- 1School of Earth Science and Engineering, Hohai University, Nanjing, China
- 2Faculty of Natural Sciences, Hung Vuong University, Ho Chi Minh, Vietnam
- 3Biosystems and Environmental Engineering Research Group, Department of Soil Science and Agricultural Engineering, University of Zimbabwe, Harare, Zimbabwe
- 4Department of Water and Environmental Science and Engineering, Nelson Mandela African Institution of Science and Technology, Arusha, Tanzania
- 5Faculty of Science and Technology, Campus of Banekane, Université des Montagnes, Bangangté, Cameroon
- 6Angewandte Geologie, Universität Göttingen, Göttingen, Germany
- 7Centre for Modern Indian Studies (CeMIS), University of Göttingen, Göttingen, Germany
The suitability of remediation systems using metallic iron (Fe0) has been extensively discussed during the past 3 decades. It has been established that aqueous Fe0 oxidative dissolution is not caused by the presence of any contaminant. Instead, the reductive transformation of contaminants is a consequence of Fe0 oxidation. Yet researchers are still maintaining that electrons from the metal body are involved in the process of contaminant reduction. According to the electron efficiency concept, electrons from Fe0 should be redistributed to: i) contaminants of concern (COCs), ii) natural reducing agents (e.g., H2O, O2), and/or iii) reducible co-contaminants (e.g. NO3-). The electron efficiency is defined as the fraction of electrons from Fe0 oxidation which is utilized for the reductive transformations of COCs. This concept is in frontal contradiction with the view that Fe0 is not directly involved in the process of contaminant reduction. This communication recalls the universality of the concept that reductive processes observed in remediation Fe0/H2O systems are mediated by primary (e.g., FeII, H/H2) and secondary (e.g., Fe3O4, green rusts) products of aqueous iron corrosion. The critical evaluation of the electron efficiency concept suggests that it should be abandoned. Instead, research efforts should be directed towards tackling the real challenges for the design of sustainable Fe0-based water treatment systems based on fundamental mechanisms of iron corrosion.
Introduction
Metallic iron (Fe0), also termed as zero-valent iron (ZVI) is widely considered as a cost-effective reducing agent for organic pollutants in groundwater (Henderson and Demond 2007; Guan et al., 2015; Cao et al., 2020; He et al., 2020). The Fe0-based permeable reactive barrier (PRB) technology for groundwater remediation is rooted on this premise (Gillham 2008; Chen et al., 2019; Xiao et al., 2020a; Xiao et al., 2020b; He et al., 2020; Wang et al., 2022). Fe0 has also been successfully used for the removal of various inorganic contaminants (e.g. As, NO3−) and pathogens (e.g., bacteria, viruses) from polluted waters (Richardson and Nicklow 2002; Henderson and Demond 2007; Gheju 2011; Guan et al., 2015; Cao et al., 2020; Kim et al., 2021; Noubactep, 2021). However, these applications are mainly perceived to be derived from the Fe0 PRB technology for organic pollutants (Obiri-Nyarko et al., 2014; Naseri et al., 2017). The concept that Fe0 is an electron donor under environmental conditions has never been experimentally established (Warren et al., 1995; Farrell et al., 2001; Lavine et al., 2001; Jiao et al., 2009; Naseri et al., 2017; Cao et al., 2020; Hu et al., 2020; Noubactep, 2022). For example, while investigating the reductive dechlorination of carbon tetrachloride (CT) in Fe0/H2O systems, Jiao et al. (2009) clearly demonstrated that reducing electrons are not from Fe0, although iron corrosion was helpful for CT reductive dechlorination. Their conclusions read as: “The inherent relationship between the dechlorination of CT and the corrosion of iron is attributed to the fact that the adsorbed hydrogen atoms produced during the iron corrosion process are necessary for the dechlorination process of CT.” Table 1 summarizes some key arguments presented in the broad scientific literature prior to the advent of the recent Fe0 remediation technology, and disproving the reductive transformation concept.
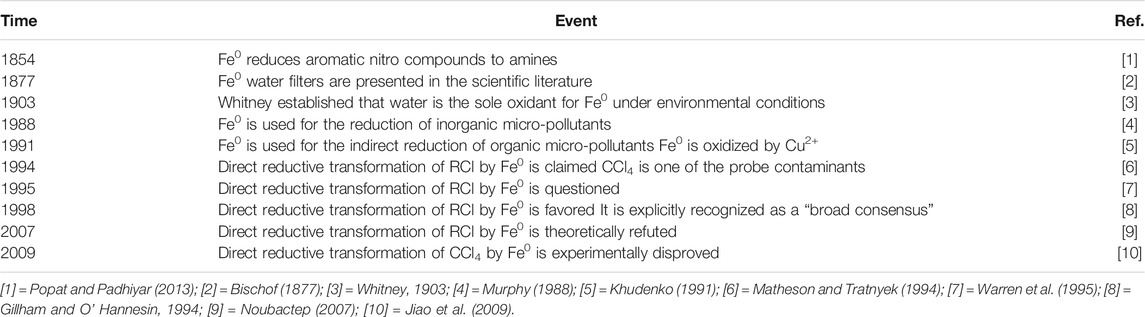
TABLE 1. An overview of the most important discoveries regarding the reduction mechanisms in Fe0/H2O system shown in the form of a timeline. For the discussion herein, it suffices to consider two facts: i) ref. [6] has not considered previous works, and ii) the results of [6] was disproved by ref. [10].
The idea that organic pollutants are reductively transformed by Fe0 was introduced in the scientific literature by Reynolds et al. (1990). Scientists from the University of Waterloo (Canada) were investigating the potential for sampling bias caused by sorption of chlorinated organic species to materials commonly used in groundwater sampling (Lee et al., 2004; Gillham 2008; Cao et al., 2020). Their results revealed losses of chlorinated organic contaminants from water samples in contact with Fe0-based vessels. Hence, reductive dechlorination was proposed as the most likely reaction path (Reynolds et al., 1990; Gillham 2008). This observation coincided with a period when geochemists were looking for suitable materials for the realization of the concept of groundwater remediation using PRBs as introduced in the 1980s by McMurty and Elton (1985). In other words, Fe0 was considered a reducing agent (or an electron donor) for organic pollutants, because their reductive transformation was observed in its presence, in the Fe0/H2O system. This coincidence was misinterpreted as a scientific fact and still prevails (Cao et al., 2020; Thakur et al., 2020; Noubactep, 2022). Investigations by Matheson and Tratnyek (1994) and Weber (1996) have been reported to confirm these observations. Moreover, it was claimed that the observation that organic pollutants can be reduced in Fe0/H2O systems was novel (Matheson and Tratnyek, 1994; Gillham 2008). Unfortunately, the then available seminal works of Khudenko (1985), Khudenko (1987), and Khudenko (1991) frontally contradict the claimed novelty (Cao et al., 2020). In particular, in the paper entitled, “Feasibility evaluation of a novel method for destruction of organics” (Khudenko, 1991), Boris Michael Khudenko demonstrated that Cu2+ cementation by Fe0 can be used to induce the reductive degradation of organic pollutants (Table 2). Clearly, Fe0 is oxidized by Cu2+ and reaction products (FeII and H/H2 species) act as reducing agents for the (organic) contaminants of concern (COCs). In other words, FeII species resulting from iron corrosion are used for the “destruction of organics.” Factually, H2 and H species also resulting from iron corrosion are reducing agents as demonstrated by Jiao et al. (2009).

TABLE 2. Summary of experimental conditions and results for the Fe0/H2O systems investigated by Khudenko (1991). pH0 = initial pH, [X] = concentration of X, pH = final pH, E = per cent removal efficiency, n.s: not specified.
Table 2 summarizes the experimental conditions and results of Khudenko (1991) with regard to the Fe0/H2O system. In Khudenko (1991)’s work, an aqueous solution of Direct Yellow 12 was acidified to an initial pH value of 4.5 using H2SO4. Two parallel experiments were performed differing in the addition or non-addition of 100 mg L-1 Cu2+ (CuSO4). Used Fe0 was a 1 m long iron wire of 0.2 mm in diameter. The filtrate was stirred by a magnetic stirrer. Results demonstrated that with Cu2+ addition, complete discoloration was achieved within 2.5 min, while the system without Cu2+ could not be discolored at all after 60 min of stirring. More Fe0 was consumed in the absence of Cu2+ than with Cu2+. The second experiment was conducted with a wastewater from finishing operations of a textile mill. The wastewater had a rosy color, and an initial pH value of 8.0. Addition of Cu2+ alone changed the color to dark blue suggesting formation of Cu2+ complex. Cementation with Fe0 and Cu2+ resulted in 98.5% color removal, while the process without copper resulted in just 10% color removal (Table 2).
It is surprising that 3 years after the brilliant concept of Khudenko (1991) and its validation using both synthetic and real wastewater, Matheson and Tratnyek (1994) introduced the contradiction without proving Khudenko (1991) wrong. In fact, Matheson and Tratnyek (1994) did not even consider that earlier work by Khudenko (1991). Bigg and Judd, 2000 were the first scientists to cite Khudenko (1991), 2 years after the report that the concept that, Fe0 is a reducing agent was a “broad consensus” (O’Hannesin and Gillham, 1998). It is also surprising that the view of Matheson and Tratnyek (1994) was claimed to be experimentally validated (Roberts et al., 1996; Weber, 1996) and is still favored by the majority of active researchers on the remediation Fe0/H2O system (Naseri et al., 2017; Xiao et al., 2020a; Xiao et al., 2020b; Cao et al., 2020; Hu et al., 2020; Hu et al., 2021; Noubactep, 2022). Accordingly, the whole mechanistic discussion is based on the idea that there is some electron transfer from the Fe0 bulk material to COCs, which are potentially transformed into non-toxic or less toxic species (He et al., 2020). The extent of this reaction was conventionally evaluated using the reaction rate constant k (kobs or kSA) (Johnson et al., 1996; McGeough et al., 2007). However, according to Liu et al. (2013) this is inappropriate because the extent of iron corrosion and the proportion of electrons used for the transformation of COCs should be considered to assess the economics of the system. In other words, the goal was to avoid superfluous Fe0 dosages which impede the economics of the designed systems (Wu et al., 2014; Shufen et al., 2018, He et al., 2020). During the past 8 years, an important number of papers has been published on the suitability of the electron efficiency concept (EE concept). He et al. (2020) give an excellent overview on the topic, and, interested readers are referred to this very recent review article.
To this point, the presentation has highlighted that the EE concept is intrinsically wrong, because Fe0 does not play any significant role in the process of contaminant reductive transformation in Fe0/H2O systems (Whitney, 1903; Jiao et al., 2009; Noubactep, 2022). In fact, while Jiao et al. (2009) have proven Matheson and Tratnyek (1994) wrong, Whitney (1903) had already established that under environmental conditions, Fe0 is oxidized only by protons (H+), even in the presence of dissolved oxygen (O2) and carbonic acid (H2CO3) (Table 1). The present communication aims at demonstrating the fallacy of the EE concept in order to avoid its further propagation. The presentation starts with chemistry of the Fe0/H2O system, followed by a historical overview on water treatment using Fe0, and ends with a critical evaluation of the usefulness of the EE concept.
The Chemistry of the Fe0/H2O System
Aqueous iron corrosion is an electrochemical process which needs four compartments to occur: an anode, a cathode, a conductor and an electrolyte (Landolt, 2007; Groysman, 2010). In the remediation Fe0/H2O system, the conductor is the metal body (Fe0), the electrolyte is the polluted water, the anode is an area of the Fe0 surface where oxidative dissolution occurs (releasing ferrous iron–Fe2+), and the cathode is an area of the Fe0 surface where electrons left behind by Fe2+ are transferred to a reducible species. A key feature of this process is that, the reactions at the anode and the cathode occur simultaneously, and the prerequisite is that the electrolyte must be in contact with both the anode and the cathode (Landolt, 2007; Noubactep, 2014; Noubactep, 2016).
Aqueous iron corrosion proceeds as follows: i) Fe0 is oxidatively dissolved at the anode to release Fe2+, ii) the generated Fe2+ ions migrate in the polluted water (electrolyte), and iii) then electrons left behind by Fe2+ are transferred through the metal body (conductor) to a reducible species at the cathode. It is crucial to underline that electrons are transported from the anode to the cathode by Fe0. Electron transfer to any adsorbed species only occurs if there is no conduction barrier at the Fe0 surface (Landolt, 2007; Nesic, 2007; Lazzari, 2008; Groysman, 2010). It is well-known that, at pH > 4.5, an oxide scale forms on the Fe0 surface and shields it from dissolved species, including dissolved O2 (Stratmann and Mü ller, 1994; Lazzari, 2008). For the EE concept to be applicable, it means that the universal oxide scale on Fe0 should be electronically conductive, which is not the case in Fe0/H2O systems (Noubactep 2007; Noubactep, 2008; Noubactep, 2014; Noubactep, 2016). Figure 1 summarized the pathways of contaminant removal and transformations in Fe0/H2O systems.
