likely due to flux from residual decaying organic matter in the wetland. Organic and particulate forms continued to be exported from the CW, with particulate N export increasing when compared to before maintenance (DON -3% RE and particulate N -167% RE). In light of this, the ongoing effective treatment of NOx-N continued to drive overall removal of total N after maintenance (Table 1). After maintenance, the CW showed improved (but limited) treatment of total P (after maintenance 1% RE) (Table 1). This improvement was driven by an increase in dissolved organic P removal (61% RE) (Figure 3). After maintenance the CW’s ability to remove dissolved inorganic P (phosphate) decreased (14%), and there was ongoing export of particulate P (−121% RE). Similar to before and during maintenance, particulate P made up the greatest proportion of the outflow total P. The CW also remained a chronic source of sediment to downstream receiving waters during baseflow conditions after maintenance, with the export of material increasing compared to before maintenance, decreasing the removal efficiency even further (−127% RE) Table 1 and Figure 4).
Event Flow Conditions
Fifteen rainfall events were sampled over 10 months before maintenance. 178.0 kg of total N and 19.0 kg of total P cumulatively entered the CW during this phase. Dissolved inorganic forms (NOx-N and phosphate) made up the largest proportion of the inflowing N and P load. At this time, the CW was removing total N and total P and all dissolved and particulate fractions (Table 2). The wetland exported 65.9 kg of total N and 14.2 kg of total-P, giving RE% of 63% total N and 25% total P. Removal of total N was being driven by treatment of NOx-N (84% RE) and P removal by particulate phosphorus retention (24% RE). Refractory DON was the largest proportion of the total N export load, highlighting the CWs strong capacity to treat dissolved inorganic N when fully vegetated (Figure 5). The largest proportion of export total P was phosphate, which had limited treatment at 5% RE. This highlights during event flow conditions there is minimal assimilation of dissolved inorganic P and overall treatment of P is likely due to adsorption to sediment, evidenced by the high removal of particulate P (Figure 6). The CW received over 9,000 kg of inflowing total sediment before maintenance. Most was retained, with over 2,800 kg exported, giving 69% RE (Table 2; Figure 7).
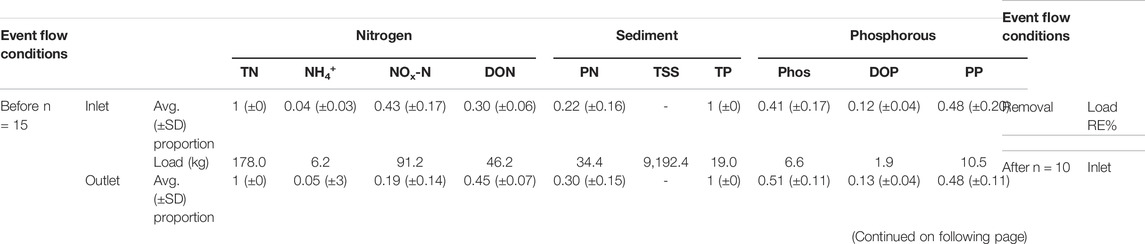
TABLE 2. Pollutant load and average (± standard deviation) proportion of constituent fractions and overall removal efficiency (%) during event flow conditions in the study constructed wetland.
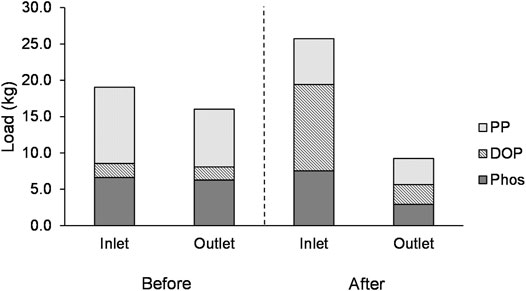
FIGURE 6. Event flow sum phosphorous loads at the CW inlet and outlet, before and after maintenance.
Ten rainfall events were sampled over 10 months after maintenance. Overall, the CW received 42.3 kg of total N during this time (Table 2). Dissolved inorganic N (NOx-N) made up the largest proportion of the inflowing N load. Removal of the vegetation and sediments disrupted N treatment. After maintenance the CW was a source of N, exporting 50.5 kg (−19% RE), driven by export of NOx-N (−7% RE), DON (−75% RE) and particulate N (−14%) (Table 2). Dissolved organic N was the dominant form of the N export load, followed closely by NOx-N. Before maintenance, the proportion of NOx-N in the outflowing treated stormwater was 19% of the total N load. After maintenance the proportion of NOx-N in the outflow increased to 35%. This highlights the role of vegetation and organic laden anaerobic sediments in transforming and removing bioavailable forms of N in the CW and that in this condition following maintenance, greater loads of bioavailable forms of N are exported to receiving waterways (Figure 5). The CW received 25.7 kg of inflow total P, the dominant forms being dissolved organic P. 6.7 kg of total P was exported, giving 74% RE—a large improvement from the 25% RE before maintenance (Table 2). Treatment of DOP (77% RE) and phosphate (61% RE) were the main drivers (Figure 6). Retention of particulate P also improved to 43% RE. The CW, cleared of all vegetation and associated bed sediments, was acting as a sedimentation pond during rainfall events, with inflowing sediment loads being retained at a greater rate than before maintenance (Figure 7). Over 5,600 kg of sediment entered the wetland, and over 1,100 kg was exported, giving 80% RE (Table 2).
Discussion
Before maintenance, under baseflow conditions the wetland was removing bioavailable forms of N and P but exporting organic and particulate forms. Sediment was also being exported. During event flow conditions the CW was actively treating all target pollutants, with export loads smaller than inflow loads. The removal efficiency of total N before maintenance is within the removal range of 40–55% reported in global comparisons of constructed wetlands under baseflow conditions, but was greater than this under event flow conditions (Vymazal, 2007). This suggests the initial perceptions of the CW manager that the ability of the CW to treat urban stormwater had declined were incorrect. Vegetation and bed sediment removal as a maintenance activity had major changes on nutrient and sediment treatment dynamics. The maintenance disrupted N treatment, but arguably improved P treatment (Figure 8).
