- 1School of Archaeology, University of Oxford, Oxford, United Kingdom
- 2School of Archaeology and Anthropology, The Australian National University, Canberra, ACT, Australia
- 3Santa Fe Institute, Santa Fe, NM, United States
- 4Department of Archaeology, Durham University, Durham, United Kingdom
- 5Department of Earth Sciences, Durham University, Durham, United Kingdom
- 6Department of Archaeology and Ancient History, Lund University, Lund, Sweden
- 7Department of Anthropology, Washington University in St Louis, St Louis, MO, United States
- 8Department of Anthropology, Trent University, Peterborough, ON, Canada
- 9Headland Archaeology, Hereford, United Kingdom
Stable isotope analysis of plant remains recovered from archaeological sites is becoming more routine. There remains a lack of consensus, however, on how to appropriately select archaeological plant remains for isotopic analysis, how to account for differences in preservation and the effect of potential contamination, and how to interpret the measured isotope values in terms of the conditions in which the plants grew. In this paper, we outline the main issues to be considered when planning and conducting an isotopic study of archaeobotanical remains. These include: (1) setting out the research question(s) that will be answerable using available analytical approaches, (2) considering the archaeological context from which plant remains derive, (3) determining appropriate sample size through consideration of estimate precision, (4) establishing the conditions in which plant remains have been preserved and potential effects on their isotope values, and (5) accounting for possible contamination during deposition. With these issues in mind, we propose some recommendations for researchers to consider when planning and conducting an isotopic study of archaeobotanical remains.
1 Introduction
Stable isotope values of plants reflect their growing conditions, which can be influenced by both natural environmental conditions and anthropogenic activities. In the last 10–15 years, there has been an increasing number of studies that have analyzed the stable carbon and nitrogen isotope values of charred crop remains recovered from archaeological sites to provide insights into past agricultural practices (e.g., Araus et al., 2014; Bogaard et al., 2013; Li et al., 2022; Riehl et al., 2008) and serve as an isotopic reference for reconstructing human and animal diets (e.g., Fraser et al., 2013b; Isaakidou et al., 2022; Knipper et al., 2020; Styring et al., 2018). Specifically, the stable carbon isotope (δ13C) values of crops can provide information about their water status (Ehleringer et al., 1993), which can help to identify watering practices such as irrigation in otherwise dry environments (e.g., Araus et al., 1997). Stable nitrogen isotope (δ15N) values of crops reflect the source and cycling of soil nitrogen, so can be affected by the addition of fertilizers such as animal manure, as well as environmental conditions such as waterlogging and aridity (e.g., Högberg, 1997).
When interpreting stable isotope values of archaeological crop remains in terms of past agricultural practices, it is important to consider the theory, method and practice underlying the disciplinary approach. One of the main principles underlying this approach is the extrapolation of experimental observations of modern crop experiments to the archaeobotanical record. This is a key theoretical pillar of experimental archaeology (Coles, 1979), but it is important to recognize that the modern observations are based on simulation experiments, not direct replication of the unknowable combination of soil, climate, plant physiology and societal factors for any one field or cultivation plot in the human past (Outram, 2008; Reynolds, 1999). As such, studies that outline the variability of modern crop isotope values in different soil and climatic conditions can be used as a quantitative guide and framework for interpretation, but caution must be used when directly quantitatively comparing the modern to the ancient isotopic values, a key theoretical consideration discussed in the wider experimental archaeology literature (Lin et al., 2018; Outram, 2008).
These theoretical considerations implicitly and explicitly underpin the types of research questions that are commonly set when analyzing stable isotope values in archaeological crop remains. There is a wide range of research establishing the modern baseline isotopic variability for different crop, climatic and soil amendment conditions across different parts of the world (e.g., Araus et al., 1997; Blanz et al., 2019; Dong et al., 2022; Fraser et al., 2011; Wallace et al., 2013) and an increasing number of taphonomic studies addressing issues of isotopic fractionation through preservation processes, such as carbonization, and how to identify, record and mitigate for these factors from experimental and archaeological crop remains (e.g., Nitsch et al., 2015; Stroud et al., 2023a; Teira-Brión et al., 2024; Varalli et al., 2023). Examples of common research questions, alongside some case studies that have addressed these using stable isotope analysis of archaeobotanical remains, are in Table 1.
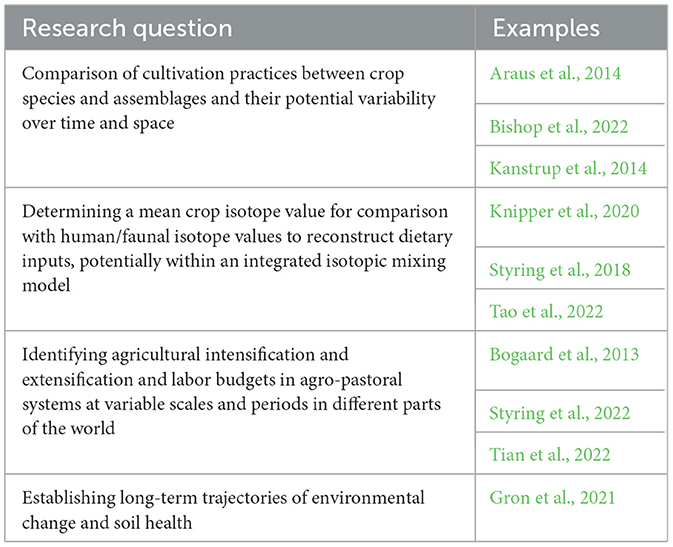
Table 1. Examples of common research questions and case studies that have employed stable isotope analysis of archaeobotanical remains.