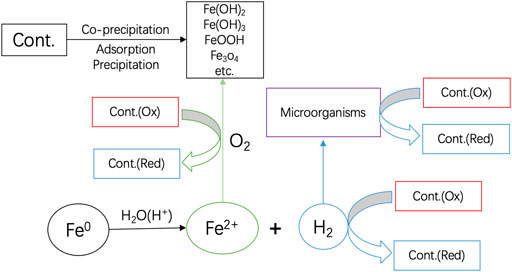
FIGURE 1. Pathways of contaminant removal and transformations in Fe0/H2O systems: Only water has access to the Fe0 surface. Fe2+ and H2 are stand alone reducing agents. O2 is reduced by Fe2+. H2 can be used by microorganisms to induce redox transformations of dissolved species. Upon oxidation of Fe2+, various solid iron hydroxides/oxides precipitate and act as contaminant scavengers. Reproduced with permission from Elsevier, the original was published in Chemosphere 287 (2022) 132314 (Noubactep, 2022).
The electrochemical reaction for aqueous iron corrosion is depicted in Eq. 1:
Eq. 1 shows that iron corrosion consumes protons, thereby increasing the pH value. This means that adding protons (acidification) is a powerful tool to intensify iron corrosion where it is needed, for example for H2 evolution (Ndé -Tchoup é et al., 2020). However, for environmental remediation, a pH shift to lower values is not typically envisaged such that a pH increase occurs as a rule (Lipczynska-Kochany et al., 1994; Matheson and Tratnyek, 1994; Schreier and Reinhard, 1994; Hu et al., 2021). This pH increase favours the formation of ferrous hydroxides [Fe(OH)2], which polymerize and precipitate at the surface of Fe0 or in its vicinity (Eq. 2). When dissolved oxygen (O2) is present, ferric hydroxides [Fe(OH)3] are formed as well. In the real world, whether the conditions are anoxic or oxic, iron corrosion generates an oxide scale which permanently shields its surface and is made up of several oxides and hydroxides (Odziemkowski and Simpraga, 2004). The process of oxide scale formation and transformation is a dynamic one (Odziemkowski et al., 1998; Sikora and Macdonald, 2000; Nesic, 2007; Lazzari, 2008; Groysman, 2010). It is certain that no electronically conductive oxide scale can be formed at the Fe0 surface under environmental conditions (Nesic, 2007; Lazzari, 2008). In other words, the oxide scale, acting as diffusion barrier for contaminants and dissolved O2, also represents a conductive barrier for electrons from Fe0. For this reason, electrons from the metal body cannot (quantitatively) reduce any initially dissolved species (contaminants and O2) (Figure 1) (Stratmann and Mü ller, 1994; Noubactep 2007; Noubactep, 2008; Jiao et al., 2009; Noubactep, 2013; Noubactep, 2015; Noubactep, 2019; Hu et al., 2020). Clearly, the EE concept is built on a thinking mistake. Accordingly, scientists propagating this concept are justifying their own mistakes by citing past mistakes. The EE concept would have been valid to some extent, if the oxide scale was not present. That is under acidic conditions (pH < 4.5) which is not the pH range of environmental remediation (Gillham 2008; Ghauch, 2015). The barrier nature of the oxide film implies that all reductive transformations are mediated by corrosion products (e.g., FeII, H/H2, Fe3O4, green rust). However, contaminant removal is mediated by adsorption onto and co-precipitation with solid iron corrosion products (FeCPs). In fixed beds, size-exclusion is the other relevant removal mechanism (Noubactep, 2007, Noubactep, 2008).
The Fe0 research community is reminded of a famous quote by Mahatma Gandhi “An error does not become truth by reason of multiplied propagation, nor does truth become error because nobody sees it.” Contextualizing this to the Fe0 literature, the highlighted mistake (error) has been propagated since the time the work of Khudenko (1991) was ignored. Despite several efforts by our group pointing out this conceptual mistake (Noubactep 2007, Noubactep, 2008; Noubactep, 2010a; Noubactep, 2010b; Noubactep, 2014; Noubactep, 2016), the truth has been ignored for 1 decade already (Xiao et al., 2020a; Xiao et al., 2020b; Cao et al., 2020; Cao et al., 2021a; Cao et al., 2021b; Cao et al., 2021c; Cao et al., 2021d; Hu et al., 2021; Noubactep, 2022). However, an accurate fundamental understanding of processes governing contaminant removal is critical in the design and operation of Fe0-based systems. This is particularly important given that Fe0 based remediation systems have wide practical applications. These applications have been discussed in earlier papers (Naseri et al., 2017; Antia, 2020, Huang et al., 2021a), thus, a detailed review is beyond the scope of the present paper. In summary, typical applications of Fe0-based remediation systems documented in literature include: i) decentralized safe drinking water provision in low-income settings (Huang et al., 2021b; Mueller et al., 2021), ii) industrial wastewater treatment systems (Li et al., 2019; Kulkarni et al., 2020), iii) recovery of heavy metals from industrial effluents (Vollprecht et al., 2018; Calabrò et al., 2021; Noubactep, 2021), iv) urban stormwater treatment (Rahman et al., 2013; Tian et al., 2019), v) treatment of drainage water from agroecosystems (Das et al., 2017; Lanet et al., 2021), vi) subsurface permeable reactive barriers (PRBs) for remediation of contaminated groundwater (Thakur et al., 2020; Njaramba et al., 2021, Wang et al., 2022), and vii) treatment of domestic wastewater (Wakatsuki et al., 1993; Latrach et al., 2018).
The next section gives some selected examples on how the past decade has ignored available knowledge.
Historical Overview of the Fe0 Remediation Technology
Fe0 has been used in the following applications: i) H2 production, ii) food packaging, iii) laboratory demonstration (e.g., practicals), iv) drinking water conservation, v) mining activities (e.g., copper cementation), and vi) safe drinking water provision for many decades/centuries (Davis, 1891; Bafghi et al., 2008; Noubactep, 2010a; Noubactep, 2013; Mwakabona et al., 2017; Antia, 2020; Cao et al., 2020; Noubactep, 2020; Rangan et al., 2020; Huang et al., 2021a). This section presents the various uses of the Fe0/H2O system for water treatment in a historical perspective. Interested readers are referred to a recent overview summarizing 160 years of Fe0 technology based mainly on patent literature (Antia, 2020).
Fe0 for Drinking Water Provision
Fe0 has been used for safe drinking water provision for a very long time (Bischof, 1877; Davis, 1891; van Craenenbroeck, 1998; Mwakabona et al., 2017; Antia, 2020; Noubactep, 2020). Ancient textbooks reveal that this technology was commonplace in West England in the 1850s (Davis, 1891). Bischof (1873) gives an overview of the ancient efforts for the design of household water filters culminating in the design of spongy iron filters. In 1881, spongy iron filters were successfully pilot tested in Antwerp (Belgium), and could enable the supply of drinking water to 200,000 inhabitants for 18 months without any maintenance (Mwakabona et al., 2017; Antia, 2020; Cao et al., 2020).
Spongy iron filters were used to treat yellow-colored water contaminated with pathogens (Devonshire, 1890; Antia, 2020). The yellow coloration reveals that organic substances were present in water. Fe0 was said to be oxidized by dissolved O2, and contaminants were removed by adsorption and occlusion (co-precipitation). In other words, contaminants were removed by mechanisms similar to that in the flocculation process, with the only difference that flocs were not formed in a bulk solution but in the vicinity of individual Fe0 grains (Figure 1) (Bojic et al., 2009). The design of Fe0 filters were not based on the stoichiometry of any chemical reaction, but on the probability to generate enough “flocs” on time to address the extent of water contamination.
Household and community drinking water treatment systems working on the same principles were independently designed later (Antia, 2020). Available designs include; i) the Anderson Process (Devonshire, 1890; van Craenenbroeck, 1998), ii) the Emmons Process (Lauderdale and Emmons, 1951; Lacy, 1952), iii) the SONO arsenic filters (Hussam and Munir, 2007; Neumann et al., 2013) and iv) the IITB (Indian Institute of Technology Bombay, India) arsenic filters (Chaudhari et al., 2014; Banerji and Chaudhari, 2017). It is important to point out that, in all these designs, iron corrosion products (FeCPs) for contaminant scavenging are generated without any addition of chemicals. Designs using common oxidants (e.g. H2O2, O3) to intensify iron corrosion were also presented (e.g., Gottinger et al., 2013; Kowalski and Sø gaard, 2014; Tepong-Tsindé et al., 2015). Evidently, these findings show that the formation of flocs or FeCPs is critical for contaminant removal, and reaction stoichiometry is not critical in the design and operation of these filters.
Fe0 for Wastewater Treatment
Fe0 has been used for wastewater treatment for many decades (Obiri-Nyarko et al., 2014; Antia, 2020; Lanet et al., 2021). Applications encompass the recovery of important elements (Vollprecht et al., 2018; Vollprecht et al., 2020), and the treatment of wastewaters from domestic (Wakatsuki et al., 1993), industrial (Oldright et al., 1928; Gould, 1982; Vollprecht et al., 2020), and agricultural sources (Anderson, 1989; James et al., 1992; Erickson et al., 2007). In all these applications, FeCPs serve as contaminant scavengers and the systems are designed to produce enough scavengers. Again, no reaction stoichiometry is needed, and the major reason is that the long-term kinetics of iron corrosion in each individual case is not known (Lufingo et al., 2019; Ndé -Tchoup é et al., 2020; Lanet et al., 2021; Noubactep, 2022).
The presentation until now shows that Fe0 based remediation systems have been designed and mostly satisfactorily operated for the past 170 years. During the time before the advent of the PRB technology (1990), efforts to rationalize the efficiency of the systems using reaction stoichiometry were scarce. For example, Gould (1982) found that, in their systems, more CrVI was reduced than predicted by the cementation process (electrons from Fe0). The same author speculated that some CrVI was reduced by in-situ generated H2 and pointed out that FeII species also reduce CrVI (Gheju, 2018).
Fe0 for PRBs
Research on Fe0 PRBs started around 1990 (Gillham 2008). In 1994, the four first peer-reviewed articles were published by four research groups in three different journals (Gillham and O’ Hannesin, 1994; Lipczynska-Kochany et al., 1994; Matheson and Tratnyek, 1994; Schreier and Reinhard, 1994) (Supplementary Table S1). Supplementary Table S1 summarizes their current bibliometric evaluation according to SCOPUS Web of knowledge and Table 3 summarizes their experimental conditions. This section demonstrates that the four papers have already revealed all important issues for the discussion of the invalidity of the EE concept. In particular, Schreier and Reinhard (1994) tested both Fe0 and Mn0, while Lipczynska-Kochany et al. (1994) used a pyrite mineral (FeS2) to delay the precipitation of FeCPs in the vicinity of Fe0.

TABLE 3. Summary of some experimental conditions used for batch experiments in the four first peer-reviewed articles using metallic iron (Fe0) for contaminant removal. Chlorinated hydrocarbons were used with various initial concentrations. CT stands for carbon tetrachloride. “Citation” is the number of independent citations (excluding self-citations) according to Scopus (www.scopus.com: February 25, 2021).
The common feature of the four papers is that they were investigating the reductive degradation of chlorinatedhydrocarbons in the presence of Fe0. It was clear to all of the investigators that FeCPs will form, and influence the reductive process. Among other results, Schreier and Reinhard (1994) observed a lag time in the process of contaminant reduction in Fe0/H2O systems. In biological systems, for example, a lag time is the time necessary to induce the synthesis of appropriate enzymes (Schreier and Reinhard, 1994). Such lag periods were repeatedly reported in the literature over the years (e.g., Huang et al., 1998; Noubactep et al., 2003; Hao et al., 2005; Cao et al., 2021a; Cao et al., 2021b; Cao et al., 2021c; Cao et al., 2021d). In Fe0/H2O systems, the lag time is indicative of the time required for the generation of FeCPs following Fe0 immersion into a polluted water. Proponents of the reductive transformation concept, and the EE concept, often do not provide a rational reason for the lag time.
The currently well-accepted model for the lag time was summarized in a recent review article (Sun et al., 2016). It stipulates that, the lag time corresponds to the time to transform the outer Fe2O3 layer, an air-formed oxide scale into Fe3O4. In fact, commercial Fe0 specimens used in water treatment are covered by a pre-existing oxide scale consisting of an inner Fe3O4 layer and an outer Fe2O3 layer. The inner layer is electronically conductive by virtue of the semi-conductive nature of Fe3O4 (band gap: 0.11 eV) (Odziemkowski and Simpraga, 2004; Huang and Zhang, 2005). However, electron transport is hindered by the outer non-conductive Fe2O3 layer. According to this model, contaminant reductive transformation starts when the reduction of the Fe2O3 layer is completed.