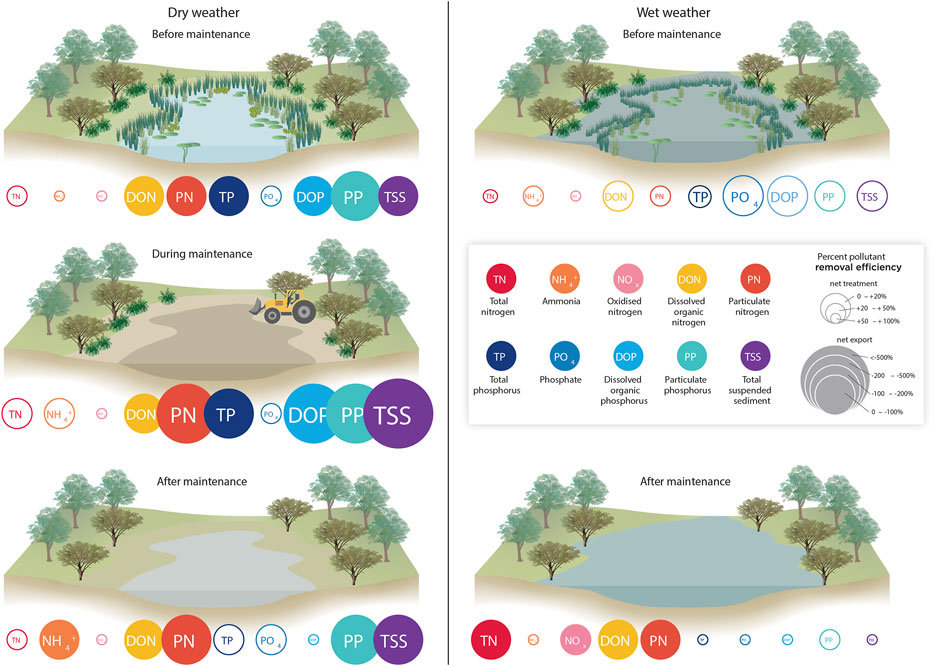
FIGURE 8. Treatment dynamics of nitrogen, phosphorous and total sediment in the study CW before, during and after maintenance, during baseflow and event flow conditions.
The export and the inability of the CW to remove dissolved inorganic-N after maintenance is consistent with one of the long-held fundamental concepts of CW design i.e. plant biomass treats dissolved nutrients. Regardless of flow condition or vegetated state, the most abundant form of N in the inflowing stormwater is consistently NOx-N, which aligns with findings in reviews of stormwater composition (Taylor et al., 2005; Francey et al., 2010). With the exception of event flow conditions after the vegetation had been removed, the removal efficiency of NOx-N was always the greatest of the total N constituent fractions, making a large relative contribution to the removal of total N. The removal of the vegetation and sediments likely had multiple effects on the wetland’s ability to remove dissolved inorganic N. The relatively high and persistent NOx-N treatment suggests that the major processes of N removal in the constructed wetland may be related to both microbial activity in the sediment plant interface and direct assimilation by aquatic plants (Saunders and Kalff, 2001; Vymazal, 2007; Griffiths and Mitsch, 2017).
Plants are typically considered the major contributor to nutrient treatment in constructed wetlands by assimilation of nutrients through their roots (Zhu et al., 2021) and incorporation into plant biomass. Additionally, a significant N removal pathway in constructed wetlands is coupled nitrification/denitrification, mediated by microbial activity in anaerobic sediments (Erler et al., 2008; Payne et al., 2014; Tan et al., 2017; Gold et al., 2019; Zhu et al., 2021). In older constructed wetlands like in this study, the rate at which N is removed from urban stormwater increases as rates of denitrification increase with increased organic carbon loading in the sediments (Lin et al., 2002; Lee et al., 2009; Griffiths and Mitsch, 2017), and aquatic vegetation establishes and biomass increases over time (Abbasi et al., 2019). The rhizomes of submerged plants can provide a source of organic carbon and nutrients for microbes that live in plant surface biofilms, meaning the plants themselves drive denitrification in the anoxic environment of the plant-sediment interface, not just a process mediated by organic rich sediments (Ruiz-Rueda et al., 2009; Adrados et al., 2014; Hua et al., 2017). Furthermore, greater abundances of denitrifying bacteria have been detected in sediments around plant rhizomes compared to bare sediments in natural and constructed wetlands (Hallin et al., 2015). This highlights a strong coupling between two critical N removal processes mediated by the presence of vegetation and the sediments they interact with. Removing the vegetation and a layer of bed sediment therefore reduces the capacity for overall effective treatment of N, resulting in the export of bioavailable forms of N under both baseflow (NH4+) and event flow conditions (NOx-N).
Contradicting expectations, the P removal efficiency improved after maintenance, under both flow conditions, with total P and all dissolved and particulate forms being treated or retained, except for particulate P under baseflow. Compared to N, it is accepted that effective P treatment in urban stormwater is challenging and highly variable (Hunt et al., 2006; Maucieri et al., 2020). Some studies have highlighted that dissolved forms of P (i.e. phosphate) can be removed by direct uptake by plants, but this is largely species dependent (Perner et al., 2006; Shan et al., 2011; Maucieri et al., 2020). Other studies have highlighted that adsorption of dissolved P to the substrate material is the most effective means of removal (Brooks et al., 2000; Vymazal, 2007; Ballantine and Tanner, 2010). The maintenance activity removed 200–300 mm of bed sediments in addition to removing the vegetation, meaning the substrate in the wetland may have been completely altered, encouraging P retention. Without targeted research to directly assess P removal processes, it is difficult to attribute the improved removal to any one factor from sampling P concentrations alone. However the fate of organic and particulate P was tightly coupled to the fate of sediment under both flow conditions, before and after maintenance. Regardless of flow conditions or vegetated state, if suspended sediment is being retained, so is total P, and if suspended sediment is being exported so is total P, which is commonly observed in wetlands (Rogers et al., 1991; Noe et al., 2007; Parker, 2010; Yu et al., 2012). P removal may be linked to physical processes like sedimentation rather than algal or plant uptake (Nguyen et al., 2020). The higher export (i.e., lower removal efficiency) of suspended sediment and particulate nutrients during low flow conditions compared to retention during high flow conditions may be related to the newly deposited material being easily and readily exported from the wetland under low energy following initial settling and storage during rainfall events. Total P removal often decreases with age as wetlands become shallower with sedimentation, and phosphorus loaded sediments are more prone to resuspension and easily exported in low flow conditions (Mitsch et al., 2012; Mitsch et al., 2014; Mitsch and Gosselink, 2015; Griffiths and Mitsch, 2017).