Despite the increasing number of studies employing stable isotope analysis of archaeological crop remains, there remains a lack of consensus on how best to plan and conduct this type of research. Complications associated with the effect of charring, contamination, and post-depositional alteration on plant isotope values, as well as the interpretation of crop isotope values in terms of palaeoenvironment, agrarian practices, and geographic origin were discussed in Fiorentino et al. (2015). But as with any scientific application, the method reconfigures when research interests evolve. The last decade has seen considerable development in new research questions using stable isotope measurements of plants in an interdisciplinary domain and it is now timely to revisit and expand upon these methodological issues. Here, we set out a series of recommendations on how to plan and conduct stable isotopic investigation of archaeobotanical remains to encourage synergies among studies in different environmental settings and to inspire new research questions. In doing so, we reiterate some of the pressing points made in Vaiglova et al. (2022), which was a broader guide to best practice in archaeological isotope studies, but we expand on the issues that are particular to plant remains recovered from archaeological sites. In this paper we focus on stable isotope analysis of charred large-grained cereal grains (e.g., wheats and barley) and pulses using the C3 photosynthetic pathway because these have been the primary focus of research development so far, but we consider points relevant to C4 crops, including smaller grained cereals like millets and sorghum, where pertinent.
2 Challenges associated with stable isotope analysis of archaeological crop remains
There are several issues to be taken into consideration when planning and conducting isotopic analysis of archaeobotanical remains. These can influence the sampling protocol, the preparation method, the instrumental set-up and can ultimately determine whether the analyses are justified and interpretations are robust. We provide an overview of these issues as a background to suggesting steps that can be taken to ensure that studies try to address these as satisfactorily as possible.
2.1 Analytical difficulties of determining stable isotope values of plant material
Depending on the sensitivity of the isotope ratio mass spectrometer (IRMS) used, there is a minimum mass of C and N required for accurate and precise isotope measurements. Uncharred and charred cereal grains comprise relatively high %C and relatively low %N, giving rise to relatively high C:N atomic ratios (Table 2). This means that it can be difficult to simultaneously determine the δ13C and δ15N values of a cereal grain without either having too little N or overloading the IRMS with carbon dioxide. Even in elemental analyser (EA)-IRMS instruments where it is possible to dilute the carbon dioxide peak relative to the nitrogen gas peak, there is still a minimum quantity of plant material required to yield sufficient N for an accurate and precise δ15N value. As a result, single grain analysis of small-grained crops such as millets and pseudocereals is not yet practical due to their low mass.
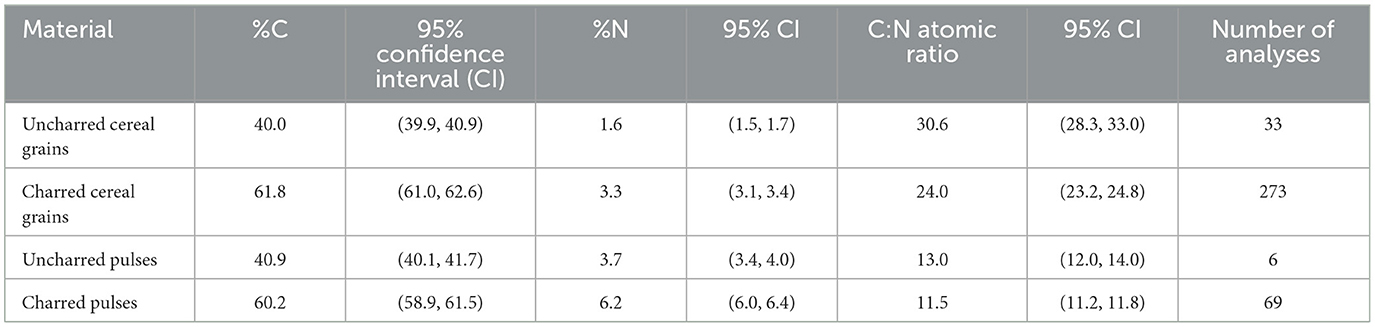
Table 2. C and N content and C:N atomic ratios of uncharred cereal grains (barley, bread wheat, einkorn, emmer, oat, rye, spelt) and pulses (lentil, pea) and cereal grains and pulses charred at between 215 and 300°C for between 4 and 24 h (from Stroud et al., 2023b: Dataset_1).
2.2 Intra-plant and intra-plot variability in crop stable isotope values
Most studies that have analyzed crop stable isotope values in modern experimental and farm plots homogenized multiple grains randomly sampled from across the growing plot to average out isotopic variation among plants (e.g., Blanz et al., 2019; Bogaard et al., 2007; Christensen et al., 2022; Fraser et al., 2011; Kanstrup et al., 2011; Styring et al., 2019; Treasure et al., 2016; Wallace et al., 2013). If single archaeological grains are to be analyzed individually, however, a good grasp of the isotopic variability among individual grains growing in the same conditions is needed in order to know whether differences in isotope values are representative of meaningful differences in growing conditions and not just a result of isotopic variability within a single plant/plot.
Relatively few studies have determined the isotopic variability among individual grains/seeds within a single cereal ear/pulse pod, but those that have been conducted have combined standard deviations below 0.4‰ (Table 3). The pooled standard deviation of δ15N values of 21 individual cereal ears is 0.36‰, while the pooled standard deviation of δ13C values of 11 individual cereal ears is 0.25‰. The pooled standard deviation of δ15N values of 8 pulse pods is 0.22‰. This variability is similar to the analytical uncertainty of stable isotope measurements, which is generally ~0.2‰−0.3‰, and therefore random sampling of grains/pulses from different parts of a cereal ear or pulse pod is unlikely to contribute significantly to inter-grain/pulse isotopic variability. However, more research is needed to expand the taxa investigated and to consider seasonal variations in flowering and/or grain filling conditions of a single plant/plot.
