- 1State Key Laboratory of Oil and Gas Reservoir Geology and Exploitation, Southwest Petroleum University, Chengdu, China
- 2The Center of New Energy Materials and Technology, School of New Energy and Materials, Southwest Petroleum University, Chengdu, China
The treatment of hazardous hydrogen sulfide (H2S) via photocatalysis technology has been known as one of the most promising green technologies. Photocatalytic production of hydrogen (H2) from H2S by two-dimensional (2D) semiconductor materials has gathered great attention owing to its large surface area and high catalytic activity. In this work, layered MoS2 has been successfully grown on TiO2 {001} surface to fabricate the 2D MoS2/TiO2 {001} composites for H2 evolution from H2S, which can be confirmed by the X-ray diffraction (XRD) and transmission electron microscopy (TEM) tests. Band structures and UV-Vis spectra provide important evidences that MoS2 loading can significantly narrow the band gap and broaden the light absorbance into the visible light region. Electron transfer is obviously visualized at the interface of MoS2/TiO2, resulting in the built-in potential from TiO2 to MoS2, which is determined by the density functional theory (DFT) calculations and X-ray photoelectron spectroscopy (XPS) test. Consequently, the photo-induced electrons and holes are accumulated at the sides of TiO2 and MoS2 under the illumination, respectively, which largely promote the interfacial electron transfer and prolong the lifetime of photo-generated electrons that participate in the photocatalytic reactions of H2 evolution from H2S. This efficient separation of photo-induced carriers can be further proved by photoluminescence (PL) spectra, photocurrent responses, and electrochemical impedance spectra. As a result, the photocatalytic activity of H2 evolution is largely increased by 9.4 times compared to the pristine TiO2. This study could offer a new and facile way to design highly efficient 2D photocatalysts for the application of H2S treatment.
Introduction
Hydrogen sulfide (H2S), as a highly toxic and corrosive pollutant, has been massively discharged from the natural gas extraction and oil refineries, which causes a serious threat on the air environment and human health with a fatal concentration over 700 ppm in the air. Thus, H2S treatment has become a long-standing issue that attracts great worldwide attention. Photocatalysis technology, directly driven by the solar energy, has been considered to be an effective new-generation green technologies (Liu et al., 2012, 2018, 2019; Wang et al., 2012; Ren et al., 2020). More importantly, clean energy H2 can be produced during the photo-splitting of H2S, which can alleviate the pressing global energy crisis and the environmental problems from the perspective of renewable resource utilization (Dan et al., 2017, 2019a,b; Xiang et al., 2019). Recently, anatase TiO2 nanosheet with exposed {001} facets has been proven to be a promising candidate for photocatalytic production of H2 because of the high reactivity and non-toxic features (Altomare et al., 2018; Chen et al., 2018; Ge et al., 2019; Yu et al., 2019), and the photocatalytic mechanism of H2 production from H2S on anatase TiO2 {001} was reported in our previous work (Cai et al., 2020). However, the wide band gap and high charge recombination enormously restrict its photocatalytic activity. Surface modification via noble metal loading such as Au and Ag is usually applied to design TiO2-based photocatalysts to pursue highly efficient production of H2 (Zhao et al., 2018; Wang et al., 2019; Sharma et al., 2020). The high cost and limited storage of noble metals are of serious concerns. Thus, it deserves to develop economy and efficient methods to modify TiO2 to achieve a high conversion activity of H2. Particularly, two-dimensional (2D) layered MoS2, as a non-noble metal catalyst, has attracted great attention because of the ability to capture electrons with the unique layered sandwich structure (Sun et al., 2018; Li Y. et al., 2019). Furthermore, various researches have reported that the layered MoS2 can act as an excellent cocatalyst for both photocatalytic and electrocatalytic evolution of H2 owing to the abundantly exposed edges (Li et al., 2011; Kibsgaard et al., 2012; Chang et al., 2014). It was shown that the fabrication of an MoS2/TiO2 heterostructure can largely promote the H2 production from H2O due to the much lower reaction energy barriers along the hydrogen evolution reaction paths (Tang et al., 2017). Other researchers also found that the MoS2 nanosheets grown on TiO2 could produce more active edges, and the efficient separation of the photo-generated carries resulted in the excellent H2 production from H2O (Liu Q. et al., 2013; Zhang et al., 2015). However, the promising MoS2/TiO2 composite has been barely applied to H2 evolution from H2S as yet, and the enhanced photocatalytic mechanism of H2 production deserves in-depth study.
In the present study, a facile two-step hydrothermal method has been adopted to prepare the layered MoS2 grown on TiO2 {001}. The morphology, component, optical properties, and interfacial electron transfer of the fabricated MoS2/TiO2 {001} are investigated in-depth by experimental tests such as transmission electron microscopy (TEM), X-ray photoelectron spectroscopy (XPS), and photoluminescence (PL) spectra as well as the density functional theory (DFT) calculations.
Experimental Method and Theoretical Calculation
TiO2 {001} Synthesis
TiO2 synthesis adopts a traditional hydrothermal method with the shape control agent HF (Yuan et al., 2016; Yang et al., 2018), as has been successfully prepared in our previous work (Yu et al., 2016). First, 3.75 ml of butyl titanate and 0.6 ml of HF is placed into a 15-ml of complete dried Teflon-lined stainless-steel autoclave. Then the autoclave is sealed and placed in an electric oven held at 200°C for 24 h. Then, 0.1 M NaOH solution is applied to neutralize the F− by stirring washing precipitates for 2 h after autoclave cooling to room temperature. Next, the precipitates are separated by vacuum filtration, washed with ultrapure water and alcohol several times until pH 7. Finally, the precipitates are dried overnight at 60°C in a vacuum oven.