The crucial role of in-situ generated FeCPs in adsorbing contaminants (Charlet et al., 1998; Furukawa et al., 2002) and their role in mediating contaminant reduction was demonstrated (White and Peterson, 1996). However, little attention was paid to the role of primary iron corrosion products (e.g. FeII, H/H2, Fe3O4, green rusts) as sole relevant source of electrons to mediate chemical reduction (Figure 1) (Jiao et al., 2009; Noubactep, 2010b; Noubactep, 2011; Cao et al., 2021a; Cao et al., 2021b). Another important observation of Schreier and Reinhard (1994) was that in experiments conducted at room temperature with Fe0, reductive transformations ceased abruptly after some 28 days. The Citation-values in Table 3 suggest that Lipczynska-Kochany et al. (1994) and Schreier and Reinhard (1994) have not continued working on the remediation Fe0/H2O system (Supplementary Table S1). The two other research groups have continuously worked on this field for at least 2 decades (Jeen et al., 2013; Fan et al., 2017). This is a plausible explanation for the fact that the research community could have widely accepted the mistake that contaminant removal is an electrochemical reaction represented by Eq. 3:
Table 3 summarized some relevant experimental conditions of the first four peer-reviewed articles on the remediation Fe0/H2O system. A total of 14 chlorinated hydrocarbons (RCl) were tested. Carbon tetrachloride (CT) was tested in 3 works. The tested RCl initial concentrations varied widely. It is seen that different experimental procedures were employed. While Fe0 powder (20 and 100 mesh) was used in individual works, there were huge differences in the used mass loading (17–250 g L−1), the nature and the volume of the reaction vessels, the mixing intensities (2–175 rpm), the availability of dissolved O2, and the experimental duration (1 h–75 days). It has been clearly demonstrated that these differences in the experimental designs are responsible for reported discrepancies (Devlin and Allin, 2005; Henderson and Demond 2007; Gheju 2011; Ghauch, 2015; Guan et al., 2015). Efforts towards more reliable experimental conditions were discussed and constantly actualized for example from 1999 to 2011 by The Interstate Technology and Regulatory Council (www.itrcweb.org) (ITRC, 2011). However, the key factor that the formation of oxide scales in the vicinity of Fe0 should be favored has received little attention (Devlin and Allin, 2005; Noubactep, 2008; Cao et al., 2021b). This premise implies that only quiescent or very slow-mixed batch experiments would produce results relevant for the design of filters (Noubactep et al., 2009). As concerning column experiments, low-flow-rate conditions should be observed (Colabro et al., 2021). These conditions enable the Fe2+ concentration to increase in the vicinity of Fe0 and eventually attain the saturation concentration. In batch experiments, beside quiescent conditions, limited testing volume favor a rapid formation of oxide scales on Fe0. The oxide scale ultimately exerts rate control through the control of reactant diffusion transport kinetics (Devlin and Allin, 2005; Noubactep, 2008; Cao et al., 2020; Noubactep 2020; Cao et al., 2021a).
The presentation until now shows that a great deal of work has been done under test conditions that do not permit formation of the universal oxide scale on Fe0. Accordingly, reproducible results could be obtained but they are by no means relevant for field conditions. These obvious problems of relevance of results has also favor the introduction of the EE concept which is vehemently refuted herein.
Falsifying the Reductive Transformation Concept
The present work intends to disprove the view that Fe0 is a reducing agent under environmental conditions. This section revisits Matheson and Tratnyek (1994) in the perspective of assessing whether or not their conclusions were supported by any methodical approach. The clear experimental observation is that there is reductive transformation of chlorinated methanes (RCl) in Fe0/H2O systems (Reynolds et al., 1990; Gillham and O’ Hannesin, 1994; Matheson and Tratnyek, 1994). The question is whether Matheson and Tratnyek (1994) have given a molecular level understanding of this process or established the reaction mechanism. This information is very important for the design of sustainable Fe0/H2O systems and was even the objective of their investigations (Matheson and Tratnyek, 1994). The history of science teaches that the most powerful advances in surface phenomena (including catalysis) are those that improve the ability to predict the efficiency of engineered systems (Buskirk and Baradaran, 2009). As recently recalled by Scott (2019), the fundamental understanding of the mechanisms of chemical reactions broadens the range of accurate predictions.
Any postulated reaction mechanism is a working hypothesis, whose predictions must be compared with experimental observations (Scott, 2019). Following this principle, Matheson and Tratnyek (1994) considered three possible pathways to justify the RCl reduction in Fe0/H2O systems: i) reductive dehalogenation by Fe0 (Eq. 3) which is equivalent to Fe0 oxidation by RCl (RCl = oxidizing agent, electrochemical mechanism) (Pathway 1), ii) reductive dehalogenation by Fe2+ (Eq. 4) which is equivalent to Fe2+ oxidation by RCl (chemical mechanism) (Pathway 2), and iii) reductive dehalogenation by H+ (Eq. 5) which is equivalent to H+ oxidation by RCl (chemical mechanism) (Pathway 3). These three mechanisms are all consistent with the named experimental observation. However, the reaction stoichiometry and the corrosion rate are not known, making the discussion with the spectral signatures of reactants, intermediates and products highly speculative. A profound analysis by Lee et al. (2004) revealed that “no carbon balances between reactants and products have ever been successfully done for many chlorinated hydrocarbons, which indicates that reduction pathways of metal-mediated reactions are not fully understood yet.” The statement by Lee et al. (2004) is just another hint for the lack of concrete evidence on the reductive transformation concept. The lack of mass-balance disproves the concept in the sense that reduction is not necessarily quantitative as the missing fraction of carbon is rather enmeshed in the matrix of iron corrosion products (co-precipitation) (Eusterhues et al., 2011; Noubactep, 2011). However, in mechanistic discussions, co-precipitation is mostly considered relevant for metallic species (Henderdon and Demond 2007; Colabro et al., 2021). The lack of mass-balance collectively questions the validity of the three postulated mechanisms.
The most important feature from Matheson and Tratnyek (1994) is the approach they used to rule out reduction after Pathway 2 and Pathway 3: Uncatalyzed reduction by dissolved H2 or Fe2+. They performed control experiments with H2-saturated water and a 100 mg L−1 FeCl2 over 15 days in the absence of Fe0 and could not observe any dehalogenation. They acknowledged that it was “difficult to exclude the possibility that adsorbed Fe2+ or nascent hydrogen” from Eq. 1 “may be participating in the dehalogenation reaction.” Additionally, the amendment of Fe0/H2O systems with external Fe2+ or H2 did not impact RCl reduction. Finally, the addition of 0.5 mM ethylenediaminetetraacetic acid (EDTA) had no effect on the RCl dehalogenation rate. EDTA was supposed to fix Fe2+, avoid hydoxide precipitation and keep the Fe0 surface free for RCl electrochemical reduction. Matheson and Tratnyek (1994) concluded on the basis of the presented experiments, that “reductive dehalogenation directly coupled with oxidative dissolution of the metal” (Pathway 1) was the “dominant process.” It is very important to note that Matheson and Tratnyek (1994) have just initiated a discussion, but just 2 years later, Weber (1996) claimed to have confirmed the electrochemical nature of contaminant reduction in Fe0/H2O systems. This section seeks to convince the reader that this concept was not established by any scientific approach.
In the absence of mass balance, Matheson and Tratnyek (1994) could not establish the mechanism of RCl reduction. However, there are two more intriguing facts: i) Fe0 oxidation by water is not discussed, and ii) water is just considered as a proton source for reaction after Eq. 3. The presentation of the authors textually reads, alkyl halides “can also be reduced by iron. In the presence of a proton donor like water, they typically undergo reductive dehalogenation.” This means that the seminal work of Whitney (1903) was ignored as well as thousands of works describing corrosion as resulting from the presence of water, including impurities in natural oil (Brondel et al., 1994) and atmospheric humidity (Stratmann and Müller, 1994). Moreover, the is no iron corrosion in dry (H2O free) chlorinated solvents (Rhodes and Carty, 1925; Archer, 1979). Fe0 corrosion by H2O (including moisture) and no Fe0 corrosion by dry RCl clearly indicates that more attention should have been paid to water as corroding agent in the Fe0 remediation literature (Ghauch, 2015; Cao et al., 2021b; Noubactep, 2022).
The extent to which it is possible to confirm a reaction mechanism is an issue that has preoccupied researchers in the chemical sciences for many decades (Brenner, 2010; Scott, 2019). In Geology, it is recommended to generate and test multiple working hypotheses in scientific inquiry to guard against drawing premature conclusions (Chamberlin, 1890). The science philosophers Karl Popper and Thomas Kuhn further asserted that scientific hypothesis must be falsifiable, or refutable (Scott, 2019). Following this approach, experiments should be designed to test the viability of multiple proposed reaction mechanisms. A mechanistic hypothesis can be falsified, resulting in its modification or even abandonment (Scott, 2019). Because the reaction mechanism of Matheson and Tratnyek (1994) was falsified by Jiao et al. (2009) it should be abandoned. Moreover, because their concept has not properly considered the redox reactivity of water (E0 = 0.00 V), the concept was false at the introduction.
This section calls authors to exercise vigilance to avoid making claims that a proof exists where it does not. To enable progress in the design of sustainable Fe0/H2O systems, attempts should be made to discredit rather than prove available mechanistic concepts (Scott, 2019). Louis Pasteur once formulated the following advice: “When you believe you have found an important scientific fact, and are feverishly curious to publish it, constrain yourself for days, weeks, years sometimes, fight yourself, try and ruin your own experiments, and only proclaim your discovery after having exhausted all contrary hypotheses” (Scott, 2019).
The Electron Efficiency Concept: Redox and Mass Balance Perspectives
Electron efficiency is defined as the fraction of total electrons from Fe0 that are used in the reduction of COCs (Eq. 3). The presentation until now has demonstrated that no single electron from Fe0 can be transferred to COCs because of the presence of the universal oxide scale which is never electronically conductive (Table 4). If electrons from Fe0 were transferred to any COC, there would have not been a lag time between the start of the experiment and the start of reductive transformation of COCs (Schreier and Reinhard, 1994; Huang et al., 1998; Hao et al., 2005; Cao et al., 2021d). Consequently, COCs, O2 and co-contaminants are reduced by FeII, H2, Fe3O4, green rust, and other reducing species generated in the Fe0/H2O system (Figure 2). For simplification, it can be assumed that reductive transformations are mainly mediated by Fe2+ and H2 from Eq. 1.
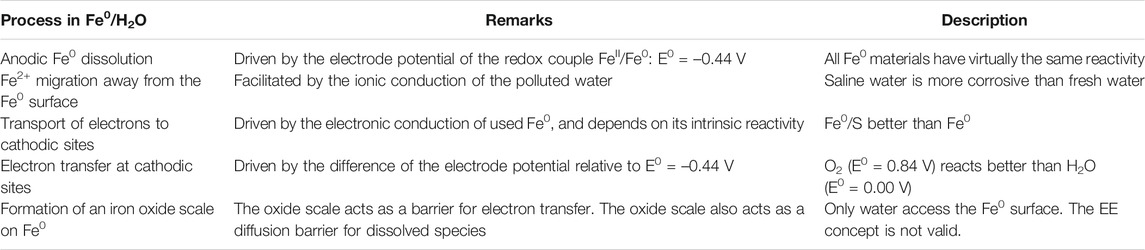
TABLE 4. Summary of the key factors affecting changes in Fe0/H2O systems and their implication for the validity of the electron efficiency concept (EE concept).
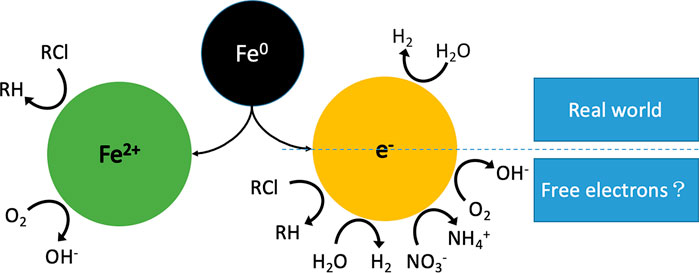
FIGURE 2. Pathways of contaminant transformations in Fe0/H2O systems. In the real world, only water has access to the metal surface. Fe2+ and H2 are stand alone reducing agents. O2 and RCl are reduced by Fe2+. The scenario of the EE concept corresponds to free electrons within the Fe0/H2O system, which is an absurdity.
Eq. 1 implies that the oxidation of 1 mole of Fe0 produces 1 mole of Fe2+ and 1 mole of H2. Fe2+ can donate one electron and H2 two electrons. This means that the electrochemical oxidation of 1 mole of Fe0 by water (H+ or H2O—the solvent), indirectly produces 3 moles of electrons for the reduction of O2, NO3− and all other oxidizing agents. In other words, the EE concept has better considered how the 3 moles of electrons are distributed. In this laborious effort, the mass balance of all involved species must be performed, starting with that of iron, which implies the need for experiments entailing the controlled dissolution of FeCPs. To the best of the authors’ knowledge, such a work has not been published. It is very strange, that mechanistic discussions have been performed without complete mass balance for 3 decades (Lavine et al., 2001; Lee et al., 2004; Noubactep, 2011; Cao et al., 2021c). Yet mass balance analysis is critical for proving the validity of the EE concept.