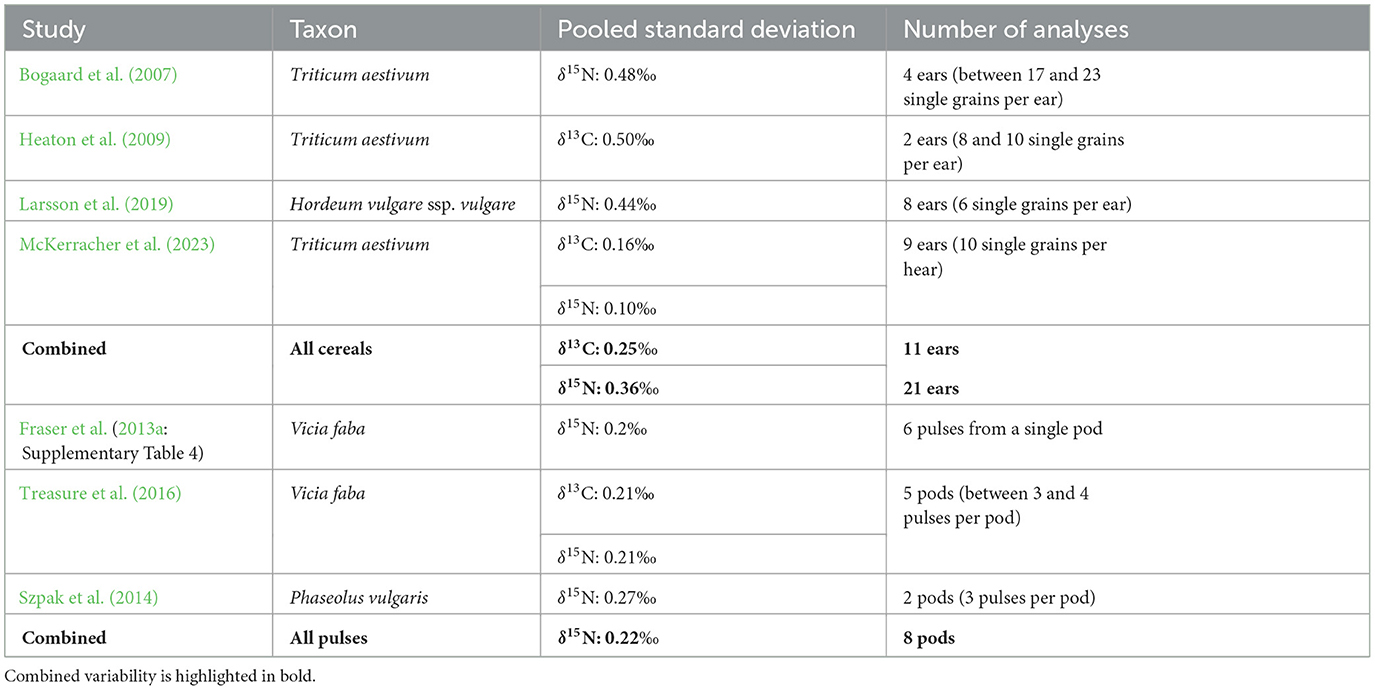
Table 3. Intra-ear/pod variability in δ13C and δ15N values of single grains/pulses (full dataset in Supplementary Table 1).
Even fewer studies have determined the isotopic variability among individual grains/pulses within a cultivation plot. Those that have been conducted show that the pooled standard deviation in δ15N from 7 plots is 0.76‰ and the pooled standard deviation in δ13C from 11 plots is 0.30‰ (Table 4). There are indications that there is greater isotopic variability among cereal grains grown in manured plots because of spatial variability in manure application (Larsson et al., 2019). For this reason, nitrogen isotopic variability within plots is likely constrained by the isotopic difference between the applied soil amendment and endogenous soil nitrogen. Similarly, the intra-plot variability in plant δ13C values is likely constrained by surface water availability and light intensity, which will vary more in plots with diverse topographies, and presence of or proximity to water bodies and/or overhanging vegetation. These values of intra-plot isotopic variability should therefore be treated as rough guidelines that will likely vary by context.
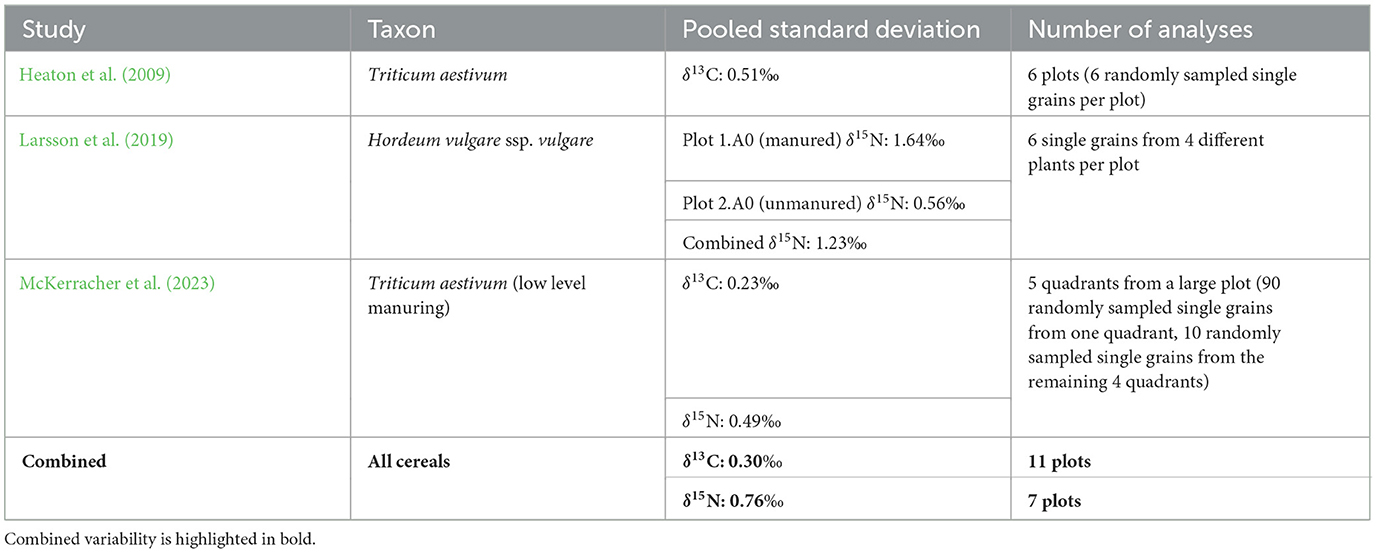
Table 4. Intra-plot variability in δ13C and δ15N values of single cereal grains (full dataset in Supplementary Table 2).