Preparation of MoS2/TiO2 {001} (MoS2/TiO2) Composites
A two-step hydrothermal method is conducted to prepare the MoS2/TiO2 composites. First, 200 mg of TiO2 powder is placed into a 50-ml Teflon-lined stainless-steel autoclave containing 25 ml of ultrapure water. Then, 7.7 mg ammonium molybdate and 72.6 mg thiourea are added into the above autoclave. After stirring for 15 min, the autoclave is heated at 180°C for 24 h. The final powder is washed several times with ultrapure water and ethanol and dried at 60°C under a vacuum environment. Finally, the prepared composite is named as MoS2/TiO2-0.5. Other composites with 15.4, 30.8, and 46.2 mg of ammonium molybdate and 145.2, 290.4, and 435.6 mg of thiourea are ordered as MoS2/TiO2-1, MoS2/TiO2-2, and MoS2/TiO2-3.
Material Characterization
The powder structure and crystal phase of the samples are determined by X'pert powder X-ray diffraction (XRD) radiation operated at 40 kV/40 mA. UV-Vis spectrum is recorded at room temperature by Shimadzu UV-2600 spectrophotometer equipped with a double integrating sphere, and BaSO4 is used as the reflectance standard. XPS (Thermo ESCALAB 250XI) measurement is conducted to investigate the surface chemical environment of samples referenced to the C 1s level at 284.8 eV. TEM (JEM 2100F) tests are carried out to investigate the microstructure of samples. Energy dispersive X-ray spectroscopy (EDS) is used to obtain an elemental mapping. The photoluminescence (PL) spectra are tested with a fluorescence spectrophotometer (excitation at 340 nm). The progress of photocatalytic activity measurements is referenced to our previous work (Cai et al., 2020).
Theoretical Calculations
2D MoS2/TiO2 configuration is built with relaxed rectangular 5 × MoS2 monolayers and a relaxed four-layer 3 × 1 anatase TiO2 {001} surface. The crystal lattice match can be quantified by δ = |a001 – ag|/a001 (Lin et al., 2017), where a001 is the lattice constant of the relaxed anatase TiO2 {001} surface, and ag is the lattice constant of the relaxed MoS2 monolayer. The calculated δ for the lattice parameters a and b are 2.8 and 2.1% for the MoS2/TiO2, respectively, which are in a reasonable and acceptable range. The bottom Ti and O atoms of MoS2/TiO2 composites are fixed during the calculations, while other atoms are fully relaxed to simulate the interaction between TiO2 surface and MoS2 sheet. A vacuum space of 20 Å is applied to construct MoS2/TiO2 composites in order to avoid interactions between the periodic neighboring slabs. The Vienna ab initio simulation package (VASP) with the GGA-PBE exchange-correlation method is adopted in all calculation processes (Kresse and Furthmüller, 1996; Kresse and Joubert, 1999). The PAW method is used to describe the electron–ion interactions for Ti, O, Mo, and S atoms. Furthermore, to correct the strong interaction of Ti 3d and Mo 4d electron orbital, the coulombic interaction U and exchange energy J parameters are set according to the previous theoretical study (Cao et al., 2014). Supplementary Figure 1 showed the MoS2/TiO2 structure after geometry optimization. To study the structural stability of the MoS2/TiO2 composites, the interface binding energy (Eb) is obtained in Equation (1):
where ETM, EM, and ET are the total energies of relaxed MoS2/TiO2 composites, relaxed MoS2, and TiO2 surface.
Interfacial electron transfer between TiO2 and MoS2 can be investigated by the following Equation (2):
where ρTM, ρM, and ρT are the charge densities of the MoS2/TiO2 composites, MoS2 structure, and TiO2 surface, respectively. Furthermore, adsorption energy (Ead) is used to describe the interaction between surface and adsorbed molecule, and the more negative Ead value meant a more stable reaction progress, which can be calculated by the following Equation (3):
Eadsorbate/surface represented the energy of the surface with adsorbed molecule, Eadsorbate is the energy of solitary adsorbed molecule, and Esurface is the energy of surface.
Work function (Φ) is calculated by the energy difference between the electrostatic potential of the vacuum level (Evac) and the Fermi energy (EF) (Toroker et al., 2011).
Result and Discussion
Morphology Analysis
Crystallographic structures of prepared TiO2, MoS2, and MoS2/TiO2 composites are characterized by powder XRD (Figure 1). Prepared TiO2 is indexed to the anatase phase (JCPDS No. 12-2172). It is worth noting that ammonium ions from ammonium molybdate hydrolysis can easily inlayer into MoS2, which results in (002) peak obvious shifting to 9.65° (Liu et al., 2015; Zhang et al., 2017). Furthermore, no obvious MoS2 peaks occur on MoS2/TiO2, which may be due to the small loading amount and its high dispersion on the surface of TiO2 (Yuan et al., 2016).
Photocatalytic Activity of TiO2 and MoS2/TiO2 Composites
Figure 2 shows the photocatalytic H2 production from H2S of pristine TiO2 and MoS2/TiO2 composites. As shown in Figure 2A, TiO2 reveals a poor photocatalytic hydrogen evolution activity. Remarkably, all the MoS2/TiO2 composites exhibit a higher photocatalytic performance than the pristine TiO2. In particular, MoS2/TiO2-2 possesses the highest H2 evolution rate compared to TiO2, which is about 9.4 times higher than TiO2 (Figure 2B). Meanwhile the H2 evolution rate is close to the previous report (Zhang et al., 2020) using the different MoO2/MoS2 composites as the photocatalyst with the same reaction media (Supplementary Table 1). The stable growth trend of H2 evolution indicated that H2S decomposed on MoS2/TiO2 is steady in an H2S environment. Meanwhile, the variation of Ead also can reveal the decomposition stability of H2S. The Ead values for the decomposed fragments of H2S including HS–H, H–S–H, and H2-S on the TiO2 surface are −2.65, −1.72, and −0.44 eV, while that on the MoS2/TiO2 surface are −2.69, −1.77, and −0.51 eV, respectively (Figures 2C,D). The more negative Ead value suggests that the decomposition of H2S fragments on the MoS2/TiO2 surface are more stable, which can be responsible for the high and stable photocatalytic activity. According to the activity results, the following characterizations including the crystal structure, morphology, surface chemical state, and optoelectronic properties are investigated for MoS2/TiO2-2 with the optimal activity as well as pristine TiO2 for comparison.