Another important feature about the EE concept is the way it addresses the electrode redox potential. The electrode potential of the FeII/Fe0 redox couple (E0 = –0.44 V) is not relevant to explain the reduction potential of the system towards individual contaminants. Instead, the potential of the couples FeIII/FeII and H+/H2, both in adsorbed and dissolved states are considered. For FeII, it has been demonstrated that, while dissolved FeII is far less powerful than Fe0, adsorbed FeII, also referred to as structural FeII is sometimes more powerful than Fe0: −0.65 ≤ E0 (V) ≤ −0.34 (White and Peterson, 1996). This last argument demonstrates that reduction of COCs is favourable, but this reduction of COCs is a chemical process as demonstrated for example by Khudenko (1991) and Jiao et al. (2009).
The last important feature about the EE concept is its usefulness. Reactivity loss and permeability loss are the two main challenges of the Fe0 remediation technology (Henderson and Demond 2007; Ghauch, 2015; Guan et al., 2015; Cao et al., 2020). Avoiding material wastage is certainly a noble goal, but it should start when the intrinsic reactivity of each relevant material is documented, and its long-term changes characterized (Ndé -Tchoup é et al., 2020). This presents a challenge, because the current situation is that Fe0 materials are not characterized, and their long term behaviour has not been really investigated (Li et al., 2019; Lufingo et al., 2019; Ndé -Tchoup é et al., 2020). For example, steel wool (d ≤ 90 μm), iron wire (d = 200 μm) and iron nails (d > 200 μm) are just tested as “Fe0 materials” in independent researches (Tepong-Tsindé et al., 2019).
In summary, evidence shows that: i) the EE concept fails to account for the lag time widely reported in Fe0/H2O systems, ii) the application of the EE concept is not based on any iron mass balance, iii) the EE concept disregards the decade old doubts on the reducing nature of Fe0, and iv) the EE concept does not contribute to solve the two major design issues for Fe0/H2O systems (i.e., reactive loss and permeability loss).
Revisiting Reactivity Loss and Permeability Loss
The two major problems of Fe0-based filters as documented from laboratory experiments, large-scale experiments and field implementations are reactivity loss and permeability loss (Guan et al., 2015; Cao et al., 2020). This corresponds to the state-of-the-art knowledge as summarized 14 years ago by Henderson and Demond (2007). Since then, the research community is divided in two schools. Some few researchers have realized that the dynamic nature of the Fe0/H2O system implies a different approach of investigation (Ghauch, 2015) while the large majority is still considering that isolated instrumental characterizations would help to understand the system. Clearly, the still praised monitoring of physical changes of Fe0 features using sophisticated tools including X-ray microcomputer tomography have not mediated the expected understanding of the technology as a whole. In other words, changes of Fe0 surface, Fe0 particle size, nature of expanding FeCPs, the reducing porosity can be experimentally documented. However, all these observations are collectively only static snap-shots and their measurements are inaccurate. Therefore, they cannot enable the generation of non-trivial models of the dynamic processes within Fe0/H2O systems (Santisukkasaem and Das, 2019), especially as these occur over an enormous range of time scales (from few seconds to some 2 decades) (Gillham 2008; Wilkin et al., 2014; Cao et al., 2020).
Reactivity Loss
The present paper posits that the consideration of wrong reactions and improper descriptors has been a major problem the Fe0 literature. This section clarifies this key issue. A parent compound (e.g., RX) reductively transformed in a Fe0/H2O system has been assumed to be transformed by electrons from the Fe0 body according to Eq. 3 (Section Fe0 for PRBs). A pseudo-first-order model is applied to describe the transformation of RX (Eq. 6). The natural logarithmic transformation of Eq. 6 yields a linear equation with the first-order rate constant kobs as slope (Eq. 7) (Reardon, 1995; Velimirovic et al., 2013). Usually, the mass normalized rate constants (kM, L g−1 h−1) and specific surface area normalized rate constants (kSA, L m−2 h−1) are used to account for the Fe0 mass and/or the Fe0 surface area in the systems
where C is the concentration at any time and C0 is the initial concentration of RX, kobs is the pseudo-first-order rate constant (h−1) and t (h) is the reaction time. In essence, rooting the reasoning on the available mass loading of Fe0 particles (g L−1) and/or their surface area concentration (m2 L−1) is a bright idea. However, the mistake made in considering Eq. 3 is not corrected.
Eq. 6 contents no single parameter related to water which clearly oxidizes Fe0 according to the definition of the EE concept (Liu et al., 2013). However, in trying to account for the “fraction of electrons” used to reduce water, the EE concept has replicated the same mistake. Therefore, the EE concept is not able to address the problem of reactivity loss. Reactivity loss has been documented for H2 generation (Eq. 1) in the absence of any contaminant (Reardon, 1995; Tang et al., 2017; Qin et al., 2018). In fact, reactivity loss is the “natural” time-dependent decrease of the kinetics of iron corrosion (Romanoff, 1957; Melchers and Petersen, 2018). As an intrinsic characteristic, reactivity loss can never be suppressed (Miyajima and Noubactep, 2015). In other words, reactivity loss has occurred in Fe0 reactive barriers satisfactorily operating for decades (O’Hannesin and Gillham, 1998; Guan et al., 2015; Wilkin et al., 2019). These systems are still efficient because their designs (e.g., Fe0 characteristics, Fe0 amount, Fe0:aggregate ratio, flow velocity) are somehow favorable. The rationale for their efficiency or the failure of other systems (e.g., Morrison et al., 2006; Ngai et al., 2007) is yet to be investigated.
Efforts to overcome “reactivity loss” are numerous, and include the following: i) increasing the available reactive surface area by using either porous materials (Hussam and Munir, 2007) or nano-sized Fe0 (Zhang, 2003), ii) creating galvanic cells by alloying Fe0 mostly in bimetallic materials (e.g. Fe0/Pd0, Fe0/Ni0), and iii) admixing Fe0 with reactive aggregates (e.g., Fe0/FeS2, Fe0/Fe3O4, Fe0/GAC, Fe0/MnO2) (Bojic et al., 2009; Btatkeu et al., 2014a; Btatkeu et al., 2014b; Dong et al., 2018; Han et al., 2019; Hu et al., 2021). GAC stands for granular activated carbon. Although GAC is chemically inert, it can create galvanic cells with Fe0 to alleviate its surface passivation (Gatcha-Bandjun et al., 2014; Dong et al., 2018). However, it is evident that none of these efforts has attempted to correct the original mistake. The documented enhanced efficiency is just due to increased iron corrosion or increased “electron density” (Noubactep and Caré, 2010; Noubactep et al., 2012). The proof for this statement is that admixing Fe0 with inert aggregates (e.g., Fe0/gravel, Fe0/sand) yields a more efficient system than the pure Fe0 system (Song et al., 2005; Btatkeu et al., 2014a; Btatkeu et al., 2014b). Accordingly, testing activated carbon, metal oxides and other reactive materials without a reference system (e.g., Fe0/sand) can only give qualitative results. It is thus not surprising that the reaction mechanisms and removal pathways using metal oxides assisted Fe0-based systems have not been investigated (Dong et al., 2018; Hu et al., 2021).
Permeability Loss
Permeability loss describes the time-dependent decrease of the hydraulic conductivity of Fe0-based filter (Caré et al., 2013, Domga et al., 2015, Cao et al., 2020, Antia, 2020, Njaramba et al., 2021). While considering Fe0 as the reducing agent and corrosion by water as a side reaction, permeability loss has been mainly attributed to the precipitation of foreign species evolving for instance CO32− or HCO3− (Henderson and Demond 2007; Henderson and Demond, 2011; Santisukkasaem and Das, 2019). However, the fact that contaminants are likely reduced by FeII species which are transformed to FeIII ones, suggest that (at least) twice more Fe0 is needed as suggested by the stoichiometry of Eq. 3: 1 mole of Fe0 exchanges 2 moles of electrons and 1 mole of Fe2+ only 1 mole of electrons. At pH > 4.5, each oxidized Fe0 atom is transformed to an iron hydroxide or oxide which volume is at least twice larger than that of the parent metal (Caré et al., 2008; Zhao et al., 2011; Caré et al., 2013; Domga et al., 2015; Yang et al., 2021; Noubactep, 2022). In other words, before considering the contribution of any foreign species (e.g., Ca2+, CO32−, HCO3−) in the process of permeability loss, the own contribution of iron corrosion should be properly discussed. The contribution of iron corrosion to permeability loss is obvious and has been firstly experimentally documented by Luo et al. (2013) using deionized water (Yang et al., 2021). Properly considering the expansive nature of iron corrosion has prompted Domga et al. (2015) to recommend only hybrid systems (e.g., Fe0/sand) for sustainable filters. According to Naseri et al. (2017), in testing a Fe0 system, the main interest should be to check whether the designed system generated enough contaminant scavengers for efficient water treatment, regardless from the redox properties or even the chemical nature of the pollutants. It is recalled that Fe0 filters also remove pathogens (You et al., 2005; Bradley et al., 2011; Tepong-Tsindé et al., 2019; Kulkarni et al., 2020) and many other species without redox properties (Richardson and Nicklow 2002; Henderson and Demond 2007; Ullah et al., 2020).
Starting a New Area in Fe0 Research
The core problem of the Fe0 remediation literature is that tools such as the first-order rate constants (kobs) or the specific reaction rate first-order constants (kSA) premised on the misconception that Fe0 is a reducing agent have had an adverse effect on its development. The EE concept is such a questionable tool. Rather than help the Fe0 research community to exploit the mainstream corrosion science, these inappropriate tools have isolated the community to a modern knowledge system, reasoning circularly on an avoidable mistake introduced by some few individuals nearly 3 decades ago. Yet despite several efforts highlighting the mistakes, the scientific community continues to propagate the same errors (Xiao et al., 2020a; Xiao et al., 2020b; Cao et al., 2020; Hu et al., 2020; Cao et al., 2021a; Cao et al., 2021b; Hu et al., 2021). Consequently, in terms of progress the Fe0 remediation technology has not progressed much in the last decade, a scenario referred to as the “lost decade” (Noubactep, 2019). The Fe0 research community cannot afford to further lose time by further perpetuating the errors of yesteryear, hence the need to root any further research on the correct mechanistic understanding of iron corrosion (Makota et al., 2017; Cao et al., 2020; Hu et al., 2021, Huang et al., 2021). Therefore, to design better systems, there is no other option besides the following: i) to come back to the historical work of Khudenko (1991) as a starting point, and ii) consider progress made by some few research groups during the last decades (Gheju, 2011; Ghauch, 2015; Noubactep, 2015). It is only on the basis of this consideration that research on designing efficient and sustainable Fe0 will progress in the next coming decades.
Notably, abandoning the view that Fe0 is an environmental reducing agent will not fix everything at once. It will take time to acquire relevant data pertaining to the specificity and long-term kinetics of iron corrosion as used in environmental remediation and water treatment. Further, it takes time for research funding institutions to adapt their funding practices to support a research in which pilot tests shall last for years exceeding the 2–3 years typical of most funded research project. It is particularly obvious that the common 2 or 3 years grants are not suitable for such research efforts. It is important to bear in mind that, Fe0 remediation research is going back to its roots: Corrosion Science (Whitney, 1903; Howard, 1910; Dickerson et al., 1979; Nesic, 2007).
The change in strategic direction suggested in this communication is seemingly controversial, because it refutes the consensus of the late 1990s (Gillham and O’ Hannesin, 1994). However, the consensual approach has not worked during the past 2 decades, resulting in unsatisfactory technical systems (Morrison et al., 2006; Ngai et al., 2007; Comba et al., 2011; Mueller et al., 2011; Kowalski and Sø gaard, 2014; Bretzler et al., 2020; Ogata et al., 2020; Huang et al., 2021b; Mueller et al., 2021). There is no alternative to abandoning the misleading concept for mere conveniences since the aspirations of younger scientists are yet to be met.