2.3 Environmental variability in crop stable isotope values
While the main focus of isotopic studies of archaeological crop remains has been to provide insights into past agricultural practices such as watering and soil amendment, there are environmental and physiological factors which also affect plant δ13C and δ15N values. Stable δ13C values of plants are influenced by the ratio of leaf intercellular (ci) to ambient (ca) carbon dioxide concentrations (Farquhar et al., 1982b). In C3 plants, this ratio is strongly affected by stomatal conductance and photosynthetic activity, which are in turn affected by water availability, soil salinity, and light intensity (Farquhar et al., 1989). When soil moisture levels decrease, stomatal conductance decreases, decreasing the ci and resulting in less negative δ13C values. The δ13C values of C3 plants are therefore negatively correlated with mean annual precipitation (e.g., Kohn, 2010) and water inputs in general—which could include irrigation—but other factors such as soil texture and organic matter content can affect soil water retention and also contribute to variability in crop δ13C values (Hudson, 1994). There is no straightforward relationship between water availability and the δ13C values of C4 plants (Cernusak et al., 2013), but a number of studies have observed that the δ13C values of C4 plants increase slightly with increased water availability (i.e., along a rainfall gradient; Schulze et al., 1996; Tieszen and Boutton, 1989), the opposite trend to that observed in C3 plants. High soil salinity reduces the ability of plants to take up water, thereby increasing the δ13C values of plant tissues of both halophytic and non-halophytic C3 species (Farquhar et al., 1982a), but decreasing the δ13C values of C4 plants slightly (Omoto et al., 2012).
Light intensity is an additional factor that has been found to influence plant δ13C values, with plants growing in lower light levels having lower rates of photosynthesis, leading to higher ci that results in lower δ13C values (Ehleringer et al., 1986, 1987). While most crops would be expected to have been cultivated in open plots and so would have been unaffected by low light intensity, if plots were situated in woodland clearings, there could be shading from surrounding vegetation for part of the day. This effect could be particularly apparent in tropical or semi-tropical environments with horticultural traditions and where rainforests can form particularly dense canopies. Furthermore, the grains of different plant taxa grown in the same environmental conditions have been observed to have different δ13C values, which is likely due to different timing of grain filling resulting in the δ13C values reflecting water availability at different times of the year (e.g., Araus et al., 1997; Wallace et al., 2013).
The δ15N values of plants are affected by the N isotopic composition of the soil in which they grow, which is influenced by the isotopic composition of any N inputs and by the loss of any N as a result of N cycling processes (e.g., Högberg, 1997). N can be added in the form of human/animal manure, bird guano, and/or composted organic matter, all of which tend to have relatively high δ15N values but can vary according to their source (see Szpak, 2014 for a compilation of the d15N values of different soil amendments). Soil properties may also moderate the isotopic effect of soil amendments, with an experimental study finding that similar inputs of biogas residues as fertilizer at two locations with different soil types resulted in very different δ15N values of cereal grains (Larsson et al., 2024). However, any process that favors the volatilisation of 15N-depleted N also drives an increase in the δ15N value of soil and plants (Handley et al., 1999). Such processes include aridity (e.g., Hartman and Danin, 2010), waterlogging (Finlay and Kendall, 2008), recent forest clearance by burning (Ehrmann et al., 2014), and high levels of organic N relative to plant demand (Craine et al., 2009). The presence and type of mycorrhizal associations also affect plant δ15N values (e.g., Craine et al., 2009) and plants growing in saline soils tend to have higher δ15N values than those growing in non-saline soils (Heaton, 1987). Each of these factors can result in isotopic variability that can confound interpretation of plant isotope values in terms of agricultural practice.
2.4 Preservation
The majority of plant remains in temperate contexts are preserved by charring (Zohary et al., 2012, p. 10). Charring results in changes to crop seed δ13C and δ15N values, the degree of which varies with the crop species and the temperature and duration of heating (Aguilera et al., 2008; Fraser et al., 2013a; Hart and Feranec, 2020; Hartman et al., 2020; Nitsch et al., 2015; Poole et al., 2002; Stroud et al., 2023a; Styring et al., 2019; Varalli et al., 2023). While it is not possible to determine the exact conditions in which crop remains were charred from morphological or chemical investigation, it has been shown that heating between 230 and 300°C results in well preserved cereal grains that are identifiable to species, although grains heated above 260°C tend to have a less dense matrix with voids that can become filled with soil (Stroud et al., 2023a). The average isotopic offset associated with heating cereal grains (barley, oat, pearl millet, rye, sorghum, wheat) between 230 and 300°C has been calculated to be ~0.2‰ for δ13C values and ~0.3‰ for δ15N values (Nitsch et al., 2015; Stroud et al., 2023a; Styring et al., 2019; Varalli et al., 2023).
While it has long been posited that the isotope values of desiccated plant remains are unreliable due to the effect of diagenesis (DeNiro and Hastorf, 1985), more recent studies have demonstrated that they can in fact yield reliable isotope values (Metcalfe and Mead, 2018; Szpak and Chiou, 2019). Szpak and Chiou (2019) propose plotting the C:N atomic ratios of archaeological plant remains against their δ15N values, and if a strong positive correlation is observed this could indicate the preferential loss of 14N during degradation. No investigation of the effect of waterlogging on the isotope values of uncharred plant remains has yet been carried out.
2.5 Contamination
Plant remains that have been buried in the soil are susceptible to contamination. The main potential contaminants are carbonates (with δ13C values of ~0‰; Schidlowski, 2001), nitrates (with δ15N values that have been found to vary between −2 and +8‰; Rock et al., 2011), and soil-derived humic acids (with δ13C and δ15N values similar to those of plants because this is what soil organic matter is derived from; Schnitzer and Khan, 1975). Vaiglova et al. (2014) used Fourier Transform-Infrared Spectroscopy (FT-IR) to detect these contaminants in charred plant material down to 10 % by dry weight of the charred plant material analyzed (down to 5 % for carbonate; Figure 1). Figure 2 shows the change in the δ13C and δ15N values of a powdered sample of charred peas (δ13C = −22.9‰, δ15N = 6.4‰) from an archaeological site contaminated with different proportions of carbonate (δ13C = 2.5‰), nitrate fertilizer (δ13C = 43.8‰, δ15N = −1.8‰), and a commercially purchased humic acid sodium salt (δ13C = −25.9‰, δ15N = 2.7‰) (data from Vaiglova et al., 2014: Table 4). With the exception of the nitrate fertilizer, 10 % by dry weight contamination of the archaeological pea sample resulted in changes in its δ13C or δ15N value of < 0.5‰.