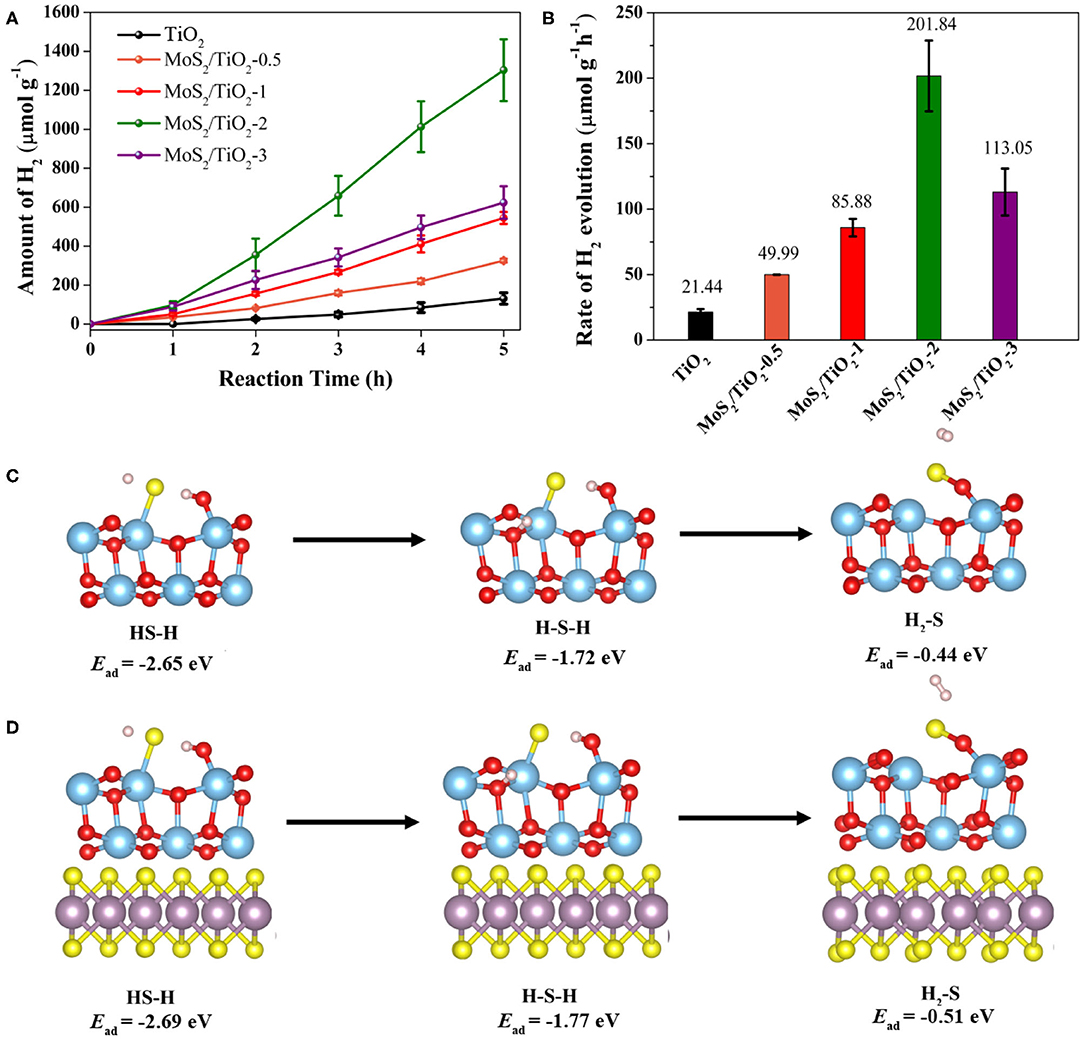
Figure 2. Photocatalytic H2 production on TiO2 and MoS2/TiO2 (A). Photocatalytic H2 evolution rate of TiO2 and MoS2/TiO2 (B). The variation of Ead for H2S fragments on the TiO2 surface (C) and MoS2/TiO2 surface (D).
Microstructure Analysis
To gain an intuitional understanding of the interface morphological structure between MoS2 and TiO2, the morphology of the TiO2 and MoS2/TiO2-2 nanostructures is thoroughly investigated by the TEM test. Meanwhile, EDS mapping of MoS2/TiO2-2 in Supplementary Figure 2 revealed four kinds of elements including Ti, O, Mo, and S. This result suggested that Mo and S have loaded on TiO2. The TEM image of MoS2/TiO2-2 photocatalyst presented in Figure 3c shows that the composite exhibits a morphology similar to ultrathin pure TiO2 nanosheet (Figure 3a). In addition, nanolayer materials are remarkably cladding with TiO2 nanosheet. HRTEM is applied to explore the morphology structure of prepared photocatalysts. As displayed in Figure 3b, the HRTEM image recorded from a single nanosheet clearly shows that the sample is made up of anatase TiO2 nanosheets with lattice fringes of 0.35 and 0.24 nm, which are attributed to the (101) plane and (001) plane of anatase TiO2, respectively (Zhou et al., 2013; Yang et al., 2018). Compared to ultrathin pure TiO2, the HRTEM image of MoS2/TiO2-2 (Figures 3c,d) indicates the extra crystal lattice fringes of 0.63 nm, which are attributed to the (002) plane of the nanolayer MoS2 (Liu et al., 2017).
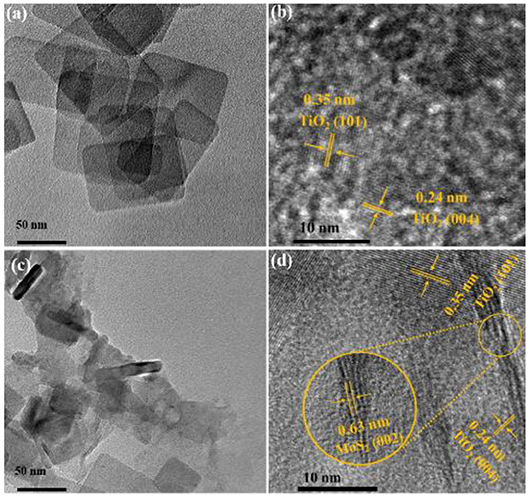
Figure 3. Transmission electron microscopy (TEM) and HRTEM spectra of TiO2 (a,b) and MoS2/TiO2-2 (c,d).