Sediment export during baseflow conditions remained an issue after maintenance, however removal efficiency of sediment during event flow conditions improved after maintenance. After maintenance, the wetland was functionally similar to a sedimentation basin, which by design has high sediment storage capacity (Parker, 2010; Water By Design, 2010). While this was a benefit, it was offset by the continuous export of sediment during the more frequent baseflow conditions, makes any benefit negligible as continuous export places ongoing pressure on receiving environments downstream by reducing light availability to both benthic and pelagic primary producers, impacting productivity (Cloern et al., 2014).
Despite the improvements in some pollutant removal efficiency after maintenance, the physical disturbance resulted in a mass export of suspended sediment, particulate-N and particulate-P that would have been deposited over many years. This was the first significant maintenance operation on the wetland in its >10-years lifecycle. This essentially erased the benefit of several years’ worth of treatment in a 3-week period. There was an almost 30-fold increase in sediment export based on differences in total sediment concentrations, which ultimately was transported to the nearby estuarine receiving environment. This was coupled with very large export of organic matter in the form of particulate nutrients, which had the potential to be remineralised as dissolved forms in the receiving waterway (Nixon, 1981). Increased turbidity and sedimentation can cause many issues in shallow estuarine areas (Lohrer et al., 2004; Duarte, 2007; Davis et al., 2016), and organic matter enrichment can contribute to eutrophication (Hallett et al., 2019). This emphasises the need for careful planning of maintenance activities and suitable controls to minimise risk to downstream receiving environments.
In controlled settings, harvesting of submerged macrophytes has been used an effective means of maintaining constructed wetlands when carried out at an appropriate frequency and manner. Verhofstad et al. (2017) found that intermediate harvesting frequency of 2–3 times per year is appropriate when aiming to remove nutrients in a low nutrient loading scenario, as well as encouraging macrophyte cover, height and species abundance in recovery. In contrast, annual plant harvesting has also been found to significantly improve the effectiveness of CWs because harvesting plants not only directly removes nutrient and organic matter standing stocks, but also encourages the breakdown of organic matter, N and P by improving the oxygen and light conditions (Luo, et al., 2018; Wang et al., 2015). These macrocosm based studies, however, cannot account for the practical realities of harvesting full scale CWs, such as the large exports that occurred during the maintenance process. This calls into question whether the outcomes of lab-based studies can be scaled up to enhance understanding of field-scale processes, without proper consideration of practical constraints. Full-field based, whole of system studies like ours provide valuable, novel insight into the real life operation and processes occurring in CWs, and outcomes from both settings need to be considered when planning maintenance strategies.
Investigations to assess SCM effectiveness are increasingly common, yet there are gaps in our understanding of how different processes and removal and transformation pathways vary temporally (including seasonally), spatially, in response to rainfall and among interactions of different pollutants, such as N and P (Payne et al., 2014; Gold et al., 2019; Williams, et al., 2013; Duan et al., 2016). Understanding the nutrient cycling processes and removal pathways within CWs is arguably much more valuable, as it highlights why removal efficiencies identified in traditional effectiveness studies are occurring. The results of this study highlight the importance of investigating and acknowledging that the constituent fractions of N and P are responding differently to management and flow. This argues against the value of a reliance on total nutrient concentrations and loads for removal efficiency assessments. An overall assessment of a wetland might find that there was (hypothetically) a net removal of total N and net production of total P, with the mechanisms, however, being quite different. Dissolved N and P were being removed while outflowing particulate N and P concentrations were greater than inflowing concentrations, suggesting organic and particulate material was being exported as part of the total suspended material pool. In the case of P, the magnitude of the increase in particulate P was sufficient to overwhelm the removal of dissolved P. This means that the removal efficiency picture is quite different for different classes of constituents and that simple statements about overall efficiencies are missing much of the ecologically important information. Dissolved nutrients are readily bioavailable (Howarth and Marino, 2006) so if algal growth in receiving waters is of concern, then this is the most important perspective. Particulate nutrients may be a concern if they are in a labile form which might lead to oxygen reduction via organic loading (Lusk et al., 2020), but if they are largely refractory, they may be largely irrelevant. Similarly, dissolved organic forms are suggested to be refractory (e.g., humic and tannic acids; Neff et al., 2003; Fan et al., 2017), though in some circumstances they may also be considered labile (Jani et al., 2020; Lusk et al., 2020) and influence nutrient and organic matter cycling in receiving waterways (Howarth and Marino, 2006; Li et al., 2019; Garzon-Garcia et al., 2021).
Transformations and removal of dissolved and particulate forms, especially in relation to dissolved inorganic nitrogen as a proportion of N, are the drivers of total N and P removal in CWs. Improved understanding on the removal efficiency of these fractions can inform managers of how current and past conditions in CWs (vegetation cover, hydraulic residence time, water level, etc.) are enabling transformation and removal processes to occur that will be reflected in total N and P concentrations and loads. Furthermore, managers should be designing SCMs and planning maintenance operations that functionally enhance transformation and removal processes of dissolved and particulate nutrient fractions, to enhance overall removal efficiency. This understanding then needs to transfer to an analysis of the marketing material for proprietary products—so that end users can properly assess whether a product is suitable for their objectives.