A further argument against the “reducing Fe0” is presented by Ito and colleagues (Satur et al., 2007; Tabelin et al., 2017a; Tabelin et al., 2017b; Seng et al., 2019; Parka et al., 2020). It took 1 decade to Ito and his collaborators to establish Fe0 addition as the most promising tool to suppress pyrite oxidation under environmental conditions (Seng et al., 2019). The idea of the so called carrier-microencapsulation (CME) technique, developed during the second half of the 2000s (Satur et al., 2007), is to coat pyrite with a layer of metal hydroxide or oxide, in order to stop mineral oxidative dissolution which causes acid mine drainage (AMD) (Tabelin et al., 2017a; Tabelin et al., 2017b). Several Al, Fe and Ti complexes with organics were tested over the years but were not really specific to FeS2 in mine tailings and waste rocks. The bright idea, based on the electrochemistry of Fe0 (E0 = −0.44 V) and FeS2 (E0 = 0.25 V), was that, in a Fe0/FeS2 mixture, Fe0 is the anode and dissolved Fe2+ migrates to the cathode (FeS2) which is passivated upon formation of an oxide scale. Seng et al. (2019) have verified this sound hypothesis using Al0 and Fe0. Interested readers are referred to the related publications. For the presentation herein, its suffices to recall that the semi-conductives properties of FeS2 have been regarded as the reason for enhanced efficiency of Fe0/FeS2 systems (Xiao et al., 2020a; Xiao et al., 2020b; Hu et al., 2021). It is obvious that thermodynamics is not all but the results of Sheng et al. (2019) are a further call to revisit the operating mode of remediation Fe0/H2O systems.
The research group of the corresponding author and some few others have been working according to a new paradigm for a decade and have already achieved some good results than can be summarized as: i) Fe0 is not the source of electrons for the reduction of any contaminant. Tested species include organic (e.g., diclofenac, utriafol) and inorganic (e.g., Cr, Se) compounds (Gheju, 2011; Gheju, 2018; Gheju and Balcu, 2019), ii) the Fe0/H2O system is ionic selective, negatively charged species are favoured (Phukan et al., 2015; Phukan et al., 2016), iii) only hybrid filtration systems (e.g., Fe0/pumice, Fe0/sand) are sustainable (Ghauch, 2015; Noubactep, 2016), iv) each Fe0 has a different corrosion rate which is never constant (Btatkeu et al., 2013; Lufingo et al., 2019; Hildebrant et al., 2020), and v) Fe0 filters are a special case of “corrosion in porous media” (Ndé -Tchoup é et al., 2018; Yang et al., 2021). Yang et al. (2021) have recently introduced the most really holistic attempt to predict the service life of Fe0 filters. They considered for the first time the Faraday’s law to tentatively predict the time to clogging. The limitation is that there are no reliable corrosion rates available, this limitation is common to all branches of science investigating corrosion in porous media, including corrosion of reinforcing steel in concrete (Caré et al., 2008; Zhao et al., 2011; Stefanoni et al., 2018; Stefanoni et al., 2019). Therefore, long-term data for iron corrosion are needed (Noubactep, 2022).
Concluding Remarks
Fe0/H2O systems have a long history of application in environmental remediation and water treating, and several new applications are emerging. However, the Fe0 technology suffers from a number of misconceptions and mistakes pertaining to the mechanisms of contaminant removal. In the current communication, evidence was presented highlighting the mistakes of the electron efficiency concept, based on the misunderstanding that contaminant removal occurs according to reaction stoichiometry involving Fe0 as electron donor (electrochemical mechanism). A number of lines of evidence were presented to demonstrate the invalidity of the EE concept, including: i) the lag time widely reported in Fe0/H2O systems, ii) the universal formation of a non-conductive oxide layer that acts as a barrier for electron transfer, and iii) lack of mass balance data confirming its validity. Despite these limitations, and several studies demonstrating more plausible mechanisms based on iron corrosion, the bulk of the Fe0 research community continues to propagate the kSA, kobs and electron efficiency concepts. As a result, Fe0 research has been nearly stagnant for the past 2 decades, a scenario that has constrained the development of robust and efficient Fe0/H2O systems. Hence, this communication highlighted the need for a paradigm shift from flawed historical concepts, to a future where the design of the next generation of Fe0 is rooted on sound fundamental principles based on the science of iron corrosion. The suggested approach would enable an improved exploitation of the capacity of Fe0 technology beyond it actual stand as summarized by Antia (2020). To achieve this, a critical evidence based on the reactivity and long-term kinetics of Fe0 is needed, and research to generate such evidence requires long-term funding commitments.
Author Contributions
Conception (RH and CN), developing the original idea (RH, CN, AN, and VC), literature review (all), supervision (RH, CN and WG), redaction and revisions (all).
Funding
This work is supported by the Ministry of Science and Technology of China through the Programs “Research on Mechanism of Groundwater Exploitation and Seawater Intrusion in Coastal Areas” (Project Code 20165037412), “Driving process and mechanism of three-dimensional spatial distribution of high-risk organic pollutants in multi field coupled sites” (Project Code 2019YFC1804303) and Postgraduate Research and Practice Innovation Program of Jiangsu Province (Project Code: SJKY19_0519, 2019B60214).
Conflict of Interest
The authors declare that the research was conducted in the absence of any commercial or financial relationships that could be construed as a potential conflict of interest.
Publisher’s Note
All claims expressed in this article are solely those of the authors and do not necessarily represent those of their affiliated organizations, or those of the publisher, the editors and the reviewers. Any product that may be evaluated in this article, or claim that may be made by its manufacturer, is not guaranteed or endorsed by the publisher.
Acknowledgments
Esther Laurentine Nya (University of Maroua - Cameroon) is thanked for technical support.
Supplementary Material
The Supplementary Material for this article can be found online at: https://www.frontiersin.org/articles/10.3389/fenvc.2021.677813/full#supplementary-material
References
Anderson, M. A. (1989). Fundamental aspects of selenium removal by Harza process. Sacramento: Rep San Joaquin Valley Drainage Program, US Dep Interior.
Antia, D. D. J. (2020). “Water treatment and desalination using the eco-materials n-Fe0 (ZVI), n-Fe3O4, n-FexOyHz[mH2O], and n-Fex[Cation]nOyHz[Anion]m [rH2O],” in Handbook of Nanomaterials and Nanocomposites for Energy and Environmental Applications. Editor O. V. Kharissova (Switzerland AG: Springer Nature), 1–85. doi:10.1007/978-3-030-11155-7_66-1
Archer, W. L. (1979). Comparison of Chlorinated Solvent-Aluminum Reaction Inhibitors. Ind. Eng. Chem. Prod. Res. Dev. 18, 131–135. doi:10.1021/i360070a011
Bafghi, M. S., Zakeri, A., Ghasemi, Z., and Adeli, M. (2008). Reductive dissolution of manganese ore in sulfuric acid in the presence of iron metal. Hydrometallurgy 90, 207–212. doi:10.1016/j.hydromet.2007.07.003
Banerji, T., and Chaudhari, S. (2017). “A cost-effective technology for arsenic removal: Case study of zerovalent iron-based IIT Bombay arsenic filter in West Bengal,” in Water and Sanitation in the New Millennium. Editors K. Nath, and V. Sharma (New Delhi: Springer). doi:10.1007/978-81-322-3745-7_11
Bigg, T., and Judd, S. J. (2000). Zero-valent iron for water treatment. Environ. Tech. 21, 661–670. doi:10.1080/09593332108618077
Bischof, G. (18731873). The purification of water: Embracing the action of spongy iron on impure water. Glasgow: Bell and Bain, 19.
Bojic, A. L., Bojic, D., and Andjelkovic, T. (2009). Removal of Cu2+ and Zn2+ from model wastewaters by spontaneous reduction-coagulation process in flow conditions. J. Hazard. Mater. 168, 813–819. doi:10.1016/j.jhazmat.2009.02.096
Bradley, I., Straub, A., Maraccini, P., Markazi, S., and Nguyen, T. H. (2011). Iron oxide amended biosand filters for virus removal. Water Res. 45, 4501–4510. doi:10.1016/j.watres.2011.05.045
Brenner, S. (2010). Sequences and consequences. Phil. Trans. R. Soc. B 365, 207–212. doi:10.1098/rstb.2009.0221
Bretzler, A., Nikiema, J., LalanneHoffmann, F. L., Hoffmann, L., Biswakarma, J., Siebenaller, L., et al. (2020). Arsenic removal with zero-valent iron filters in Burkina Faso: Field and laboratory insights. Sci. Total Environ. 737, 139466. doi:10.1016/j.scitotenv.2020.139466
Brondel, D., Edwards, R., Hayman, A., Hill, D., Mehta, S., and Semerad, T. (1994). Corrosion in the Oil Industry. Oilfield Rev. 6, 4–18.
Btatkeu K., B. D., Miyajima, K., Noubactep, C., and Caré, S. (2013). Testing the suitability of metallic iron for environmental remediation: Discoloration of methylene blue in column studies. Chem. Eng. J. 215-216, 959–968. doi:10.1016/j.cej.2012.11.072
Btatkeu-K, B. D., Olvera-Vargas, H., Tchatchueng, J. B., Noubactep, C., and Caré, S. (2014a). Characterizing the impact of MnO2 on the [47-49]efficiency of Fe0-based filtration systems. Chem. Eng. J. 250, 416–422. doi:10.1016/j.cej.2014.04.059
Btatkeu-K, B. D., Olvera-Vargas, H., Tchatchueng, J. B., NoubactepCaré, C. S., and Caré, S. (2014b). Determining the optimum Fe0 ratio for sustainable granular Fe0/sand water filters. Chem. Eng. J. 247, 265–274. doi:10.1016/j.cej.2014.03.008
Buskirk, A., and Baradaran, H. (2009). Can reaction mechanisms be proven. J. Chem. Educ. 86, 551–554. doi:10.1021/ed086p551
Calabrò, P. S., Bilardi, S., and Moraci, N. (2021). Advancements in the use of filtration materials for the removal of heavy metals from multicontaminated solutions. Curr. Opin. Environ. Sci. Health 20, 100241. doi:10.1016/j.coesh.2021.100241
Cao, V., Alyoussef, G., Gatcha-Bandjun, N., Gwenzi, W., and Noubactep, C. (2021a). Characterizing the impact of MnO2 addition on the efficiency of Fe0/H2O systems. Sci. Rep. 11, 9814. doi:10.1038/s41598-021-89318-w
Cao, V., Alyoussef, G., Gatcha-Bandjun, N., Gwenzi, W., and Noubactep, C. (2021b). The key role of contact time in elucidating the mechanisms of enhanced decontamination by Fe0/MnO2/sand systems. Sci. Rep. 11, 12069. doi:10.1038/s41598-021-91475-x
Cao, V., Alyoussef, G., Gatcha-Bandjun, N., Gwenzi, W., and Noubactep, C. (2021c). The suitability of methylene blue discoloration (MB method) to investigate the Fe0/MnO2 system. Processes 9, 548. doi:10.3390/pr9030548
Cao, V., Ndé-Tchoupé, A. I., Hu, R., Gwenzi, W., and Noubactep, C. (2021d). The mechanism of contaminant removal in Fe(0)/H2O systems: The burden of a poor literature review. Chemosphere 280, 130614. doi:10.1016/j.chemosphere.2021.130614
Cao, V., Yang, H., Ndé-Tchoupé, A. I., Hu, R., Gwenzi, W., and Noubactep, C. (2020). Tracing the scientific history of Fe0-based environmental remediation prior to the advent of permeable reactive barriers. Processes 8, 977. doi:10.3390/pr8080977
Caré, S., Crane, R., Calabrò, P. S., Ghauch, A., Temgoua, E., and Noubactep, C. (2013). Modeling the permeability loss of metallic iron water filtration systems. Clean. Soil Air Water 41, 275–282. doi:10.1002/clen.201200167
Caré, S., Nguyen, Q. T., L'Hostis, V., and Berthaud, Y. (2008). Mechanical properties of the rust layer induced by impressed current method in reinforced mortar. Cement Concrete Res. 38, 1079–1091. doi:10.1016/j.cemconres.2008.03.016
Chamberlin, T. C. (1890). The method of multiple working hypotheses. Science ns-15, 92–96. doi:10.1126/science.ns-15.366.92
Charlet, L., Silvester, E., and Liger, E. (1998). N-compound reduction and actinide immobilisation in surficial fluids by Fe(II): the surface FeIIIOFeIIOH° species, as major reductant. Chem. Geology. 151, 85–93. doi:10.1016/s0009-2541(98)00072-2
Chaudhari, S., Banerji, T., and Kumar, P. R. (2014). “Domestic- and Community-Scale Arsenic Removal Technologies Suitable for Developing Countries,” in Water Reclamation and Sustainability. Editor S. Ahuja (Elsevier), 155–182. doi:10.1016/b978-0-12-411645-0.00007-9
Chen, Q., Fan, G., Na, W., Liu, J., Cui, J., and Li, H. (2019). Past, present, and future of groundwater remediation research: A scientometric analysis. Ijerph 16, 3975. doi:10.3390/ijerph16203975
Comba, S., Di Molfetta, A., and Sethi, R. (2011). A Comparison between field applications of nano-, micro-, and millimetric zero-valent iron for the remediation of contaminated aquifers. Water Air Soil Pollut. 215, 595–607. doi:10.1007/s11270-010-0502-1
Das, S., Lindsay, M. B. J., Essilfie-Dughan, J., and Hendry, M. J. (2017). Dissolved selenium(VI) removal by zero-valent iron under oxic conditions: Influence of sulfate and nitrate. ACS Omega 2, 1513–1522. doi:10.1021/acsomega.6b00382
Davis, F. (1891). An Elementary Handbook on Potable Water. New York, Boston, Chicago/USA: Silver, Burdett & Co, 118.