Electronic Properties and Chemical Valence
According to the above analysis, MoS2 loading has little effect on the morphological structure of TiO2. To explore the reason for the high photocatalytic activity, the interfacial interaction between TiO2 and MoS2 are further studied. As shown in Figure 4A, the band gap (Eg) of TiO2, MoS2, and MoS2/TiO2 is 2.77, 1.60, and 1.54 eV, respectively. The introduction of MoS2 largely reduced Eg by 1.23 eV compared to TiO2, and the narrowed Eg can broaden the light absorption into the visible light region and make the electron excitation much easier. Figure 4B illustrates that the valence band and conduction band of MoS2/TiO2 are interlaced consisted by TiO2 and MoS2, resulting in the interfacial electron transfer from O 2p of TiO2 to Mo 4d of MoS2. The obvious peaks of O 2p and Mo 4d orbits locate at the same energy level at the top of valence bands, and this orbital hybridization features indicate the strong interface interaction between TiO2 and MoS2.
The chemical valence state of Ti 2p, O 1s, Mo 3d, and S 2p are analyzed by X-ray photoelectron spectroscopy (XPS), which can examine the changes in valence states. The chemical valence state of Ti 2p is shown in Figure 5A. The two peaks at 464.4 and 458.8 eV are ascribed to Ti 2p1/2 and 2p3/2 with +4 valence from TiO2, which are in good agreement with the previous report (Yang, 2018). O 1s spectrum is given in Figure 5B; peak separation for TiO2 shows two kinds of oxygen with binding energies of 529.7 and 530.2 eV, which are assigned to the O lattice of TiO2 and oxygen coordination with Ti species (Gao et al., 2015; Li X. et al., 2019). In comparison, Ti 2p and O 1s of MoS2/TiO2-2 show an evident shift to higher energy, suggesting electron depletion or transfer at the composite interface, which is consistent with the DOS result. Figure 5C shows the Mo 3d valence state of MoS2/TiO2-2 composites. The typical peaks of 228.1 and 232.3 eV ensure the appearance Mo4+ of MoS2 (Shuxian et al., 1986; Woo et al., 1992). Meanwhile, peaks at 231.1 and 234.9 eV are consistent with the Mo4+ of MoO2 (Brox and Olefjord, 1988), which is due to the higher electronegativity of O (Bao et al., 2016). Furthermore, peaks at 161.2 and 162.5 eV demonstrate the existence of S2− in Figure 5D, suggesting the formation of MoS2 (Liu L. et al., 2013; Song et al., 2018). In addition, sulfur peak at 164.3 eV means some sulfur ions may be reduced in the reaction process (Zeng et al., 2019).
According to DOS analysis, it can be found that some electron transfer from TiO2 to MoS2 at the interface of MoS2/TiO2-2 composites, thus, causing a strong interfacial interaction. Furthermore, the electron transfer behavior at the interface is further explored by charge density difference maps as shown in Figure 6. A large amount of charge redistribution at MoS2 side may be induced by the strong interaction between TiO2 and MoS2. Furthermore, there are 0.10 e electrons flowing from TiO2 to MoS2, which is larger than the previous report (electron transfer quantity is 0.035 e) (Cao et al., 2014). A built-in electric field with the direction from TiO2 to MoS2 will form at the interface after the redistribution of the electron reaches an equilibrium state. The same phenomenon also can be found in DOS and XPS, which suggests a strong interfacial interaction between TiO2 and MoS2. Furthermore, the binging energy (Eb) of MoS2/TiO2 is −0.07 eV. The more negative Eb value means the more stable constructed structure and the stronger interface bonding at the composite interface (Bhowmik et al., 2007; Rybolt et al., 2007). As a result, MoS2/TiO2 can provide more electrons to participate in the reaction under light irradiation, and the electron transfer quantity of H2-S fragments on the surface (Supplementary Figure 3) also reveals the same result that the electron transfer quantity has increased by 0.01 e on the MoS2/TiO2 surface. At the same time, the interfacial photo-generated carrier transfer mechanism (Figure 7) illustrates the interfacial electron migration route under the illumination. According to the electrostatic potential in vacuum, the Φ value of TiO2 and MoS2/TiO2 is 4.52 and 5.23 eV, respectively (Supplementary Figure 4). Meanwhile, due to the more negative conduction band of TiO2 and the more positive valence band of MoS2, electrons and holes are consumed at the TiO2 conduction band and MoS2 valence band to participate in the redox reaction. Correspondingly, electrons and holes are accumulated at the MoS2 conduction band and TiO2 valence band, respectively. Because of the existence of the built-in electric field, the electrons at the TiO2 conduction band cannot transfer to the MoS2 conduction band, and holes at the MoS2 valence band cannot transfer to the TiO2 valence band. As a result, the translation direction of the electrons at the interface is from the MoS2 conduction band to the TiO2 valence band under light irradiation. Finally, the interfacial electron transfer mechanism prevents the electron from transferring from the conduction band of TiO2 to the valence band of TiO2 and results in a Z-scheme heterojunction. In other words, the interfacial electron transfer mechanism promotes the separation of electrons–holes and prolonged the lifetime of electrons, which facilitates the electrons accumulated at the conduction band of TiO2 to take part in the photocatalytic reactions. This plays an important role in enhancing the H2 production.
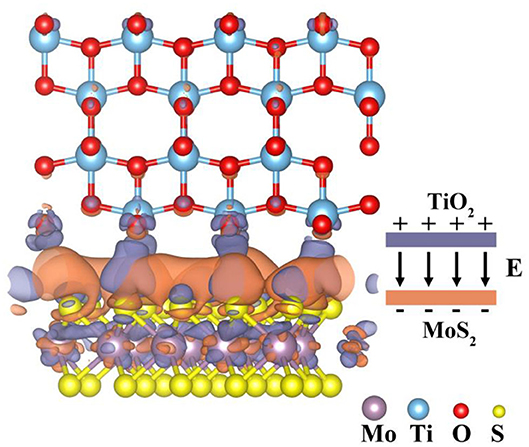
Figure 6. The charge density difference map (0.0002 electrons/Å3) of MoS2/TiO2. Orange and violet represent electron accumulation and depletion, respectively.