Conclusion
Mechanical removal of all aquatic vegetation and underlying sediments caused significant physical disturbance, resulting in mass export of sediment and particulate nutrients, possibly reversing many years’ worth of retention in a 3-week period. After maintenance, the wetland was functionally similar to a sediment basin, storing sediment during rainfall events, but continuous export of resuspended sediment occurred during low flow conditions. The fate of P in the wetland followed trends in sediment. Without vegetation, the wetland’s ability to treat N diminished. The results of this study highlight that improper maintenance approaches have the potential to cause more damage than benefit, and how evidence-based advice on potential impacts is needed ahead of maintenance operations. It also highlighted that field-scale studies such as this can yield a wider range of findings when assessing pollutant removal rates of SCMs; laboratory based controlled experiments targeting specific removal rates of plants or microbial processes may not highlight whole of system processes that are occurring in operational devices. Maintenance operations of this scale and timing need careful planning and controls to minimise negative impact, and consideration of whether the benefit to the devices’ performance outweighs the risks to the receiving environment if sediment, nutrients and other pollutants are exported during the process.
Data Availability Statement
The raw data supporting the conclusion of this article will be made available by the authors, without undue reservation.
Author Contributions
AW designed the sampling program, carried out sample collection and analysis, summarised, analysed and interpreted data, and prepared the draft manuscript. MD assisted data interpretation, preparing manuscript and provided review comment. PS assisted data interpretation, preparing manuscript and provided review comment.
Funding
Funding was provided by Lake Macquarie City Council and supported by the Water, Wetlands, Coast Science Branch of NSW DPE.
Conflict of Interest
The authors declare that the research was conducted in the absence of any commercial or financial relationships that could be construed as a potential conflict of interest.
Publisher’s Note
All claims expressed in this article are solely those of the authors and do not necessarily represent those of their affiliated organizations, or those of the publisher, the editors and the reviewers. Any product that may be evaluated in this article, or claim that may be made by its manufacturer, is not guaranteed or endorsed by the publisher.
Acknowledgments
Field sampling and sample analysis could not have been completed without tireless effort from Chris Baiada and Michael Sutherland of the Estuaries and Catchments Team. Analytical advice and endless moral support was provided by Callum Muir, Raissa Gill, Giselle Firme and Mat Windhagauer of the COAST lab, UTS. Symon Walpole and Jason Parsons from LMCC provided much direction and local insight. Mary-Louise Wright provided space, time and support.
References
Abbasi, H. N., Xie, J., Hussain, S. I., and Lu, X. (2019). Nutrient Removal in Hybrid Constructed Wetlands: Spatial-Seasonal Variation and the Effect of Vegetation. Water Sci. Tech. 79 (10), 1985–1994. doi:10.2166/wst.2019.196
Adrados, B., Sánchez, O., Arias, C. A., Becares, E., Garrido, L., Mas, J., et al. (2014). Microbial Communities from Different Types of Natural Wastewater Treatment Systems: Vertical and Horizontal Flow Constructed Wetlands and Biofilters. Water Res. 55, 304–312. doi:10.1016/j.watres.2014.02.011
Adyel, T. M., Oldham, C. E., and Hipsey, M. R. (2017). Storm Event-Scale Nutrient Attenuation in Constructed Wetlands Experiencing a Mediterranean Climate: a Comparison of a Surface Flow and Hybrid Surface-Subsurface Flow System. Sci. Total Environ. 598, 1001–1014. doi:10.1016/j.scitotenv.2017.04.044
Adyel, T. M., Oldham, C. E., and Hipsey, M. R. (2016). Stormwater Nutrient Attenuation in a Constructed Wetland with Alternating Surface and Subsurface Flow Pathways: Event to Annual Dynamics. Water Res. 107, 66–82. doi:10.1016/j.watres.2016.10.005
Ahammed, F. (2017). A Review of Water Sensitive Urban Design Technologies and Practices for Sustainable Stormwater Management. Sust. Water Resour. Manag. 3, 269–282. doi:10.1007/s40899-017-0093-8
APHA (2005). Standard Methods for the Examination of Water and Wastewater. 21st Edition. Washington DC, USA: American Public Health Association/American Water Works Association/Water Environment Federation.
Ballantine, D. J., and Tanner, C. C. (2010). Substrate and Filter Materials to Enhance Phosphorus Removal in Constructed Wetlands Treating Diffuse Farm Runoff: a Review. New Zealand J. Agric. Res. 53 (1), 71–95. doi:10.1080/00288231003685843
Barbosa, A. E., Fernandes, J. N., and David, L. M. (2012). Key Issues for Urban Stormwater Management. Water Res. 46 (20), 6787–6798. doi:10.1016/j.watres.2012.05.029
Bell, C. D., McMillan, S. K., Clinton, S. M., and Jefferson, A. J. (2017). Characterising the Effects of Stormwater Mitigation on Nutrient export and Stream Concentrations. Environ. Manage. 59, 604–618. doi:10.1007/s00267-016-0801-4
Blacktown City Council (2019). Water Sensitive Urban Design Inspection and Maintenance Guidelines. Blacktown, NSW, Australia: Blacktown City Council.
Blecken, G-T., Hunt, W. F., Al-Rubaei, A. M., Viklander, M., and Lord, W. G. (2017). Stormwater Controle Measure (SCM) Maintenance Considerations to Ensure Designed Functionality. Urban Water J. 14 (3), 278–290. doi:10.1080/1573062x.2015.1111913
BMT WBM (2017). New South Wales Marine Estate Threat and Risk Assessment Final Report. Broadmeadow NSW, Australia: BMT WBM Pty Ltd.
Brooks, A. S., Rozenwald, M. N., Geohring, L. D., Lion, L. W., and Steenhuis, T. S. (2000). Phosphorus Removal by Wollastonite: a Constructed Wetland Substrate. Ecol. Eng. 15 (1), 121–132. doi:10.1016/s0925-8574(99)00056-7
Bureau of Meterology station 061393 (2022). (Edgeworth WWTP). Available at http://www.bom.gov.au/jsp/ncc/cdio/weatherData/av?p_nccObsCode=136&p_display_type=dailyDataFile&p_startYear=2019&p_c=-753822857&p_stn_num=061393 (Accessed February 25, 2022).