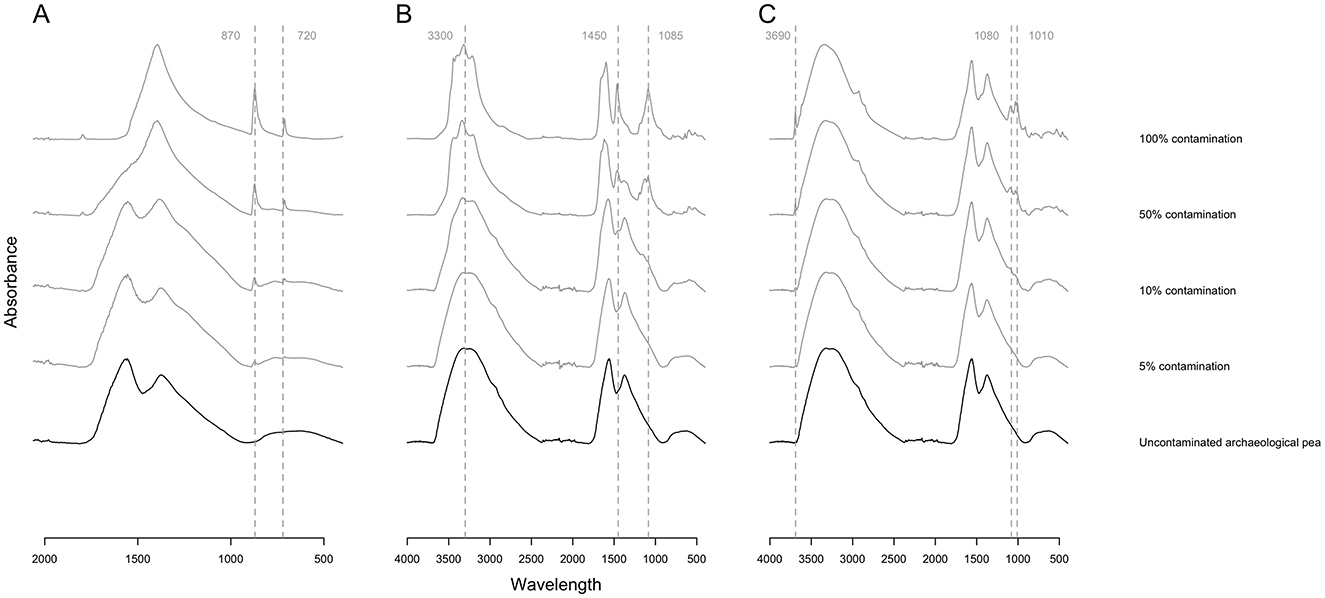
Figure 1. FTIR spectra of archaeological charred pea contaminated with varying percentages by dry mass of (A) carbonate, (B) nitrate fertilizer, and (C) humic acid sodium salt. Numbers next to dotted vertical lines refer to the wavelength of contaminant peaks identified by Vaiglova et al. (2014). Data from Vaiglova et al. (2014).
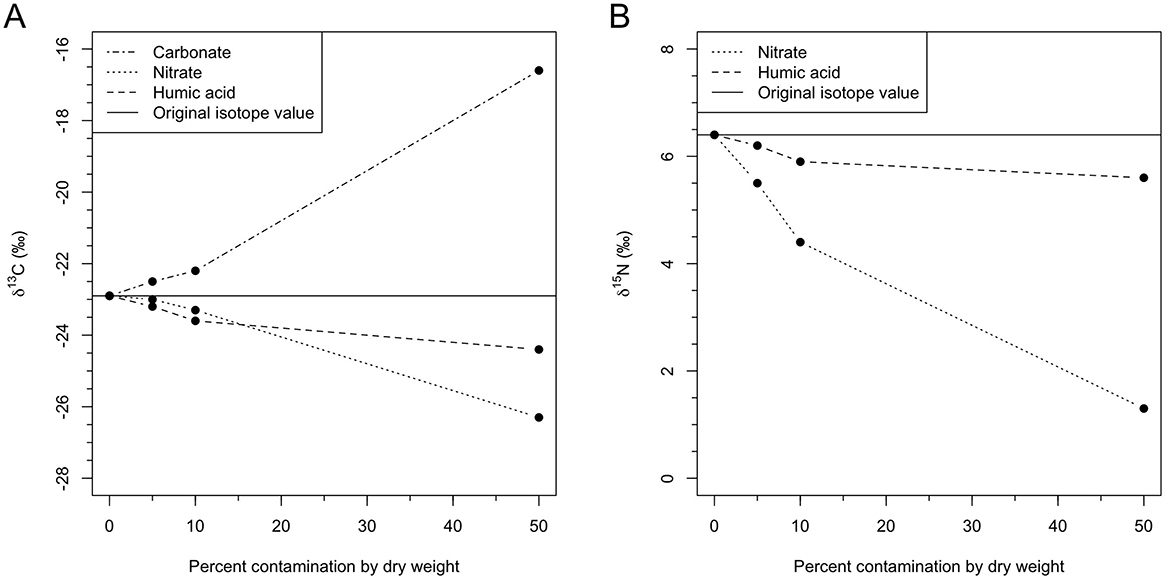
Figure 2. The effect of contaminating a powdered sample of charred archaeological peas (δ13C = −22.9‰, δ15N = 6.4‰; solid line in plots) with differing percentages by dry weight of carbonate (δ13C = 2.5‰), nitrate fertilizer (δ13C = −43.8‰, δ15N = −1.8‰), and a commercially purchased humic acid sodium salt (δ13C = −25.9‰, δ15N = 2.7‰) on (A) its δ13C values and (B) δ15N values (data from Vaiglova et al., 2014: Table 4).