Optical and Photoelectric Properties
The UV-vis spectrum (Figure 8A) illustrates that the absorption edges of MoS2/TiO2 have obviously shifted to the visible light area compared with pure anatase TiO2 nanosheets. This phenomenon suggests that the MoS2/TiO2 band gap is smaller than TiO2 and brings the light absorbance broadening to the visible-light area, which is consistent with the band structure result that MoS2/TiO2 with the narrower Eg should have a better light response. Meanwhile, the broader light absorbance of MoS2/TiO2 can produce more photo-generated electrons and boost the photocatalytic reaction. The photoluminescence (PL) emission spectra (Figure 8B) are further used to investigate the behavior of photo-generated carriers. Remarkably, the lower emission peak intensity of MoS2/TiO2 proves a higher photo-generated carrier separation efficiency and provides more photo-generated electrons to participate in H2 evolution reaction as possible, which has the same result with interfacial electron transfer mechanism. Figure 8C presents the periodic on/off photocurrent response of TiO2 and MoS2/TiO2-2 under irradiation with a 300-W Xe lamp. TiO2 generates a relatively low photocurrent density with a value of 0.25 μA cm−2. The photocurrent density of MoS2/TiO2-2 significantly increases after MoS2 loading on the surface of TiO2 nanosheets. MoS2/TiO2-2 shows the highest photocurrent density of 0.79 μAcm−2, which is about three times higher than that of TiO2. This conclusively demonstrates that the separation efficiency of photo-generated charge carriers can be significantly enhanced by MoS2 existing on MoS2/TiO2 under light irradiation, which may be caused by the interfacial electron transfer mechanism. Furthermore, MoS2/TiO2-2 displays the lowest electrochemical impedance under light irradiation, giving a fast carrier separation performance (Figure 8D). As a result, the growth of the layered MoS2 on TiO2 cannot only promote the photo-generated carrier separation but also stimulates photo-generated electron transfer.
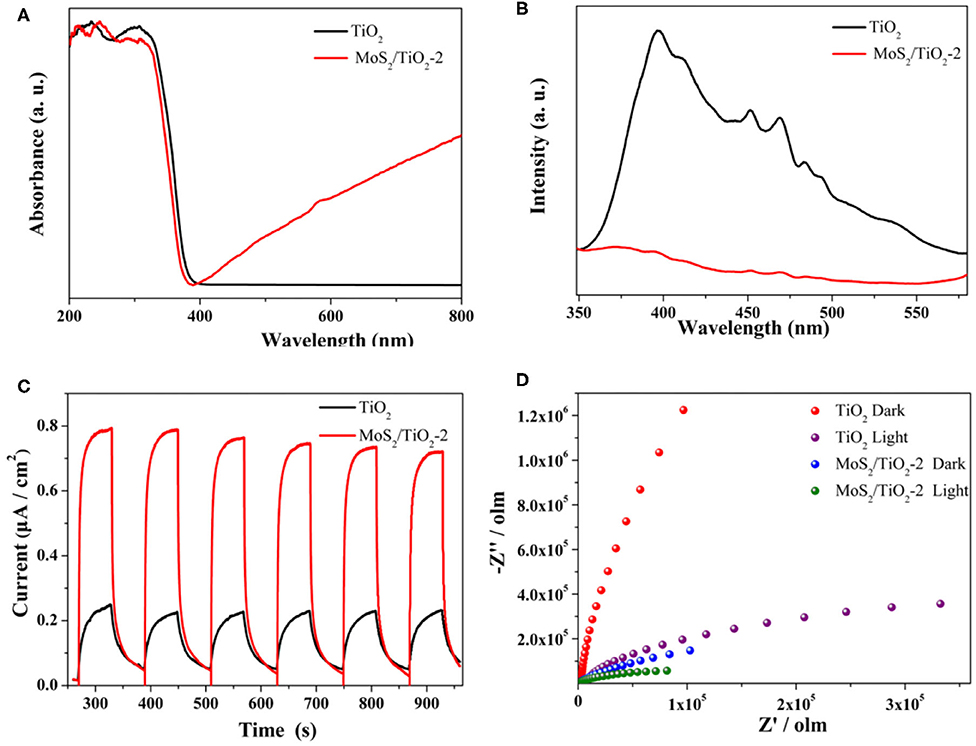
Figure 8. UV-vis absorption spectrum (A). Photoluminescence (PL) spectrum of as-prepared samples (B). Transient photocurrent responses of TiO2 and MoS2/TiO2-2 (C). Electrochemical impedance spectrum for TiO2 and MoS2/TiO2-2 with the light on or off (D).
According to the above analysis, the effects of the layered MoS2 loading on TiO2 {001} can be ascribed to the following several points. First, loading MoS2 can enhance the photocatalytic H2 evolution. MoS2/TiO2-2 shows about 9.4 times H2 evolution rate than TiO2. The stable growth trend of H2 evolution indicated that H2S decomposing on MoS2/TiO2 is steady in the H2S environment. Second, the interfacial electron transfer mechanism can prolong the life-time photo-generated electrons and increased the possibility of electrons to take part in H2 evolution reaction, which can result in high photocatalytic activity for MoS2/TiO2. The band structure shows that the narrower band gap of MoS2/TiO2 can broaden the light response into the visible light area. Meanwhile, the broader light response area means more photo-generated electron production, which is beneficial to the photocatalytic reaction. Furthermore, the lower PL intensity and higher photocurrent response of MoS2/TiO2 caused by the interfacial electron transfer mechanism reveals the high efficiency of photo-generated carrier separation, which can be the key factor for high H2 evolution.