Burton, G. A., and Pitt, R. E. (2002). Stormwater Effects Handbook: A Toolbox for Watershed Managers, Scientists and Engineers. Boca Raton MI, USA: Lewis Publishers, CRC Press.
Carleton, J. N., Grizzard, T. J., Godrej, A. N., and Post, H. E. (2001). Factors Affecting the Performance of Stormwater Treatment Wetlands. Water Res. 35 (6), 1552–1562. doi:10.1016/s0043-1354(00)00416-4
Central Coast Council (2007). Water Cycle Management Guidelines. Gosford, NSW, Australia: Central Coast Council.
Chimney, M. J., and Pietro, K. C. (2006). Decomposition of Macrophyte Litter in a Subtropical Constructed Wetland in South Florida (USA). Ecol. Eng. 27 (4), 301–321. doi:10.1016/j.ecoleng.2006.05.016
Cloern, J. E., Foster, S. Q., and Kleckner, A. E. (2014). Phytoplankton Primary Production in the World’s Estuarine-Coastal Ecosystems. Biogeosciences 11, 2477–2501. doi:10.5194/bg-11-2477-2014
Collins, K. A., Lawrence, T. J., Stander, E. K., Jontos, R. J., Kaushal, S. S., Newcomer, T. A., et al. (2010). Opportunities and Challenges for Managing Nitrogen in Urban Stormwater: a Review and Synthesis. Ecol. Eng. 36, 1507–1519. doi:10.1016/j.ecoleng.2006.05.016
Conley, D. J., Paerl, H. W., Howarth, R. W., Boesch, D. F., Seitzinger, S. P., and Havens, K. E. (2009). Controlling Eutrophication: Nitrogen and Phosphorus. Science 323, 1014–1015. doi:10.1126/science.1167755
Davis, T. R., Harasti, D., Smith, S. D. A., and Kelaher, B. P. (2016). Using Modelling to Predict Impacts of Sea Level Rise and Increased Turbidity on Seagrass Distributions in Estuarine Embayments. Estuarine, Coastal Shelf Sci. 181, 294–301. doi:10.1016/j.ecss.2016.09.005
Duan, H-F., Li, F., and Yan, H. (2016). Multi-objective Optimal Design of Detention Tanks in Urban Stormwater Drainage System: LID Implementation and Analysis. Water Resour. Manag. 30, 4635–4648. doi:10.1007/s11269-016-1444-1
Duarte, C. (2007). The Future of Seagrass Meadows. Environ. Conservation 29, 192–206. doi:10.1017/s0376892902000127
Erler, D. V., Eyre, B. D., and Davison, L. (2008). The Contribution of Anammox and Denitrification to Sediment N2 Production in a Surface Flow Constructed Wetland. Environ. Sci. Tech. 42, 9144–9150. doi:10.1021/es801175t
Fan, L., Brett, M. T., Jiang, W., and Li, B. (2017). Dissolved Organic Nitrogen Recalcitrance and Bioavailable Nitrogen Quantification for Effluents from Advanced Nitrogen Removal Wastewater Treatment Facilities. Environ. Pollut. 229, 225–263. doi:10.1016/j.envpol.2017.05.093
Fletcher, T. D., Duncan, H. P., Poelsma, P., and Lloyd, S. D. (2004). Stormwater Flow and Quality, and the Effectiveness of Non-proprietary Stormwater Treatment Measures - a Review and Gap Analysis. (Melbourne, Australia: CRC for Catchment Hydrology), CRC for Catchment Hydrology. Technical report.
Francey, M., Fletcher, T. D., Deletic, A., and Duncan, H. (2010). New Insights into the Quality of Urban Stormwater in South Eastern Australia. J. Environ. Eng. 136 (4). doi:10.1061/(asce)ee.1943-7870.0000038
Garcia-Chance, L. M., Van Brunt, S. C., Majsztrik, J. C., and White, S. A. (2019). Short- and Long-Term Dynamics of Nutrient Removal in Floating Treatment Wetlands. Water Res. 159, 153–163. doi:10.1016/j.watres.2019.05.012
Garzon-Garcia, A., Burton, J. M., Lewis, S., Bainbridge, Z., De Hayr, R., Moody, P., et al. (2021). The Bioavailability of Nitrogen Associated with Sediment in Riverine Plumes of the Great Barrier Reef. Mar. Pollut. Bull. 173 (A), 112910. doi:10.1016/j.marpolbul.2021.112910
Geng, Y., Ge, Y., Luo, B., Chen, Z., Min, Y., Schmi, B., et al. (2019). Plant Diversity Increases N Removal in Constructed Wetlands when Multiple rather Than Single N Processes Are Considered. Ecol. Appl. 29 (7), e01965. doi:10.1002/eap.1965
Gold, A. C., Thompson, S. P., and Piehler, M. F. (2019). Nitrogen Cycling Processes within Stormwater Control Measures: A Review and Call for Research. Water Res. 149, 578–587. doi:10.1016/j.watres.2018.10.036
Greenaway, M., and Woolley, A. (2003). Changes in Plant Biomass and Nutrient Removal over 3 Years in a Constructed Wetland in Cairns, Australia. Water Sci. Tech. 44 (11-12), 303–310. doi:10.2166/wst.2001.0844
Griffiths, L. N., and Mitsch, W. J. (2017). Removal of Nutrients from Urban Stormwater Runoff by Storm-Pulsed and Seasonally Pulsed Created Wetlands in the Subtropics. Ecol. Eng. 108 (B), 414–424. doi:10.1016/j.ecoleng.2017.06.053
Hallett, C. S., Valesini, F. J., Kilminster, K., Wells, N. S., and Eyre, B. D. (2019). A Rapid Protocol for Assessing Sediment Condition in Eutrophic Estuaries. Environ. Sci. Process. Impacts 6, 1021–1037. doi:10.1039/c9em00141g
Hallin, S., Hellman, M., Choudhury, M. I., and Ecke, F. (2015). Relative Importance of Plant Uptake and Plant Associated Denitrification for Removal of Nitrogen from Mine Drainage in Sub-arctic Wetlands. Water Res. 85, 377–383. doi:10.1016/j.watres.2015.08.060