3 Ways of addressing the challenges associated with stable isotope analysis of archaeological crop remains
3.1 Instrumental analysis of crop remains
Approximately 2 mg of charred cereal grain will yield ~1,200 μg C and 60 μg N (assuming 60% C and 3% N content). This is at the lower end of most IRMS limits for accurate and precise δ15N value measurements. It is therefore useful to analyse reference materials comprising plant material alongside samples to get a reasonable idea of the accuracy and precision of the isotopic measurements carried out. Wheat flour and sorghum flour IRMS reference materials are available from Elemental Microanalysis and millet flour (USGS90) and rice flour (USGS91) are available from the Reston Stable Isotope Laboratory or Arndt Schimmelmann, Indiana University. These reference materials have a lower N content (~1.5% N) than charred cereals, however, meaning that they are even more challenging to analyse by EA-IRMS and require 2–4 mg per analysis, which proves costly when performing a large number of analyses.
The development of in-house reference materials with isotopic and elemental compositions approximating those of the measured samples will likely be necessary for any researchers performing large quantities of isotopic analyses of plant remains; commercially-produced flours, similar to the USGS standards mentioned above, are frequently homogenous enough to produce consistent internal reference materials with stable isotope values measured over many years.
The carbon dioxide peak will always need to be diluted relative to the nitrogen gas peak (or diverted) in plant samples to avoid carryover to the nitrogen gas peak. If dilution is not possible or can only be applied to some limited extent, the δ13C and δ15N values could be measured on a small (< 1 mg) aliquot of the sample. This analysis will produce a reliable δ13C value as well as a reliable %N (but not δ15N) value. Based on this %N value, the analyst can then produce a second aliquot weighed specifically to produce precisely the appropriate amount of nitrogen gas required, while removing any of the produced CO2. The mass required for accurate and precise δ15N value measurements is higher for uncharred material (which tends to have lower %N) and lower for pulses that have higher %N (see Table 2). Given that preparation of samples (at a minimum involving crushing and weighing into tin capsules) invariably results in loss of material, it is advisable to weigh all potential samples before proceeding with any further preparative work to ensure that they are a minimum of 5–10 mg.
3.2 Determining optimal sample sizes using precision for planning
The method of “precision for planning” (also called Accuracy in Parameter Estimation, AIPE) is a powerful tool for determining optimal number of samples by specifying a desired statistical precision and calculating how many samples it would take to reach that precision. Tools such as the one provided on thenewstatistics.com > esci web > precision for planning (https://esci.thenewstatistics.com/esci-precision.html#tab-1) enable us to set the target precision on the x-axis and read the optimal sample size off the y-axis. Here, the margin of error (MoE)—which is one half of a confidence interval around a mean (i.e., the distance between the mean and the lower or upper limits of its confidence interval)—is used to determine the target precision. MoE can be standardized by dividing by the standard deviation, which makes it possible to work with samples that come from populations with different variabilities.
Here, we use precision for planning instead of statistical power because of the strong concerns that have been raised by the statistical community over null hypothesis significance testing (e.g., Amrhein et al., 2019; Byrd, 2007; Cohen, 1990; Cumming, 2014; Fidler et al., 2004; Goodman, 2008; Greenland et al., 2016; Ioannidis, 2005). In analyzing plant stable isotope datasets, we recommend the use of estimation science (i.e., focusing on describing what the actual difference between groups is) over declaring whether or not a test statistic passes an arbitrary threshold such as p = 0.01 or p = 0.05. Cumming and Calin-Jagerman (2024) provide many useful discussions and tools for applying estimation and inferential statistics in research in place of null hypothesis significance testing.
A common rule of thumb is to choose a MoE that is half of the expected effect size (i.e., the difference between the groups of interest). Let's consider a hypothetical archaeological scenario to illustrate how this works. Suppose we have archaeobotanical remains from a European site dating to three occupation phases: Neolithic, Bronze Age and Iron Age. The aim of our study is to determine whether barley was grown in different soil conditions during the three periods. Based on the differences in cereal grain δ15N values between low, medium and high levels of manuring established by Bogaard et al. (2013), we set our expected effect size to 3.0‰. In other words, we predict that if there is a true difference in soil management conditions, the mean δ15N values would differ by 3.0‰ or more. This would make the target MoE 1.5‰ (half of 3.0‰). Using the pooled standard deviation in δ15N values of individual grains/pulses from single cultivation plots of 0.76‰ (Section 2.2; Table 4), the standardized MoE would be 1.5/0.76 = 2 in standard deviation units.
Figure 3 demonstrates how the online esci web > precision for planning tool can be used to calculate the optimal number of samples. Figure 3A shows that setting the MoE to 2 (by toggling the slider on the x-axis) results in four barley grains required per phase. However, this precision will be reached only 51% of the times, since 49% of the distribution curve (in orange) lies above MoE = 2.0‰. To determine sample size at 99% assurance, the corresponding checkbox can be clicked in the panel on the left-hand side. When that is done (Figure 3B), the distribution curve (in orange) shifts so that 99% of the area falls under MoE = 2.0 and the optimal sample size changes to 6. However, because archaeobotanical grains rarely come from the same cultivation plot (or even the same temporal unit within an occupation phase), it should be expected that the real standard deviation is larger, in which case more conservative estimates should be made (e.g., MoE = 1.5/1 = 1.5, yielding a minimum of nine grains per period at 99% assurance).