Devlin, J. F., and Allin, K. O. (2005). Major anion effects on the kinetics and reactivity of granular iron in glass-encased magnet batch reactor experiments. Environ. Sci. Technol. 39, 1868–1874. doi:10.1021/es040413q
Devonshire, E. (1890). The purification of water by means of metallic iron. J. Franklin Inst. 129, 449–461. doi:10.1016/0016-0032(90)90189-p
Dickerson, R. E., Gray, H. B., and Haight, G. P. (1979). Chemical Principles. Edition, 3. Amsterdam: Benjamin/Cummings Inc. London, 944.
Domga, R., Togue-Kamga, F., Noubactep, C., and Tchatchueng, J.-B. (2015). Discussing porosity loss of Fe0 packed water filters at ground level. Chem. Eng. J. 263, 127–134. doi:10.1016/j.cej.2014.10.105
Dong, G., Huang, L., Wu, X., Wang, C., Liu, Y., Liu, G., et al. (2018). Effect and mechanism analysis of MnO2 on permeable reactive barrier (PRB) system for the removal of tetracycline. Chemosphere 193, 702–710. doi:10.1016/j.chemosphere.2017.11.085
Erickson, A. J., Gulliver, J. S., and Weiss, P. T. (2007). Enhanced sand filtration for storm water phosphorus removal. J. Environ. Eng. 133, 485–497. doi:10.1061/(asce)0733-9372(2007)133:5(485)
Eusterhues, K., Rennert, T., Knicker, H., Kögel-Knabner, I., Totsche, K. U., and Schwertmann, U. (2011). Fractionation of Organic Matter Due to Reaction with Ferrihydrite: Coprecipitation versus Adsorption. Environ. Sci. Technol. 45, 527–533. doi:10.1021/es1023898
Fan, D., Lan, Y., Tratnyek, P. G., Johnson, R. L., Filip, J., O’Carroll, D. M., et al. (2017). Sulfidation of Iron-Based Materials: A Review of Processes and Implications for Water Treatment and Remediation. Environ. Sci. Technol. 51, 13070–13085. doi:10.1021/acs.est.7b04177
Farrell, J., Wang, J., O'Day, P., and Conklin, M. (2001). Electrochemical and spectroscopic study of arsenate removal from water using zero-valent iron media. Environ. Sci. Technol. 35, 2026–2032. doi:10.1021/es0016710
Furukawa, Y., Kim, J.-W., Watkins, J., and Wilkin, R. T. (2002). Formation of ferrihydrite and associated iron corrosion products in permeable reactive barriers of zero-valent iron. Environ. Sci. Technol. 36, 5469–5475. doi:10.1021/es025533h
Gatcha-Bandjun, N., Noubactep, C., and Loura Mbenguela, B. (2014). Water treatment with Fe0/H2O systems: Learning from internal electrolysis. Fresenius Environ. Bull. 23, 2663–2669.
Ghauch, A. (2015). Iron-based metallic systems: An excellent choice for sustainable water treatment. Freiberg Online Geosci. 32, 1–80.
Gheju, M., and Balcu, I. (2019). Sustaining the efficiency of the Fe(0)/H2O system for Cr(VI) removal by MnO2 amendment. Chemosphere 214, 389–398. doi:10.1016/j.chemosphere.2018.09.129
Gheju, M. (2011). Hexavalent chromium reduction with zero-valent iron (ZVI) in aquatic systems. Water Air Soil Pollut. 222, 103–148. doi:10.1007/s11270-011-0812-y
Gheju, M. (2018). Progress in Understanding the Mechanism of CrVI Removal in Fe0-Based Filtration Systems. Water 10, 651. doi:10.3390/w10050651
Gillham, R. W. (2008). “Development of the granular iron permeable reactive barrier technology (good science or good fortune),” in Advances in environmental geotechnics: proceedings of the International Symposium on Geoenvironmental Engineering in Hangzhou, China, September 8-10, 2007. Editors Y. Chen, X. Tang, and L. Zhan (Berlin/London: Springer), 5–15.
Gillham, R. W., and O'Hannesin, S. F. (1994). Enhanced degradation of halogenated aliphatics by zero-valent iron. Ground Water 32, 958–967. doi:10.1111/j.1745-6584.1994.tb00935.x
Gottinger, A. M., McMartin, D. W., Wild, D. J., and Moldovan, B. (2013). Integration of zero valent iron sand beds into biological treatment systems for uranium removal from drinking water wells in rural Canada. Can. J. Civ. Eng. 40, 945–950. doi:10.1139/cjce-2012-0512
Gould, J. P. (1982). The kinetics of hexavalent chromium reduction by metallic iron. Water Res. 16, 871–877. doi:10.1016/0043-1354(82)90016-1
Guan, X., Sun, Y., Qin, H., Li, J., Lo, I. M. C., He, D., et al. (2015). The limitations of applying zero-valent iron technology in contaminants sequestration and the corresponding countermeasures: The development in zero-valent iron technology in the last two decades (1994-2014). Water Res. 75, 224–248. doi:10.1016/j.watres.2015.02.034
Han, S., Huang, Y., and Liu, Z. (2019). Bacterial indicator reduction in dairy manure using hybrid zero-valent iron (h-ZVI) system. Environ. Sci. Pollut. Res. 26, 10790–10799. doi:10.1007/s11356-019-04501-x
Hao, Z. W., Xu, X. H., Jin, J., He, P., Liu, Y., and Wang, D. H. (2005). Simultaneous removal of nitrate and heavy metals by iron metal. J. Zhejiang Univ. Sci. B 6, 307–310. doi:10.1631/jzus.2005.B0307
He, F., Gong, L., Fan, D., Tratnyek, P. G., and Lowry, G. V. (2020). Quantifying the efficiency and selectivity of organohalide dechlorination by zerovalent iron. Environ. Sci. Process. Impacts 22, 528–542. doi:10.1039/c9em00592g
Henderson, A. D., and Demond, A. H. (2011). Impact of solids formation and gas production on the permeability of ZVI PRBs. J. Environ. Eng. 137, 689–696. doi:10.1061/(asce)ee.1943-7870.0000383
Henderson, A. D., and Demond, A. H. (2007). Long-term performance of zero-valent iron permeable reactive barriers: a critical review. Environ. Eng. Sci. 24, 401–423. doi:10.1089/ees.2006.0071
Hildebrant, B., Ndé-Tchoupé, A. I., Lufingo, M., Licha, T., and Noubactep, C. (2020). Steel wool for water treatment: Intrinsic reactivity and defluoridation efficiency. Processes 8, 265. doi:10.3390/pr8030265
Howard, R. S. (1910). “The Corrosion of Iron and its Alloys,” in Bachelor Thesis in Chemistry (Illinois: University of Illinois).
Hu, R., Cui, X., Xiao, M., Gwenzi, W., and Noubactep, C. (2021). Characterizing the impact of pyrite addition on the efficiency of Fe0/H2O systems. Sci. Rep. 11, 2326. doi:10.1038/s41598-021-81649-y
Hu, R., Yang, H., Tao, R., Cui, X., Xiao, M., Amoah, B. K., et al. (2020). Metallic iron for environmental remediation: Starting an overdue progress in knowledge. Water 12, 641. doi:10.3390/w12030641
Huang, C.-P., Wang, H.-W., and Chiu, P.-C. (1998). Nitrate reduction by metallic iron. Water Res. 32, 2257–2264. doi:10.1016/s0043-1354(97)00464-8
Huang, Y. H., and Zhang, T. C. (2005). Effects of dissolved oxygen on formation of corrosion products and concomitant oxygen and nitrate reduction in zero-valent iron systems with or without aqueous Fe2+. Water Res. 39, 1751–1760. doi:10.1016/j.watres.2005.03.002
Huang, Z., Cao, V., Nya, E. L., Gwenzi, W., and Noubactep, C. (2021b). Kanchan arsenic filters and the future of Fe0-based filtration systems for single household drinking water supply. Processes 9, 58.
Huang, Z., Nya, E. L., Cao, V., Gwenzi, W., Rahman, M. A., and Noubactep, C. (2021a). Universal access to safe drinking water: Escaping the traps of non-frugal technologies. Sustainability 13, 9645. doi:10.3390/su13179645
Hussam, A., and Munir, A. K. M. (2007). A simple and effective arsenic filter based on composite iron matrix: Development and deployment studies for groundwater of Bangladesh. J. Environ. Sci. Health A 42, 1869–1878. doi:10.1080/10934520701567122
ITRC (2011). Permeable reactive barrier: technology update. Washington, D.C: Interstate Technology & Regulatory Council ITRC.
James, B. R., Rabenhorst, M. C., and Frigon, G. A. (1992). Phosphorus sorption by peat and sand amended with iron oxides or steel wool. Water Environ. Res. 64, 699–705. doi:10.2175/wer.64.5.6
Jeen, S.-W., Yang, Y., Gui, L., and Gillham, R. W. (2013). Treatment of trichloroethene and hexavalent chromium by granular iron in the presence of dissolved CaCO3. J. Contaminant Hydrol. 144, 108–121. doi:10.1016/j.jconhyd.2012.11.004
Jiao, Y., Qiu, C., Huang, L., Wu, K., Ma, H., Chen, S., et al. (2009). Reductive dechlorination of carbon tetrachloride by zero-valent iron and related iron corrosion. Appl. Catal. B: Environ. 91, 434–440. doi:10.1016/j.apcatb.2009.06.012
Johnson, T. L., Scherer, M. M., and Tratnyek, P. G. (1996). Kinetics of halogenated organic compound degradation by iron metal. Environ. Sci. Technol. 30, 2634–2640. doi:10.1021/es9600901
Khudenko, B. M. (1987). Mathematical Models of Cementation Processes. J. Environ. Eng. 113, 681–702. doi:10.1061/(asce)0733-9372(1987)113:4(681)
Khudenko, B. M. (1985). Mechanism and kinetics of cementation processes. Water Sci. Technol. 17, 719–731. doi:10.2166/wst.1985.0174
Kim, S., Eckart, K., Sabet, S., Chiu, P. C., Sapkota, A. R., Handy, E. T., et al. (2021). Escherichia coli Reduction in Water by Zero-Valent Iron-Sand Filtration Is Based on Water Quality Parameters. Water 13, 2702. doi:10.3390/w13192702
Kowalski, K. P., and Søgaard, E. G. (2014). Implementation of zero-valent iron (ZVI) into drinking water supply - Role of the ZVI and biological processes. Chemosphere 117, 108–114. doi:10.1016/j.chemosphere.2014.05.088
Kulkarni, P., Olson, N. D., Bui, A. Q., Bradshaw, R. N., Del Collo, L. P., Hittle, L. E., et al. (2020). Zero-valent iron sand filtration can reduce human and plant pathogenic bacteria while increasing plant growth promoting bacteria in reclaimed water. Front. Environ. Sci. 8, 541921. doi:10.3389/fenvs.2020.541921
Lacy, W. J. (1952). Removal of radioactive material from water by slurrying with powdered metal. J. - Am. Water Works Assoc. 44, 824–828. doi:10.1002/j.1551-8833.1952.tb15859.x
Landolt, D. (2007). Corrosion and Surface Chemistry of Metals. First edition. Lausanne: EPFL Press, 615.
Lanet, P., Deluchat, V., and Baudu, M. (2021). Relevant design parameters for a reactor used in P removal with ZVI-based materials. J. Ind. Eng. Chem. 104, 8–21. doi:10.1016/j.jiec.2021.08.005
Latrach, L., Ouazzani, N., Hejjaj, A., Mahi, M., Masunaga, T., and Mandi, L. (2018). Two-stage vertical flow multi-soil-layering (MSL) technology for efficient removal of coliforms and human pathogens from domestic wastewater in rural areas under arid climate. Int. J. Hyg. Environ. Health 221, 64–80. doi:10.1016/j.ijheh.2017.10.004
Lauderdale, R. A., and Emmons, A. H. (1951). A method for decontaminating small volumes of radioactive water. J. Am. Water Works Assoc. 43, 327–331. doi:10.1002/j.1551-8833.1951.tb18948.x
Lavine, B. K., Auslander, G., and Ritter, J. (2001). Polarographic studies of zero valent iron as a reductant for remediation of nitroaromatics in the environment. Microchemical J. 70, 69–83. doi:10.1016/s0026-265x(01)00075-3
Lazzari, L. (2008). General aspects of corrosion, Vol. V. Rome, Italy: Encyclopedia of Hydrocarbons, Istituto Enciclopedia Italiana. Chapter 9.1.