Conclusion
This work reports the fabrication of 2D MoS2/TiO2 via loading of the layered MoS2 on TiO2 {001} for the photocatalytic conversion of H2 from H2S. The introduction of MoS2 greatly narrows the Eg and causes the admirable light absorbance in the visible light area, which is in good agreement with the UV-Vis tests. Moreover, obvious electron transfer is visualized at the interface of MoS2/TiO2, resulting in the built-in potential from TiO2 to MoS2, which is determined by DFT calculations and XPS test. Significantly, the interfacial electron transfer largely reduces the photo-generated carrier recombination and prolongs the lifetime of photo-generated electrons, which can be determined by the lower PL intensity and higher photocurrent response. Consequently, the photoactivity of H2 evolution is significantly improved from 21.44 to 201.84 μmol g−1 h−1. This study can provide new insights into designing efficient 2D photocatalysts to meet high H2 evolution from H2S by photocatalysis technology.
Data Availability Statement
The raw data supporting the conclusions of this article will be made available by the authors, without undue reservation.
Author Contributions
QC wrote the original draft. FW conceptualized, wrote, reviewed, and edited the manuscript. JX made the formal analysis. MD and SY were responsible for visualization and investigation. YZ wrote, reviewed, edited, and supervised the study. All authors contributed to the article and approved the submitted version.
Funding
This research was financially supported by the National Natural Science Foundation of China (U1862111), CAS Light of West China Program and Open Fund of State Key Laboratory of Oil, Gas Reservoir Geology and Exploitation (Southwest Petroleum University) (PLN 201802), and Open Fund of State Key Laboratory of Industrial Vent Gas Reuse (SKLIVGR-SWPU-2020-05).
Conflict of Interest
The authors declare that the research was conducted in the absence of any commercial or financial relationships that could be construed as a potential conflict of interest.
Supplementary Material
The Supplementary Material for this article can be found online at: https://www.frontiersin.org/articles/10.3389/fenvc.2020.591645/full#supplementary-material
References
Altomare, M., Nguyen, N. T., Hejazi, S., and Schmuki, P. (2018). A cocatalytic electron-transfer cascade site-selectively placed on TiO2 nanotubes yields enhanced photocatalytic H2 evolution. Adv. Funct. Mater. 28:1704259. doi: 10.1002/adfm.201704259
Bao, T., Yin, W., Zheng, X., Zhang, X., Yu, J., Dong, X., et al. (2016). One-pot synthesis of PEGylated plasmonic MoO3−x hollow nanospheres for photoacoustic imaging guided chemo-photothermal combinational therapy of cancer. Biomaterials 76, 11–24. doi: 10.1016/j.biomaterials.2015.10.048
Bhowmik, R., Katti, K. S., and Katti, D. (2007). Molecular dynamics simulation of hydroxyapatite–polyacrylic acid interfaces. Polymer 48, 664–674. doi: 10.1016/j.polymer.2006.11.015
Brox, B., and Olefjord, I. (1988). ESCA studies of MoO2 and MoO3. Surf. Interface Anal. 13, 3–6. doi: 10.1002/sia.740130103
Cai, Q., Wang, F., He, J., Dan, M., Cao, Y., Yu, S., et al. (2020). Oxygen defect boosted photocatalytic hydrogen evolution from hydrogen sulfide over active {001} facet in anatase TiO2. Appl. Surf. Sci. 517:146198. doi: 10.1016/j.apsusc.2020.146198
Cao, L., Wang, R., Wang, D., Xu, L., and Li, X. (2014). Enhanced visible light photocatalytic activity for the hybrid MoS2/anatase TiO2(001) nanocomposite: a first-principles study. Chem. Phys. Lett. 612, 285–288. doi: 10.1016/j.cplett.2014.08.048
Chang, K., Mei, Z., Wang, T., Kang, Q., Ouyang, S., and Ye, J. (2014). MoS2/graphene cocatalyst for efficient photocatalytic H2 evolution under visible light irradiation. ACS Nano 8, 7078–7087. doi: 10.1021/nn5019945
Chen, W., Yu, S., Zhong, Y., Fan, X., Wu, L., and Zhou, Y. (2018). Effect of electron transfer on the photocatalytic hydrogen evolution efficiency of faceted TiO2/CdSe QDs under visible light, N. J. Chem. 42, 4811–4817. doi: 10.1039/C8NJ00180D
Dan, M., Wei, S., Doronkin, D. E., Li, Y., Zhao, Z., Yu, S., et al. (2019a). Novel MnS/(InxCu1−x)2S3 composite for robust solar hydrogen sulphide splitting via the synergy of solid solution and heterojunction. Appl. Catal. B Environ. 243, 790–800. doi: 10.1016/j.apcatb.2018.11.016
Dan, M., Xiang, J., Wu, F., Yu, S., Cai, Q., Ye, L., et al. (2019b). Rich active-edge-site MoS2 anchored on reduction sites in metal sulfide heterostructure: toward robust visible light photocatalytic hydrogen sulphide splitting. Appl. Catal. B Environ. 256:117870. doi: 10.1016/j.apcatb.2019.117870
Dan, M., Zhang, Q., Yu, S., Prakash, A., Lin, Y., and Zhou, Y. (2017). Noble-metal-free MnS/In2S3 composite as highly efficient visible light driven photocatalyst for H2 production from H2S. Appl. Catal. B Environ. 217, 530–539. doi: 10.1016/j.apcatb.2017.06.019
Gao, C., Zhang, Z., Li, X., Chen, L., Wang, Y., He, Y., et al. (2015). Synergistic effects in three-dimensional SnO2/TiO2/CdS multi-heterojunction structure for highly efficient photoelectrochemical hydrogen production. Sol. Energ. Mat. Sol. C 141, 101–107. doi: 10.1016/j.solmat.2015.05.026