Hathaway, J. M., and Hunt, W. F. (2011). Evaluation of First Flush for Indicator Bacteria and Total Suspended Solids in Urban Stormwater Runoff. Water Air Soil Pollut. 217, 135–147. doi:10.1007/s11270-010-0574-y
Hatt, B. E., Deletic, A., and Fletcher, T. D. (2006). Integrated Treatment and Recycling of Stormwater: a Review of Australian Practice. J. Environ. Manage. 79 (1), 102–113. doi:10.1016/j.jenvman.2005.06.003
Hijosa-Valsero, M., Sidrach-Cardona, R., and Becares, E. (2012). Comparison of Interannual Removal Variation of Various Types of Constructed Wetland Types. Sci. Total Environ. 430, 174–183. doi:10.1016/j.scitotenv.2012.04.072
Howarth, R. W., and Marino, R. (2006). Nitrogen as the Limiting Nutrient for Eutrophication in Coastal marine Ecosystems: Evolving Views over Three Decades. Limnology and Oceanography 51 (1), 364–376. doi:10.4319/lo.2006.51.1_part_2.0364
Hua, Y., Peng, L., Zhang, S. H., Heal, K. V., Zhao, J. W., and Zhu, D. W. (2017). Effects of Plants and Temperature on Nitrogen Removal and Microbiology in Pilot-Scale Horizontal Subsurface Flow Constructed Wetlands Treating Domestic Wastewater. Ecol. Eng. 108, 70–77. doi:10.1016/j.ecoleng.2017.08.007
Hunt, W., Greenway, M., Moore, T. C., Brown, R. A., Kennedy, S. G., Line, D. E., et al. (2011). Constructed Stormwater Wetland Installation and Maintenance: Are We Getting it Right? J. Irrigation Drainage Eng. 137 (8), 469–474. doi:10.1061/(asce)ir.1943-4774.0000326
Hunt, W., Jarrett, A., Smith, J., and Sharkey, L. (2006). Evaluating Bioretention Hydrology and Nutrient Removal at Three Field Sites in North Carolina. J. Irrigation Drainage Eng. 132 (6), 600–608. doi:10.1061/(asce)0733-9437(2006)132:6(600)
Jacobsen, T., Vollertsen, J., and Nielsen, A. H. (2010). Urban and Highway Stormwater Pollution: Concepts and Engineering. Boca Raton, FL: CRC Press, Taylor and Francis Inc.
Jani, J., Yang, Y-Y., Lusk, M. G., and Toor, G. S. (2020). Composition of Nitrogen in Urban Residential Stormwater Runoff: Concentrations, Loads, and Source Characterisation of Nitrate and Organic Nitrogen. PLoS One 15 (2), e0229715. doi:10.1371/journal.pone.0229715
Jefferson, A. J., Bhaskar, A. S., and Hopkins, K. G. (2017). Stormwater Management Network Effectiveness and Implications for Urban Watershed Function: A Critical Review. Hydrological Process. 31, 4056–4080. doi:10.1002/hyp.11347
Jing, S-R., Lin, Y-F., Lee, D-Y., and Wang, T-W. (2001). Nutrient Removal from Polluted River Water by Using Constructed Wetlands. Bioresour. Tech. 76 (2), 131–135. doi:10.1016/s0960-8524(00)00100-0
Lake Macquarie City Council, (2013). Stormwater Quality Improvement Device Guidelines. Speers Point, NSW, Australia: Lake Macquarie City Council.
Lee, C-G., Fletcher, T. D., and Sun, G. (2009). Nitrogen Removal in Constructed Wetland Systems. Eng. Life Sci. 9 (1), 11–22. doi:10.1002/elsc.200800049
Lee, S. Y., Dunn, R. J. K., Young, R. A., Connolly, R. M., Dale, P. E. R., Dehayr, R., et al. (2006). Impact of Urbanisation on Coastal Wetland Structure and Function. Austral Ecol. 31, 149–163. doi:10.1111/j.1442-9993.2006.01581.x
Lenhart, H. A., Hunt, W. F., and Burchell, M. R. (2012). Harvestable Nitrogen Accumulation for Five Stormwater Wetland Plant Species: Trigger for Stormwater Control Measure Maintenance? J. Environ. Eng. 138 (9), 972–978. doi:10.1061/(asce)ee.1943-7870.0000550
Li, K., Liu, C., Ma, Y., and Wang, X. (2019). Land-based Dissolved Organic Nitrogen Dynamics and Bioavailability in Jiaozhou Bay, China. Estuarine, Coastal Shelf Sci. 220, 13–24. doi:10.1016/j.ecss.2019.02.045
Li, L., and Davis, Al. P. (2014). Urban Stormwater Runoff Nitrogen Composition and Fate in Bioretention Systems. Environ. Sci. Tech. 48, 3403–3410. doi:10.1021/es4055302
Lin, Y. F., Jing, S. R., Wang, T. W., and Lee, D. Y. (2002). Effects of Macrophytes and External Carbon Sources on Nitrate Removal from Groundwater in Constructed Wetlands. Environ. Pollut. 119 (3), 413–420. doi:10.1016/s0269-7491(01)00299-8
Lohrer, A. M., Thrush, S. F., Hewitt, J. E., Berkenbusch, K., Abrens, M., and Cummings, V. J. (2004). Terrestrially Derived Sediment: Response of marine Microbenthic Communities to Thin Terrigenous Deposits. Mar. Ecol. Prog. Ser. 273, 121–138. doi:10.3354/meps273121
Lopez-Ponnada, E. V., Lynn, T. J., Ergas, S. J., and Mihelcic, J. R. (2020). Long-term Field Performance of a Conventional and Modified Bioretention System for Removing Dissolved Nitrogen Species in Stormwater Runoff. Water Res. 170, 115336. doi:10.1016/j.watres.2019.115336
Luo, W., Bai, H., Jing, Q., Liu, T., and Xu, H. (2018). Urbanisation-induced Ecological Degradation in Midwestern China: an Analysis Based on an Improved Ecological Footprint Model. Resource Conservation and Recycling 137, 113–125. doi:10.1016/j.resconrec.2018.05.015
Lusk, M. G., and Toor, G. (2014). Organic Nitrogen Concentrations and Trends in Urban Stormwater: Implications for Stormwater Monitoring and Management. American Geophysical Union. Fall Meeting 2014.