Lee, G., Rho, S., and Jahng, D. (2004). Design considerations for groundwater remediation using reduced metals. Korean J. Chem. Eng. 21, 621–628. doi:10.1007/bf02705496
Li, J., Dou, X., Qin, H., Sun, Y., Yin, D., and Guan, X. (2019). Characterization methods of zerovalent iron for water treatment and remediation. Water Res. 148, 70–85. doi:10.1016/j.watres.2018.10.025
Lipczynska-Kochany, E., Harms, S., Milburn, R., Sprah, G., and Nadarajah, N. (1994). Degradation of carbon tetrachloride in the presence of iron and sulphur containing compounds. Chemosphere 29, 1477–1489. doi:10.1016/0045-6535(94)90279-8
Liu, H., Wang, Q., Wang, C., and Li, X.-z. (2013). Electron efficiency of zero-valent iron for groundwater remediation and wastewater treatment. Chem. Eng. J. 215-216, 90–95. doi:10.1016/j.cej.2012.11.010
Lufingo, M., Ndé-Tchoupé, A. I., Hu, R., Njau, K. N., and Noubactep, C. (2019). A novel and facile method to characterize the suitability of metallic iron for water treatment. Water 11, 2465. doi:10.3390/w11122465
Luo, P., Bailey, E. H., and Mooney, S. J. (2013). Quantification of changes in zero valent iron morphology using X-ray computed tomography. J. Environ. Sci. 25, 2344–2351. doi:10.1016/s1001-0742(12)60237-x
Makota, S., Nde-Tchoupe, A. I., Mwakabona, H. T., Tepong-Tsindé, R., Noubactep, C., Nassi, A., et al. (2017). Metallic iron for water treatment: Leaving the valley of confusion. Appl. Water Sci. 7, 4177–4196. doi:10.1007/s13201-017-0601-x
Matheson, L. J., and Tratnyek, P. G. (1994). Reductive dehalogenation of chlorinated methanes by iron metal. Environ. Sci. Technol. 28, 2045–2053. doi:10.1021/es00061a012
McGeough, K. L., Kalin, R. M., and Myles, P. (2007). Carbon disulfide removal by zero valent iron. Environ. Sci. Technol. 41, 4607–4612. doi:10.1021/es062936z
McMurty, D. C., and Elton, R. O. (1985). New approach to in-situ treatment of contaminated groundwaters. Environ. Progr. 4/3, 168–170.
Melchers, R. E., and Petersen, R. B. (2018). A reinterpretation of the Romanoff NBS data for corrosion of steels in soils. Corrosion Eng. Sci. Tech. 53, 131–140. doi:10.1080/1478422x.2017.1417072
Mikhail Khudenko, B. (1991). Feasibility evaluation of a novel method for destruction of organics. Water Sci. Technol. 23, 1873–1881. doi:10.2166/wst.1991.0643
Miyajima, K., and Noubactep, C. (2015). Characterizing the impact of sand addition on the efficiency of granular iron for contaminant removal in batch systems. Chem. Eng. J. 262, 891–896. doi:10.1016/j.cej.2014.10.038
Morrison, S. J., Mushovic, P. S., and Niesen, P. L. (2006). early breakthrough of molybdenum and uranium in a permeable reactive barrier. Environ. Sci. Technol. 40, 2018–2024. doi:10.1021/es052128s
Mueller, B., Dangol, B., Ngai, T. K. K., and Hug, S. J. (2021). Kanchan arsenic filters in the lowlands of Nepal: mode of operation, arsenic removal, and future improvements. Environ. Geochem. Health 43, 375–389. doi:10.1007/s10653-020-00718-9
Mueller, N. C., Braun, J., Bruns, J., Černík, M., Rissing, P., Rickerby, D., et al. (2011). Application of nanoscale zero valent iron (NZVI) for groundwater remediation in Europe. Environ. Sci. Pollut. Res. 19, 550–558. doi:10.1007/s11356-011-0576-3
Murphy, A. P. (1988). Removal of selenate from water by chemical reduction. Ind. Eng. Chem. Res. 27, 181–191. doi:10.1021/ie00073a033
Mwakabona, H. T., Ndé-Tchoupé, A. I., Njau, K. N., Noubactep, C., and Wydra, K. D. (2017). Metallic iron for safe drinking water provision: Considering a lost knowledge. Water Res. 117, 127–142. doi:10.1016/j.watres.2017.03.001
Naseri, E., Ndé-Tchoupé, A., Mwakabona, H., Nanseu-Njiki, C., Noubactep, C., Njau, K., et al. (2017). Making Fe0-Based Filters a Universal Solution for Safe Drinking Water Provision. Sustainability 9, 1224. doi:10.3390/su9071224
Ndé-Tchoupé, A. I., Hu, R., Gwenzi, W., Nassi, A., and Noubactep, C. (2020). Characterizing the reactivity of metallic iron for water treatment: H2 evolution in H2SO4 and uranium removal efficiency. Water 12, 1523. doi:10.3390/w12061523
Ndé-Tchoupé, A., Makota, S., Nassi, A., Hu, R., and Noubactep, C. (2018). The suitability of pozzolan as admixing aggregate for Fe0-based filters. Water 10, 417. doi:10.3390/w10040417
Nesic, S. (2007). Key issues related to modelling of internal corrosion of oil and gas pipelines – A review. Corros. Sci. 49, 4308–4338.
Neumann, A., Kaegi, R., Voegelin, A., Hussam, A., Munir, A. K. M., and Hug, S. J. (2013). Arsenic removal with composite iron matrix filters in Bangladesh: A field and laboratory study. Environ. Sci. Technol. 47, 4544–4554. doi:10.1021/es305176x
Ngai, T. K., Shrestha, R. R., Dangol, B., Maharjan, M., and Murcott, S. E. (2007). Design for sustainable development-household drinking water filter for arsenic and pathogen treatment in Nepal. J. Environ. Sci. Health A. Tox. Hazard. Subst. Environ. Eng. 42, 1879–1888. doi:10.1080/10934520701567148
Njaramba, L. K., Park, J.-B., Lee, C.-S., Nzioka, A. M., and Kim, Y.-J. (2021). Permeable reactive barriers with zero-valent iron and pumice for remediation of groundwater contaminated with multiple heavy metals. Environ. Eng. Sci. 38, 245–255. doi:10.1089/ees.2020.0109
Noubactep, C. (2008). A Critical Review on the Process of Contaminant Removal in Fe0-H2O Systems. Environ. Tech. 29, 909–920. doi:10.1080/09593330802131602
Noubactep, C. (2011). Aqueous contaminant removal by metallic iron: Is the paradigm shifting. Water SA 37, 419–426. doi:10.4314/wsa.v37i3.68493
Noubactep, C., Caré, S., and Crane, R. (2012). Nanoscale metallic iron for environmental remediation: prospects and limitations. Water Air Soil Pollut. 223, 1363–1382. doi:10.1007/s11270-011-0951-1
Noubactep, C., and Caré, S. (2010). On nanoscale metallic iron for groundwater remediation. J. Hazard. Mater. 182, 923–927. doi:10.1016/j.jhazmat.2010.06.009
Noubactep, C. (2010a). Elemental metals for environmental remediation: Learning from cementation process. J. Hazard. Mater. 181, 1170–1174. doi:10.1016/j.jhazmat.2010.05.085
Noubactep, C. (2014). Flaws in the design of Fe(0)-based filtration systems. Chemosphere 117, 104–107. doi:10.1016/j.chemosphere.2014.06.014
Noubactep, C., Licha, T., Scott, T. B., Fall, M., and Sauter, M. (2009). Exploring the influence of operational parameters on the reactivity of elemental iron materials. J. Hazard. Mater. 172, 943–951. doi:10.1016/j.jhazmat.2009.07.097
Noubactep, C., Meinrath, G., Dietrich, P., and Merkel, B. (2003). Mitigating uranium in groundwater: Prospects and limitations. Environ. Sci. Technol. 37, 4304–4308. doi:10.1021/es034296v
Noubactep, C. (2015). Metallic iron for environmental remediation: a review of reviews. Water Res. 85, 114–123. doi:10.1016/j.watres.2015.08.023
Noubactep, C. (2020). “Metallic iron for environmental remediation: prospects and limitations,” in Chap. 36, A Handbook of Environmental Toxicology: Human Disorders and Ecotoxicology. Editor J. P. F. D’Mello (Wallingford: CAB International), 531544–544. doi:10.1079/9781786394675.0531
Noubactep, C. (2021). Metallic iron for the removal of metals and metalloids from aqueous solutions: an old-timer view. Curr. Opin. Environ. Sci. Health 22, 100256. doi:10.1016/j.coesh.2021.100256
Noubactep, C. (2007). Processes of Contaminant Removal in "Fe0-H2O" Systems Revisited: The Importance of Co-precipitation. Toenvirj 1, 9–13. doi:10.2174/1874233500701010009
Noubactep, C. (2013). Relevant Reducing Agents in Remediation Fe0/H2O Systems. Clean. Soil Air Water 41, 493–502. doi:10.1002/clen.201200406
Noubactep, C. (2016). Research on metallic iron for environmental remediation: Stopping growing sloppy science. Chemosphere 153, 528–530. doi:10.1016/j.chemosphere.2016.03.088
Noubactep, C. (2022). Should the term 'metallic iron' appear in the title of a research paper. Chemosphere 287, 132314. doi:10.1016/j.chemosphere.2021.132314
Noubactep, C. (2010b). The fundamental mechanism of aqueous contaminant removal by metallic iron. Water SA 36, 663–670. doi:10.4314/wsa.v36i5.62000
Noubactep, C. (2019). The operating mode of Fe0/H2O systems: Hidden truth or repeated nonsense. Fresenius Environ. Bull. 28, 8328–8330.
O'Hannesin, S. F., and Gillham, R. W. (1998). Long-term performance of an in situ "iron wall" for remediation of VOCs. Ground Water 36, 164–170. doi:10.1111/j.1745-6584.1998.tb01077.x
Obiri-Nyarko, F., Grajales-Mesa, S. J., and Malina, G. (2014). An overview of permeable reactive barriers for in situ sustainable groundwater remediation. Chemosphere 111, 243–259. doi:10.1016/j.chemosphere.2014.03.112
Odziemkowski, M. S., Schuhmacher, T. T., Gillham, R. W., and Reardon, E. J. (1998). Mechanism of oxide film formation on iron in simulating groundwater solutions: Raman spectroscopic studies. Corrosion Sci. 40, 371–389. doi:10.1016/s0010-938x(97)00141-8
Odziemkowski, M. S., and Simpraga, R. P. (2004). Distribution of oxides on iron materials used for remediation of organic groundwater contaminants - Implications for hydrogen evolution reactions. Can. J. Chem. 82, 1495–1506. doi:10.1139/v04-120
Ogata, R., Dangol, B., and Sakamoto, M. (2020). Sustainability assessment of long-term, widely used household Kanchan Arsenic Filters in Nepal. J. Environ. Sci. Health Part A 55, 517–527. doi:10.1080/10934529.2019.1710414
Oldright, G. L., Keyes, H. E., Miller, V., and Sloan, W. A. (1928). Precipitation of lead and copper from solution on sponge iron. BuMines B 281, 131.
Park, I., Tabelin, C. B., Seno, K., Jeon, S., Inano, H., Ito, M., et al. (2020). Carrier-microencapsulation of arsenopyrite using Al-catecholate complex: nature of oxidation products, effects on anodic and cathodic reactions, and coating stability under simulated weathering conditions. Heliyon 6, e03189. doi:10.1016/j.heliyon.2020.e03189
Phukan, M., Noubactep, C., and Licha, T. (2016). Characterizing the ion-selective nature of Fe 0/H 2 O systems in batch experiments. J. Environ. Chem. Eng. 4, 65–72. doi:10.1016/j.jece.2015.11.009
Phukan, M., Noubactep, C., and Licha, T. (2015). Characterizing the ion-selective nature of Fe0-based filters using azo dyes. Chem. Eng. J. 259, 481–491. doi:10.1016/j.cej.2014.08.013
Popat, V., and Padhiyar, N. (2013). Kinetic Study of Bechamp Process for P-Nitrotoluene Reduction to P-Toluidine. Ijcea 4, 401–405. doi:10.7763/ijcea.2013.v4.334
Qin, H., Guan, X., Bandstra, J. Z., Johnson, R. L., and Tratnyek, P. G. (2018). Modeling the Kinetics of Hydrogen Formation by Zerovalent Iron: Effects of Sulfidation on Micro- and Nano-Scale Particles. Environ. Sci. Technol. 52, 13887–13896. doi:10.1021/acs.est.8b04436
Rahman, M. A., Wiegand, B. A., Badruzzaman, A. B. M., and Ptak, T. (2013). Hydrogeological analysis of the upper Dupi Tila Aquifer, towards the implementation of a managed aquifer-recharge project in Dhaka City, Bangladesh. Hydrogeol. J. 21, 1071–1089. doi:10.1007/s10040-013-0978-z
Rangan, S. M., Mouti, A., LaPat-Polasko, L., Lowry, G. V., Krajmalnik-Brown, R., and Delgado, A. G. (2020). Synergistic Zerovalent Iron (Fe0) and Microbiological Trichloroethene and Perchlorate Reductions Are Determined by the Concentration and Speciation of Fe. Environ. Sci. Technol. 54 (22), 14422–14431. doi:10.1021/acs.est.0c05052
Reardon, E. J. (1995). Anaerobic corrosion of granular iron: Measurement and interpretation of hydrogen evolution rates. Environ. Sci. Technol. 29, 2936–2945. doi:10.1021/es00012a008
Reynolds, G. W., Hoff, J. T., and Gillham, R. W. (1990). Sampling bias caused by materials used to monitor halocarbons in groundwater. Environ. Sci. Technol. 24, 135–142. doi:10.1021/es00071a017
Rhodes, F. H., and Carty, J. T. (1925). The corrosion of certain metals by carbon tetrachloride. Ind. Eng. Chem. 17, 909–911. doi:10.1021/ie50189a012
Richardson, J. P., and Nicklow, J. W. (2002). In Situ Permeable Reactive Barriers for Groundwater Contamination. Soil Sediment. Contamination: Int. J. 11, 241–268. doi:10.1080/20025891106736
Roberts, A. L., Totten, L. A., Arnold, W. A., Burris, D. R., and Campbell, T. J. (1996). Reductive elimination of chlorinated ethylenes by zero-valent metals. Environ. Sci. Technol. 30, 2654–2659. doi:10.1021/es9509644
Romanoff, M. (1957). Underground Corrosion., 579. Circular: United States Department of Commerce, National Bureau of Standards.