Ge, H., Xu, F., Cheng, B., Yu, J., and Ho, W. (2019). S-scheme heterojunction TiO2/CdS nanocomposite nanofiber as H2-production photocatalyst. ChemCatChem 11, 6301–6309. doi: 10.1002/cctc.201901486
Kibsgaard, J., Chen, Z., Reinecke, B. N., and Jaramillo, T. F. (2012). Engineering the surface structure of MoS2 to preferentially expose active edge sites for electrocatalysis. Nat. Mater. 11, 963–969. doi: 10.1038/nmat3439
Kresse, G., and Furthmüller, J. (1996). Efficient iterative schemes for ab initio total-energy calculations using a plane-wave basis set. Phys. Rev. B 54, 11169–11186. doi: 10.1103/PhysRevB.54.11169
Kresse, G., and Joubert, D. (1999). From ultrasoft pseudopotentials to the projector augmented-wave method. Phys. Rev. B 59 1758–1775. doi: 10.1103/PhysRevB.59.1758
Li, X., Lv, X., Li, N., Wu, J., Zheng, Y., and Tao, X. (2019). One-step hydrothermal synthesis of high-percentage 1T-phase MoS2 quantum dots for remarkably enhanced visible-light-driven photocatalytic H2 evolution. Appl. Catal. B Environ. 243, 76–85. doi: 10.1016/j.apcatb.2018.10.033
Li, Y., Hong, H., Xue, X., Zhang, Z., and Tian, H. (2019). MoS2 as cocatalyst for improving photocatalytic performance of Bi2MoO6, ChemistrySelect 4, 5222–5227. doi: 10.1002/slct.201900016
Li, Y., Wang, H., Xie, L., Liang, Y., Hong, G., and Dai, H. (2011). MoS2 nanoparticles grown on graphene: an advanced catalyst for the hydrogen evolution reaction. J. Am. Chem. Soc. 133, 7296–7299. doi: 10.1021/ja201269b
Lin, Y., Shi, H., Jiang, Z., Wang, G., Zhang, X., Zhu, H., et al. (2017). Enhanced optical absorption and photocatalytic H2 production activity of g-C3N4/TiO2 heterostructure by interfacial coupling: a DFT+U study. Int. J. Hydrogen Energ. 42, 9903–9913. doi: 10.1016/j.ijhydene.2017.02.172
Liu, B., Liu, X., Liu, J., Feng, C., Li, Z., Li, C., et al. (2018). Efficient charge separation between UiO-66 and ZnIn2S4 flowerlike 3D microspheres for photoelectronchemical properties. Appl. Catal. B Environ. 226, 234–241. doi: 10.1016/j.apcatb.2017.12.052
Liu, L., Gu, X., Cao, Y., Yao, X., Zhang, L., Tang, C., et al. (2013). Crystal-plane effects on the catalytic properties of Au/TiO2. ACS Catal. 3, 2768–2775. doi: 10.1021/cs400492w
Liu, Q., Li, X., He, Q., Khalil, A., Liu, D., Xiang, T., et al. (2015). Gram-scale aqueous synthesis of stable few-layered 1T-MoS2: applications for visible-light-driven photocatalytic hydrogen evolution. Small 11, 5556–5564. doi: 10.1002/smll.201501822
Liu, Q., Pu, Z., Asiri, A. M., Qusti, A., Al-youbi, A., and Sun, X. (2013). One-step solvothermal synthesis of MoS2/TiO2 nanocomposites with enhanced photocatalytic H2 production. J. Nanopart. Res. 15, 2057–2064. doi: 10.1007/s11051-013-2057-8
Liu, X., Liu, B., Li, L., Zhuge, Z., Chen, P., Li, C., et al. (2019). Cu2In2ZnS5/Gd2O2S:Tb for full solar spectrum photoreduction of Cr(VI) and CO2 from UV/vis to near-infrared light. Appl. Catal. B Environ. 249, 82–90. doi: 10.1016/j.apcatb.2019.02.061
Liu, X., Xing, Z., Zhang, Y., Li, Z., Wu, X., Tan, S., et al. (2017). Fabrication of 3D flower-like black N-TiO2−x@MoS2 for unprecedented-high visible-light-driven photocatalytic performance. Appl. Catal. B Environ. 201, 119–127. doi: 10.1016/j.apcatb.2016.08.031
Liu, X., Zhang, H., Yao, X., An, T., Liu, P., Wang, Y., et al. (2012). Visible light active pure rutile TiO2 photoanodes with 100% exposed pyramid-shaped (111) surfaces. Nano Res. 5, 762–769. doi: 10.1007/s12274-012-0259-5
Ren, Z., Liu, X., Zhuge, Z., Gong, Y., and Sun, C. (2020). MoSe2/ZnO/ZnSe hybrids for efficient Cr (VI) reduction under visible light irradiation. Chin. J. Catal. 41, 180–187. doi: 10.1016/S1872-2067(19)63484-4
Rybolt, T. R., Wells, C. E., Sisson, C. R., Black, C. B., and Ziegler, K. A. (2007). Evaluation of molecular mechanics calculated binding energies for isolated and monolayer organic molecules on graphite. J. Colloid Interface Sci. 314, 434–445. doi: 10.1016/j.jcis.2007.05.083
Sharma, A., Liu, N., Ma, Q., Zheng, H., Kawazoe, N., Chen, G., et al. (2020). PEG assisted P/Ag/Ag2O/Ag3PO4/TiO2 photocatalyst with enhanced elimination of emerging organic pollutants in salinity condition under solar light illumination. Chem. Eng. J. 385:123765. doi: 10.1016/j.cej.2019.123765
Shuxian, Z., Hall, W. K., Ertl, G., and Knözinger, H. (1986). X-ray photoemission study of oxygen and nitric oxide adsorption on MoS2. J. Catal. 100, 167–175. doi: 10.1016/0021-9517(86)90082-5
Song, S., Wang, J., Peng, T., Fu, W., and Zan, L. (2018). MoS2-MoO3−x hybrid cocatalyst for effectively enhanced H2 production photoactivity of AgIn5S8 nano-octahedrons. Appl. Catal. B Environ. 228, 39–46. doi: 10.1016/j.apcatb.2018.01.077
Sun, J., Duan, L., Wu, Q., and Yao, W. (2018). Synthesis of MoS2 quantum dots cocatalysts and their efficient photocatalytic performance for hydrogen evolution. Chem. Eng. J. 332, 449–455. doi: 10.1016/j.cej.2017.09.026