Lusk, M. G., Toor, G. S., and Inglett, P. W. (2020). Organic Nitrogen in Residential Stormwater Runoff: Implications for Stormwater Runoff in Urban Watersheds. Sci. Total Environ. 707, 135962. doi:10.1016/j.scitotenv.2019.135962
Maucieri, C., Salvato, M., and Borin, M. (2020). Vegetation Contribution on Phosphorus Removal in Constructed Wetlands. Ecol. Eng. 152, 105853. doi:10.1016/j.ecoleng.2020.105853
Miller, W., and Boulton, A. J. (2005). Managing and Rehabilitating Ecosystem Processes in Regional Urban Streams in Australia. Hydrobiologia 552, 121–133. doi:10.1007/s10750-005-1510-9
Mitsch, W. J., and Gosselink, J. G. (2015). Wetlands. 5th Edition. Hoboken, New Jersey, USA: John Wiley & Sons.
Mitsch, W. J., Zhang, L., Stefanik, K. C., Nahlik, A. M., Anderson, C. J., Benal, B., et al. (2012). Creating Wetlands: Primary Succession, Water Quality Changes and Self-Design over 15 Years. BioScience 62, 237–250. doi:10.1525/bio.2012.62.3.5
Mitsch, W. J., Zhang, L., Waletzko, E., and Bernal, B. (2014). Validation of the Ecosystems Services of Created Wetlands: Two Decades of Plant Succession, Nutrient Retention and Carbon Sequestration in Experimental Riverine Marshes. Ecol. Eng. 72, 11–24.
National Research Council (2008). Urban Stormwater Management in the United States. Committee on Reducing Stormwater Discharge Contributions to Water Pollution. in Water Science and Technology Board, Division on Earth and Life Studies. Washington D.C., USA. doi:10.1016/j.ecoleng.2014.09.108
Natural Resource Management Ministerial Council (2006). Australia’s National Program of Action for Protection of the Marine Environment from Land-Based Activities. Canberra, ACT: Australian Government, Department of the Environment and Heritage.
Neff, J. C., Chapin, F. S., and Vitousek, P. M. (2003). Breaks in the Cycle: Dissolved Organic Nitrogen in Terrestrial Ecosystems. Front. Ecol. Environ. 1 (4), 205–211. doi:10.1890/1540-9295(2003)001[0205:bitcdo]2.0.co;2
Newcastle City Council (2012). Newcastle Development Control Plan. Newcastle, NSW, Australia: Newcastle City Council.
Nguyen, T. A. H., Ngo, H. H., Guo, W. S., Nguyen, T. H. H., Soda, S., Vu, N. D., et al. (2020). Whie Hard Clam Shells media to Improve Phosphorus Removal in Lab Scale Horizontal Sub-surface Flow Constructed Wetlands: Performance, Removal Pathways and Lifespan. Bioresour. Tech. 312, 123602. doi:10.1016/j.biortech.2020.123602
Nixon, S. W. (1981). in Remineralisation and Nutrient Cycling in Coastal Marine Ecosystems (Totowa, New Jersey, USA: Humana Press).Estuaries and Nutrients
Noe, G. B., Harvey, J. W., and Saiers, J. E. (2007). Characterization of Suspended Particles in Everglades Wetlands. Limnology and Oceanography 52, 1166–1178. doi:10.4319/lo.2007.52.3.1166
Parker, N. (2010). Assessing the Effectiveness of WSUD in Southeast Queensland. Master of Engineering Thesis. Brisbane, Australia: Faculty of Built Environment and EngineeringUniversity of Queensland.
Payne, E. G. I., Fletcher, T. D., Russell, D. G., Grace, M. R., Cavagnaro, T. R., Evrard, V., et al. (2014). Temporary Storage or Permanent Removal? the Division of Nitrogen between Biotic Assimilation and Denitrification in Stormwater Biofiltration Systems. PLoS One 9 (3), e90890. doi:10.1371/journal.pone.0090890
Perner, H., Schwarz, D., and George, E. (2006). Effect of Mycorrhizal Inoculation and Compost Supply on Growth and Nutrient Uptake of Young Leek Plants Grown on Peat-Based Substrates. HortScience 41 (3), 628–632. doi:10.21273/hortsci.41.3.628
Rivers, E. N., McMillan, S. K., Bell, C. D., and Clinton, S. M. (2018). Effects of Urban Stormwater Control Measures on Denitrification in Receiving Streams. Water 10 (11), 1582. doi:10.3390/w10111582
Rogers, K. H., Breen, P. F., and Chick, A. J. (1991). Nitrogen Removal in Experimental Wetland Treatment Systems: Evidence for the Role of Aquatic Plants. J. Water Pollut. Control. Fed. 63, 934–941. doi:10.2175/wer.64.7.11
Ruiz-Rueda, O., Hallin, S., and Baneras, L. (2009). Structure and Function of Denitrifying and Nitrifying Bacterial Communities in Relation to Plant Species in a Constructed Wetland. FEMS. Microbiol. Ecol. 2, 308–319. doi:10.1111/j.1574-6941.2008.00615.x
Saunders, D. L., and Kalff, J. (2001). Nitrogen Retention in Wetlands, Lakes and Rivers. Hydrobiologia 443, 205–212. doi:10.1023/a:1017506914063
Shan, B., Ao, L., Hu, C., and Song, J. (2011). Effectiveness of Vegetation on Phosphorus Removal from Reclaimed Water by a Subsurface Flow Wetland in a Coastal Area. J. Environ. Sci. 23 (10), 1594–1599. doi:10.1016/s1001-0742(10)60628-6
Stormwater Australia (2018). Stormwater Quality Improvement Device Evaluation Protocol. Version 1.2.