Santisukkasaem, U., and Das, D. B. (2019). A non-dimensional analysis of permeability loss in zero-valent iron permeable reactive barrier (PRB). Transp Porous Med. 126, 139–159. doi:10.1007/s11242-018-1096-0
Satur, J., Hiroyoshi, N., Tsunekawa, M., Ito, M., and Okamoto, H. (2007). Carrier-microencapsulation for preventing pyrite oxidation. Int. J. Mineral Process. 83, 116–124. doi:10.1016/j.minpro.2007.06.003
Schreier, C. G., and Reinhard, M. (1994). Transformation of chlorinated organic compounds by iron and manganese powders in buffered water and in landfill leachate. Chemosphere 29, 1743–1753. doi:10.1016/0045-6535(94)90320-4
Seng, S., Tabelin, C. B., Kojima, M., Hiroyoshi, N., and Ito, M. (2019). Galvanic microencapsulation (GME) using zero-valent aluminum and zero-valent iron to suppress pyrite oxidation. Mater. Trans. 60, 277–286. doi:10.2320/matertrans.m-m2018851
Shufen, F., Jia, X., Jingyi, H., Weili, R., and Xilai, Z. (2018). Effectiveness of electron transfer and electron competition mechanism in zero-valent iron-based reductive groundwater remediation systems. Prog. Chem. 30, 1035.
Sikora, E., and Macdonald, D. D. (2000). The passivity of iron in the presence of ethylenediaminetetraacetic acid I. General electrochemical behavior. J. Electrochem. Soc. 147, 4087–4092. doi:10.1149/1.1394024
Song, D.-I., Kim, Y. H., and Shin, W. S. (2005). A simple mathematical analysis on the effect of sand in Cr(VI) reduction using zero valent iron. Korean J. Chem. Eng. 22, 67–69. doi:10.1007/bf02701464
Stefanoni, M., Angst, U. M., and Elsener, B. (2018). Electrochemistry and capillary condensation theory reveal the mechanism of corrosion in dense porous media. Sci. Rep. 8, 7407. doi:10.1038/s41598-018-25794-x
Stefanoni, M., Angst, U. M., and Elsener, B. (2019). Kinetics of electrochemical dissolution of metals in porous media. Nat. Mater. 18, 942–947. doi:10.1038/s41563-019-0439-8
Stratmann, M., and Müller, J. (1994). The mechanism of the oxygen reduction on rust-covered metal substrates. Corrosion Sci. 36, 327–359. doi:10.1016/0010-938x(94)90161-9
Sun, Y., Li, J., Huang, T., and Guan, X. (2016). The influences of iron characteristics, operating conditions and solution chemistry on contaminants removal by zero-valent iron: A review. Water Res. 100, 277–295. doi:10.1016/j.watres.2016.05.031
Tabelin, C. B., Veerawattananun, S., Ito, M., Hiroyoshi, N., and Igarashi, T. (2017b). Pyrite oxidation in the presence of hematite and alumina: II. Effects on the cathodic and anodic half-cell reactions. Sci. Total Environ. 581-582, 126–135. doi:10.1016/j.scitotenv.2016.12.050
Tabelin, C. B., Veerawattananun, S., Veerawattananun, S., Ito, M., Hiroyoshi, N., and Igarashi, T. (2017a). Pyrite oxidation in the presence of hematite and alumina: I. Batch leaching experiments and kinetic modeling calculations. Sci. Total Environ. 580, 687–698. doi:10.1016/j.scitotenv.2016.12.015
Tang, F., Xin, J., Zheng, X., Zheng, T., Yuan, X., and Kolditz, O. (2017). Effect of solution pH on aging dynamics and surface structural evolution of mZVI particles: H2 production and spectroscopic/microscopic evidence. Environ. Sci. Pollut. Res. 24, 23538–23548. doi:10.1007/s11356-017-9976-3
Tepong-Tsindé, R., Crane, R., Noubactep, C., Nassi, A., and Ruppert, H. (2015). Testing metallic iron filtration systems for decentralized water treatment at pilot scale. Water 7, 868–897. doi:10.3390/w7030868
Tepong-Tsindé, R., Ndé-Tchoupé, A. I., Noubactep, C., Nassi, A., and Ruppert, H. (2019). Characterizing a newly designed steel-wool-based household filter for safe drinking water provision: Hydraulic conductivity and efficiency for pathogen removal. Processes 7, 966. doi:10.3390/pr7120966
Thakur, A. K., Vithanage, M., Das, D. B., and Kumar, M. (2020). A review on design, material selection, mechanism, and modelling of permeable reactive barrier for community-scale groundwater treatment. Environ. Tech. Innovation 19, 100917. doi:10.1016/j.eti.2020.100917
Tian, J., Jin, J., Chiu, P. C., Cha, D. K., Guo, M., and Imhoff, P. T. (2019). A pilot-scale, bi-layer bioretention system with biochar and zero-valent iron for enhanced nitrate removal from stormwater. Water Res. 148, 378–387. doi:10.1016/j.watres.2018.10.030
Ullah, S., Guo, X., Luo, X., Zhang, X., Li, Y., and Liang, Z. (2020). The coupling of sand with ZVI/oxidants achieved proportional and highly efficient removal of arsenic. Front. Environ. Sci. Eng. 14, 94. doi:10.1007/s11783-020-1273-6
van Craenenbroeck, W. (1998). Easton & Anderson and the water supply of Antwerp (Belgium). Ind. Archaeology Rev. 20, 105–116. doi:10.1179/iar.1998.20.1.105
Velimirovic, M., Larsson, P.-O., Simons, Q., and Bastiaens, L. (2013). Reactivity screening of microscale zerovalent irons and iron sulfides towards different CAHs under standardized experimental conditions. J. Hazard. Mater. 252-253, 204–212. doi:10.1016/j.jhazmat.2013.02.047
Vollprecht, D., Krois, L.-M., Sedlazeck, K. P., Müller, P., Mischitz, R., Olbrich, T., et al. (2018). Removal of critical metals from waste water by zero-valent iron. J. Clean. Prod. 208, 1409–1420.
Vollprecht, D., Plessl, K., Neuhold, S., Kittinger, F., Öfner, W., MüllerMischitz, P. R., et al. (2020). Recovery of molybdenum, chromium, tungsten, copper, silver, and zinc from industrial waste waters using zero-valent iron and tailored beneficiation processes. Processes 8, 279. doi:10.3390/pr8030279
Wakatsuki, T., Esumi, H., and Omura, S. (1993). High Performance and N & P-Removable On-Site Domestic Waste Water Treatment System by Multi-Soil-Layering Method. High Performance N. P Removable On-site Domestic Wastewater treatment system by multi-soil-layering method. Water Sci. Technol. 27, 31–40. doi:10.2166/wst.1993.0010
Wang, Q., Song, X., Wei, C., Jin, P., Chen, X., Tang, Z., et al. (2022). In situ remediation of Cr(VI) contaminated groundwater by ZVI-PRB and the corresponding indigenous microbial community responses: a field-scale study. Sci. Total Environ. 805, 150260. doi:10.1016/j.scitotenv.2021.150260
Warren, K. D., Arnold, R. G., Bishop, T. L., Lindholm, L. C., and Betterton, E. A. (1995). Kinetics and mechanism of reductive dehalogenation of carbon tetrachloride using zero-valence metals. J. Hazard. Mater. 41, 217–227. doi:10.1016/0304-3894(94)00117-y
Weber, E. J. (1996). Iron-mediated reductive transformations: investigation of reaction mechanism. Environ. Sci. Technol. 30, 716–719. doi:10.1021/es9505210
White, A. F., and Peterson, M. L. (1996). Reduction of aqueous transition metal species on the surfaces of Fe(II) -containing oxides. Geochimica et Cosmochimica Acta 60, 3799–3814. doi:10.1016/0016-7037(96)00213-x
Whitney, W. R. (1903). The corrosion of iron. J. Am. Chem. Soc. 25 (4), 394–406. doi:10.1021/ja02006a008
Wilkin, R. T., Acree, S. D., Ross, R. R., Puls, R. W., Lee, T. R., and Woods, L. L. (2014). Fifteen-year assessment of a permeable reactive barrier for treatment of chromate and trichloroethylene in groundwater. Sci. Total Environ. 468-469, 186–194. doi:10.1016/j.scitotenv.2013.08.056
Wilkin, R. T., Lee, T. R., Sexton, M. R., Acree, S. D., Puls, R. W., Blowes, D. W., et al. (2019). Geochemical and isotope study of trichloroethene degradation in a zero-valent iron permeable reactive barrier: A twenty-two-year performance evaluation. Environ. Sci. Technol. 53, 296–306. doi:10.1021/acs.est.8b04081
Wu, C.-Q., Fu, D.-T., and Chen, X. (2014). Nitrobenzene degradation by micro-sized iron and electron efficiency evaluation. Chem. Pap. 68, 1350–1357. doi:10.2478/s11696-014-0573-3
Xiao, M., Cui, X., Hu, R., Gwenzi, W., and Noubactep, C. (2020a). Validating the efficiency of the FeS2 method for elucidating the mechanisms of contaminant removal using Fe0/H2O systems. Processes 8, 1162. doi:10.3390/pr8091162
Xiao, M., Hu, R., Cui, X., Gwenzi, W., and Noubactep, C. (2020b). Understanding the operating mode of Fe0/Fe-sulfide/H2O systems for water treatment. Processes 8, 409. doi:10.3390/pr8040409
Yang, H., Hu, R., Ruppert, H., and Noubactep, C. (2021). Modeling porosity loss in Fe0-based permeable reactive barriers with Faraday's law. Sci. Rep. 11, 16998. doi:10.1038/s41598-021-96599-8
You, Y., Han, J., Chiu, P. C., and Jin, Y. (2005). Removal and inactivation of waterborne viruses using zerovalent iron. Environ. Sci. Technol. 39, 9263–9269. doi:10.1021/es050829j
Zhang, W.-x. (2003). Nanoscale iron particles for environmental remediation: an overview. J. Nano. Res. 5, 323–332. doi:10.1023/a:1025520116015
Keywords: contaminant removal, electron efficiency, environmental remediation, zero-valent iron, safe drinking water
Citation: Hu R, Ndé-Tchoupé AI, Cao V, Gwenzi W and Noubactep C (2021) Metallic Iron for Environmental Remediation: The Fallacy of the Electron Efficiency Concept. Front. Environ. Chem. 2:677813. doi: 10.3389/fenvc.2021.677813
Received: 08 March 2021; Accepted: 11 October 2021;
Published: 29 October 2021.
Edited by:
Feiping Zhao, Central South University, ChinaReviewed by:
Xiangke Wang, North China Electric Power University, ChinaMingyang Xing, East China University of Science and Technology, China
Chen Tian, Central South University, China
Copyright © 2021 Hu, Ndé-Tchoupé, Cao, Gwenzi and Noubactep. This is an open-access article distributed under the terms of the Creative Commons Attribution License (CC BY). The use, distribution or reproduction in other forums is permitted, provided the original author(s) and the copyright owner(s) are credited and that the original publication in this journal is cited, in accordance with accepted academic practice. No use, distribution or reproduction is permitted which does not comply with these terms.
*Correspondence: Chicgoua Noubactep, Y25vdWJhY0Bnd2RnLmRl