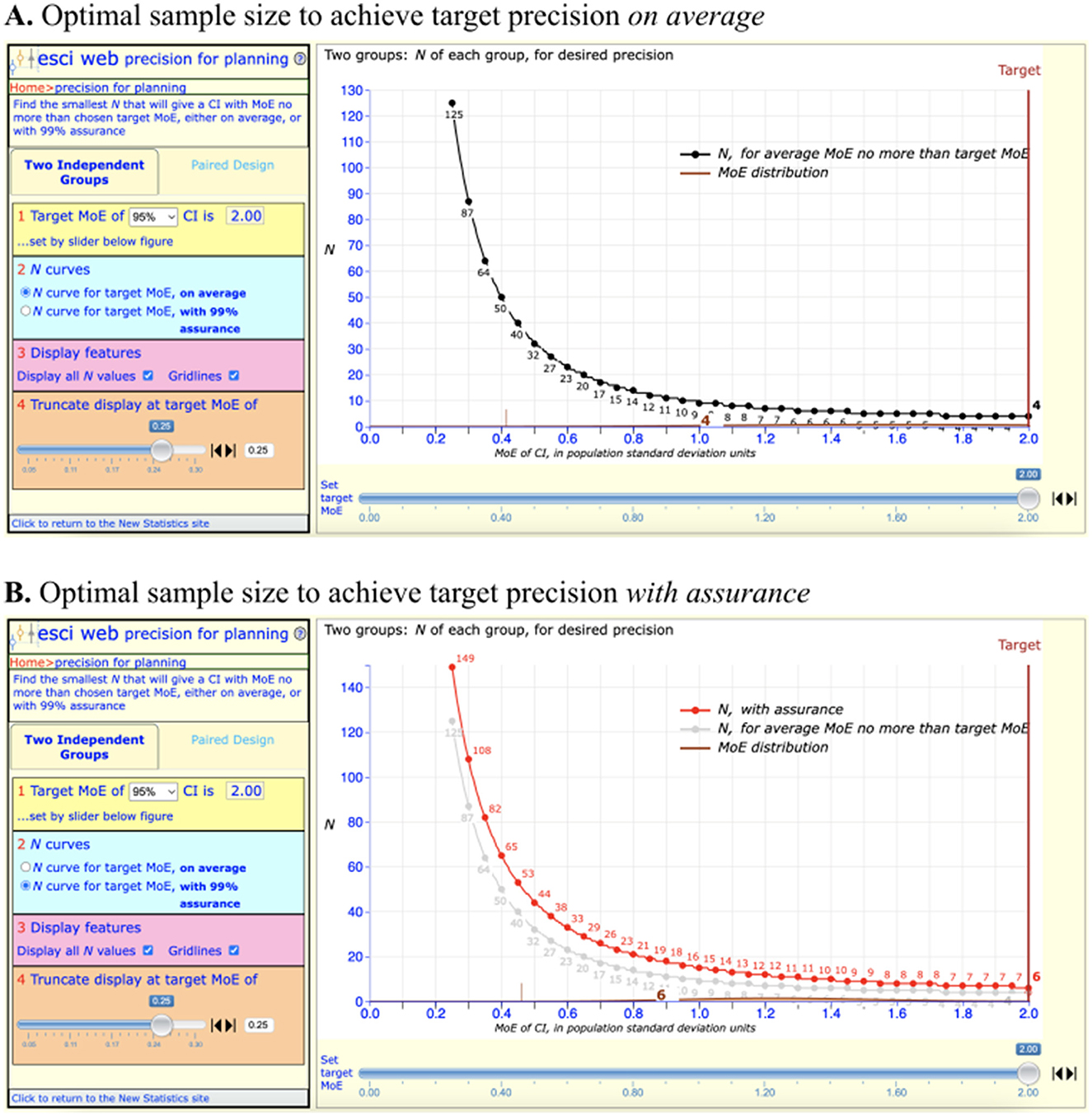
Figure 3. Demonstration of using “precision for planning” to determine optimal sample sizes. The example uses an online tool from thenewstatistics.com > esci web > precision for planning (https://esci.thenewstatistics.com/esci-precision.html#tab-1) (Cumming and Calin-Jagerman, 2024). (A) Using a target margin of error of 1.5‰ and a prediction that SD will be 0.76, the optimal number of samples for detecting a difference of 3.0‰ (on average) is 4 samples per group. (B) Using a target margin of error of 1.5‰ and a prediction that SD will be 0.76, the optimal number of samples for detecting a difference of 3.0% (at 99% assurance) is 6 samples per group.
It is useful during project preparation and grant application to consider what sample sizes would be required to detect realistic effect sizes under a range of expected standard deviations. Table 5 shows sample sizes required for capturing an effect size of 1.0‰ (Figure 3A) and an effect size of 3.0‰ (Figure 3B) with expected standard deviations between 0.2 and 1.0‰. As expected, fewer samples are needed to identify larger effect sizes. For example, only seven samples per group are needed to detect a difference of 3.0‰ with 99% assurance when SD = 0.8‰, but 30 samples per group are needed to detect a difference of 1.0‰ with 99% assurance when SD = 0.8%. However, even though large target MoE can in theory be achieved with very small sample sizes, we need to be particularly careful about using very small n (such as < 4). In replication studies, when the standard deviation of the underlying population is not actually known (as is the case in plant stable isotope studies), the width of the confidence intervals can bounce around substantially, so distinct sets of four samples can produce very different results, and the outcomes need to be treated with caution.