Strecker, E. W., Quigley, M. M., Urbonas, B. R., Jones, J. E., and Clary, J. K. (2001). Determining Urban Storm Water BMP Effectiveness. J. Water Resource Plann. Manag. 127 (3), 144–149. doi:10.1061/(asce)0733-9496(2001)127:3(144)
Tan, E., Hsu, T. C., Huang, X., Lin, H. J., and Kao, S. J. (2017). Nitrogen Transformations and Removal Efficiency Enhancement of a Constructed Wetland in Subtropical Taiwan. Sci. Total Environ. 601, 1378–1388. doi:10.1016/j.scitotenv.2017.05.282
Tao, W., Hall, K. J., and Duff, S. J. B. (2006). Performance evaluation and effects of hydraulic retention time and mass loading rate on treatment of woodwaste leachate in surface-flow constructed wetlands. Ecol. Eng. 26 (3), 252–265.
Taylor, G. D., Fletcher, T. D., Wong, T. H. F., Breen, P. F., and Duncan, H. P. (2005). Nitrogen Composition in Urban Runoff – Implications for Stormwater Management. Water Res. 39 (10), 1982–1989. doi:10.1016/j.watres.2005.03.022
Verhofstad, M. J. J. M., Poelen, M. D. M., van Kempen, M. M. L., Bakker, E. S., and Smolders, A. J. P. (2017). Finding the Harvesting Frequency to Maximise Nutrient Removal in a Constructed Wetland Dominated by Submerged Aquatic Plants. Ecol. Eng. 106, 423–430. doi:10.1016/j.ecoleng.2017.06.012
Vymazal, J. (2007). Removal of Nutrients in Various Types of Constructed Wetlands. Sci. Total Environ. 380, 48–65. doi:10.1016/j.scitotenv.2006.09.014
Vymazal, J., Zhao, Y., and Mander, U. (2021). Recent Research Challenges for Constructed Wetlands for Wastewater Treatment; A Review. Ecol. Eng. 169, 106318. doi:10.1016/j.ecoleng.2021.106318
Walsh, C. J., Fletcher, T. D., and Burns, M. J. (2012). Urban Stormwater Runoff: A New Class of Environmental Flow Problem. PLoS One 7 (9), e45814. doi:10.1371/journal.pone.0045814
Walsh, C. J., Fletcher, T. D., and Ladson, A. R. (2005a). Stream Restoration in Urban Catchments through Redesigning Stormwater Systems: Looking to the Catchment to Save the Stream. J. North Am. Benthological Soc. 24 (3), 690–705. doi:10.1899/04-020.1
Walsh, C. J., Roy, A. H., Feminella, J. W., Cottingham, P. D., Groffman, P. M., and Morgan, R. P. (2005). The Urban Stream Syndrome: Current Knowledge and the Search for a Cure. J. North Am. Benthological Soc. 24, 706–723. doi:10.1899/04-028.1
Wang, C. Y., Sample, D. J., Day, S. D., and Grizzard, T. J. (2015). Floating Treatment Wetland Nutrient Removal through Vegetation Harvest and Observations from a Field Study. Ecol. Eng. 78, 15–26. doi:10.1016/j.ecoleng.2014.05.018
Water By Design (2010). MUSIC Modelling Guidelines: SEQ Healthy Waterways Partnership. Brisbane, Queensland.
Wenger, S. J., Roy, S. H., Jackson, R., Bernhardt, E. S., Carter, T. J., Filoso, S., et al. (2009). Twenty-six Key Research Questions in Urban Stream Ecology: an Assessment of the State of the Science. J. North Am. Benthological Soc. 28 (4), 1080–1098. doi:10.1899/08-186.1
Williams, C. J., Frost, P. C., and Xenopoulos, M. A. (2013). Beyond Best Management Practices: Pelagic Biogeochemical Dynamics in Urban Stormwater Ponds. Ecol. Appl. 23 (6), 1384–1695. doi:10.1890/12-0825.1
Wu, S., Kuschk, P., Brix, H., Vymazal, J., and Dong, R. (2014). Development of Constructed Wetlands in Performance Intensifications for Wastewater Treatment: A Nitrogen and Organic Matter Targeted Review. Water Res. 57, 40–55. doi:10.1016/j.watres.2014.03.020
Yang, Y-Y., and Lusk, M. G. (2018). Nutrients in Urban Stormwater Runoff: Current State of the Science and Potential Mitigation Options. Curr. Pollut. Rep. 4, 112–127. doi:10.1007/s40726-018-0087-7
Yang, Y-Y., and Toor, G. S. (2016). 砹15N and 砹18O reveal the sources of nitrate-nitrogen in urban residential stormwater runoff. Environ. Sci. Technol. 42(6), 2881–2889.
Yu, Y., Song, J., Xuegang, L., Yuan, H., and Li, N. (2012). Distribution, Sources and Budgets of Particulate Phosphorus and Nitrogen in the East China Sea. Continental Shelf Res. 43, 142–155. doi:10.1016/j.csr.2012.05.018
Keywords: constructed wetland, WSUD, nitrogen, phosphorus, nutrient removal, total suspended sediment, maintenance
Citation: Wright AS, Doblin MA and Scanes PR (2022) Improper Maintenance Activities Alter Benefits of Urban Stormwater Treatment in a Temperate Constructed Wetland in NSW, Australia. Front. Environ. Chem. 3:834191. doi: 10.3389/fenvc.2022.834191
Received: 13 December 2021; Accepted: 17 March 2022;
Published: 12 April 2022.
Edited by:
Prasanna Egodawatta, Queensland University of Technology, AustraliaReviewed by:
Amin Mojiri, Hiroshima University, JapanMatthew Reid, Cornell University, United States
Copyright © 2022 Wright, Doblin and Scanes. This is an open-access article distributed under the terms of the Creative Commons Attribution License (CC BY). The use, distribution or reproduction in other forums is permitted, provided the original author(s) and the copyright owner(s) are credited and that the original publication in this journal is cited, in accordance with accepted academic practice. No use, distribution or reproduction is permitted which does not comply with these terms.
*Correspondence: Aaron S. Wright, YWFyb24ud3JpZ2h0QHN0dWRlbnQudXRzLmVkdS5hdQ==