Tang, C., Xu, W., Chen, C., Li, Y., and Xu, L. (2017). Unraveling the orientation of MoS2 on TiO2 for photocatalytic water splitting. Adv. Mater. Interfaces 4, 1700361–1700367. doi: 10.1002/admi.201700361
Toroker, M. C., Kanan, D. K., Alidoust, N., Isseroff, L. Y., Liao, P., and Carter, E. A. (2011). First principles scheme to evaluate band edge positions in potential transition metal oxide photocatalysts and photoelectrodes. Phys. Chem. Chem. Phys. 13, 16644–16654. doi: 10.1039/c1cp22128k
Wang, Y., Liu, J., Ozaki, Y., Xu, Z., and Zhao, B. (2019). Effect of TiO2 on altering direction of interfacial charge transfer in a TiO2-Ag-MPY-FePc system by SERS. Angew. Chem. Int. Edit. 58, 8172–8176. doi: 10.1002/anie.201900589
Wang, Y., Sun, T., Yang, D., Liu, H., Zhang, Z., Yao, X., et al. (2012). Structure, reactivity, photoactivity and stability of Ti–O based materials: a theoretical comparison. Phys. Chem. Chem. Phys. 14, 2333–2338. doi: 10.1039/c2cp23143c
Woo, H. C., Nam, I., Lee, J. S., Chung, J. S., Lee, K. H., and Kim, Y. G. (1992). Room-temperature oxidation of K2CO3MoS2 catalysts and its effects on alcohol synthesis from CO and H2. J. Catal. 138, 525–535. doi: 10.1016/0021-9517(92)90304-Z
Xiang, J., Dan, M., Cai, Q., Yu, S., and Zhou, Y. (2019). Graphene oxide induced dual cocatalysts formation on manganese sulfide with enhanced photocatalytic hydrogen production from hydrogen sulfide. Appl. Surf. Sci. 494, 700–707. doi: 10.1016/j.apsusc.2019.07.219
Yang, Y. (2018). Comparative study of low-index {101}-TiO2, {001}-TiO2, {100}-TiO2 and high-index {201}-TiO2 on glyphosate adsorption and photo-degradation. Chem. Eng. J. 360:1247. doi: 10.1016/j.cej.2018.10.219
Yang, Y., Ye, K., Cao, D., Gao, P., Qiu, M., Liu, L., et al. (2018). Efficient charge separation from F− selective etching and doping of anatase-TiO2 {001} for enhanced photocatalytic hydrogen production. ACS Appl. Mater. Interfaces 10, 19633–19638. doi: 10.1021/acsami.8b02804
Yu, H., Yuan, R., Gao, D., Xu, Y., and Yu, J. (2019). Ethyl acetate-induced formation of amorphous MoSx nanoclusters for improved H2-evolution activity of TiO2 photocatalyst. Chem. Eng. J. 375:121934. doi: 10.1016/j.cej.2019.121934
Yu, S., Zhong, Y., Yu, B., Cai, S., Wu, L., and Zhou, Y. (2016). Graphene quantum dots to enhance the photocatalytic hydrogen evolution efficiency of anatase TiO2 with exposed {001} facet. Phys. Chem. Chem. Phys. 18, 20338–20344. doi: 10.1039/C6CP02561G
Yuan, Y., Ye, Z., Lu, H., Hu, B., Li, Y., Chen, D., et al. (2016). Constructing anatase TiO2 nanosheets with exposed (001) facets/layered MoS2 two-dimensional nanojunctions for enhanced solar hydrogen generation. ACS Catal. 6, 532–541. doi: 10.1021/acscatal.5b02036
Zeng, F., Li, N., Shen, Y., Zhou, X., Jin, Z., Yuan, N., et al. (2019). Improve the electrodeposition of sulfur and lithium sulfide in lithium-sulfur batteries with a comb-like ion-conductive organo-polysulfide polymer binder. Energy Storage Mater. 18, 190–198. doi: 10.1016/j.ensm.2018.09.018
Zhang, N., Ma, W., Wu, T., Wang, H., Han, D., and Niu, L. (2015). Edge-rich MoS2 naonosheets rooting into polyaniline nanofibers as effective catalyst for electrochemical hydrogen evolution. Electrochim. Acta 180, 155–163. doi: 10.1016/j.electacta.2015.08.108
Zhang, R., Wan, W., Li, D., Dong, F., and Zhou, Y. (2017). Three-dimensional MoS2/reduced graphene oxide aerogel as a macroscopic visible-light photocatalyst. Chin. J. Catal. 38, 313–320. doi: 10.1016/S1872-2067(16)62568-8
Zhang, Y., Guo, S., Xin, X., Song, Y., Lin, Y., Wang, B., et al. (2020). Plasmonic MoO2 as co-catalyst of MoS2 for enhanced photocatalytic hydrogen evolution. Appl. Surf. Sci. 504:144291. doi: 10.1016/j.apsusc.2019.144291
Zhao, M., Xu, H., Ouyang, S., Tong, H., Chen, H., Li, Y., et al. (2018). Fabricating a Au@TiO2 plasmonic system to elucidate alkali-induced enhancement of photocatalytic H2 evolution: surface potential shift or methanol oxidation acceleration? ACS Catal. 8, 4266–4277. doi: 10.1021/acscatal.8b00317
Keywords: photo-splitting of H2S, H2, MoS2/TiO2 {001}, electron transfer, DFT
Citation: Cai Q, Wang F, Xiang J, Dan M, Yu S and Zhou Y (2020) Layered MoS2 Grown on Anatase TiO2 {001} Promoting Interfacial Electron Transfer to Enhance Photocatalytic Evolution of H2 From H2S. Front. Environ. Chem. 1:591645. doi: 10.3389/fenvc.2020.591645
Received: 05 August 2020; Accepted: 08 September 2020;
Published: 23 November 2020.
Edited by:
Dengsong Zhang, Shanghai University, ChinaReviewed by:
Likun Pan, East China Normal University, ChinaYuechang Wei, China University of Petroleum, China
Copyright © 2020 Cai, Wang, Xiang, Dan, Yu and Zhou. This is an open-access article distributed under the terms of the Creative Commons Attribution License (CC BY). The use, distribution or reproduction in other forums is permitted, provided the original author(s) and the copyright owner(s) are credited and that the original publication in this journal is cited, in accordance with accepted academic practice. No use, distribution or reproduction is permitted which does not comply with these terms.
*Correspondence: Fang Wang, d2FuZ2ZuanVzdEAxNjMuY29t; Ying Zhou, eXpob3VAc3dwdS5lZHUuY24=