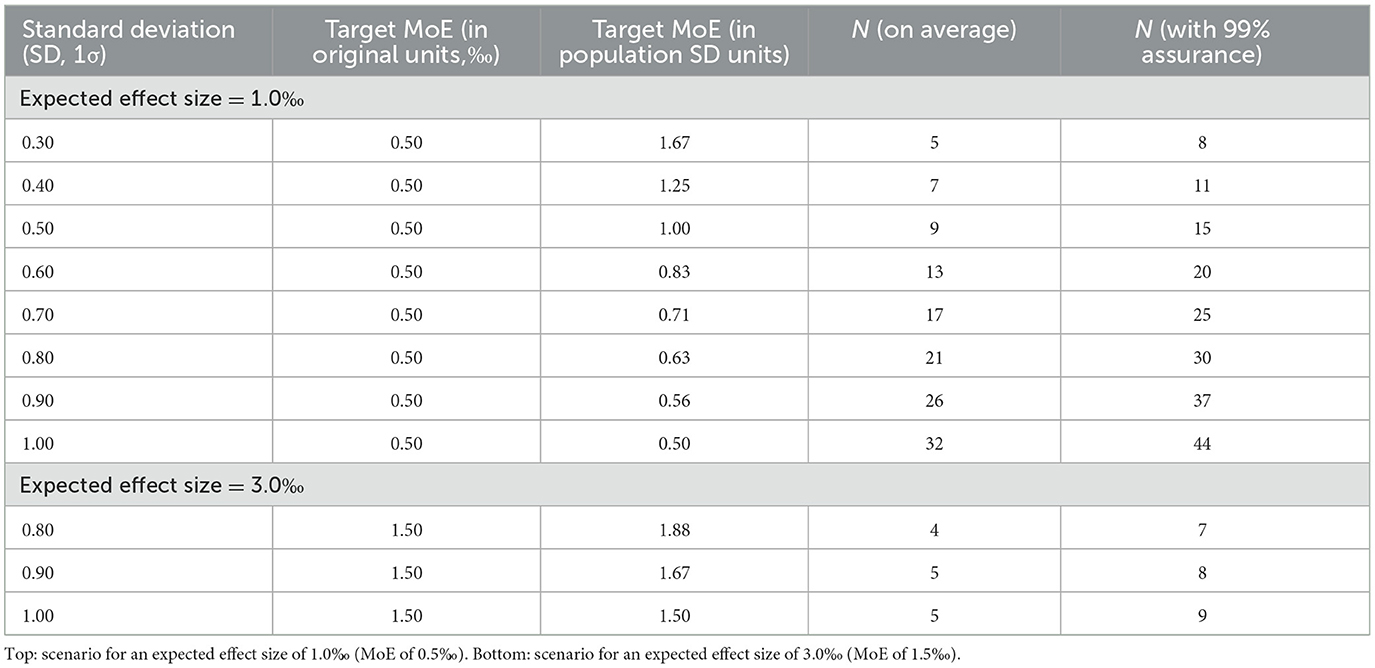
Table 5. Hypothetical combinations of expected standard deviations and effect sizes, and their effect on optimal sample sizes calculated using precision for planning.
When performing precision for planning, it is best to be conservative about the expected standard deviation, especially given the difficulties with understanding variability in archaeological datasets (Section 2.2). If it is underestimated, the results could risk missing the detection of meaningful differences. Additionally, it is important to remember that this method only works under the assumption that the samples in each group come from the same population. Thus, six samples each of wheat and barley may detect a 3.0‰ difference in δ15N values between a plot of wheat and a plot of barley, but not across several fields from distinct time periods. If chronological change is of interest, six samples should be obtained of each species from each period. If contexts are primary storage deposits (which are often the richest contexts in terms of crop remains), it may be that all items in that deposit came from the same population (i.e., same cultivation plot), but they could alternatively represent grain sourced and pooled from several fields. If primary contexts are unavailable, and the optimal sample sizes are unachievable, a 3.0‰ difference might go undetected. Further details on using precision for planning using different research designs can be found in Cumming and Calin-Jagerman (2024: Chapter 10).
Lastly, small sample sizes lead to large confidence intervals (i.e., low precision) around the estimates of interest. In some cases, this may result in a meaningful difference being obscured and undetectable. In other cases, large confidence intervals may make it impossible to identify the direction of an effect. For example, if a study aims to identify a linear correlation between δ15N and grain width and measures a Pearson's r of 0.4 using 10 grains, when a confidence interval (CI) is attached to the correlation coefficient, the result is r = 0.4, 95% CI (−0.3, 0.8). This means that the true relationship could be anywhere from weakly negative (−0.3) to strongly positive (0.8). If the isotopic difference between wheat (n = 3) and barley (n = 4) is 0.3‰, 95% CI (−1.0, 1.6), it means that the true difference could be anywhere from −1.0‰ to 1.6‰. When small sample sizes are unavoidable, it is imperative to restrict interpretations to descriptive statistics, without making unrealistic predictions about what the trends imply for the underlying population.
3.3 Accounting for environmental variability in crop isotope values
It is often not possible to distinguish between the effect(s) of environmental variability and agricultural practice on crop isotope values, but the potential of environmental factors to contribute to any differences or changes in crop isotope values should be considered and discussed for each case. For this reason, care must be taken when applying the “manuring bands” and water availability frameworks established by modern studies in particular regions (Bogaard et al., 2013; Wallace et al., 2013) to others with very different climate and soil types. Previous studies comparing crop isotope values from sites located in areas with differing annual rainfall levels have attempted to account for the effect of aridity on crop δ15N values and to normalize crop δ15N values between sites using linear regression in order to avoid incorrect interpretation of high crop δ15N values as solely due to manuring (Styring et al., 2016, 2017a, 2022). More experimental studies investigating the interplay of environmental factors and agricultural practices on crop isotope values will only improve these attempts at disentangling such confounding variables.
Another approach has been to estimate a “base-interval” of isotope values to be expected for unmanaged plants, by subtracting the mean offset between bone collagen and diet (~4‰ for δ15N; e.g., Steele and Daniel, 1978) from the isotope values of preserved wild herbivore bone collagen from the same archaeological sites and contexts as crop remains (e.g., Aguilera et al., 2018; Bogaard et al., 2013; Styring et al., 2017b). This relies, however, on the presence of wild herbivore bones and on the assumption that the plants consumed by wild herbivores were growing in similar environments to crops but that their isotope values were unaffected by human management. Domestic herbivore bone collagen isotope values are not used because they are more likely to reflect isotope values of plants that have been affected by human management.
3.4 Assessing the preservation of archaeological crop remains
There exists a corpus of images of cereal grains and pulse seeds experimentally heated for between 215 and 260°C for 4–24 h (Stroud et al., 2023b) that can be compared with the external morphology of archaeological crop remains to identify those that are likely to have been charred in similar conditions and thus minimize any isotopic offset due to charring. These are generally crop remains that can be identified to species and conform to preservation grades P2 or P3 (after Hubbard and al Azm, 1990). It is possible that even those crop remains whose external morphology suggests that they are well-preserved have voids in their cross-section indicating that they were heated to a higher temperature. These voids can also have infilling of soil, making contamination a concern. Cereal grains/pulses can therefore be cut in half using a scalpel to examine the internal morphology of the cross-section (also pictured in Stroud et al., 2023a,b) and discarded if large voids or soil contamination are present.
3.5 Addressing contamination of archaeological crop remains
Vaiglova et al. (2014) investigated the effectiveness of various pre-treatment methods in removing the most likely forms of contamination from soil: carbonates, nitrates and humic acids. They found that carbonate contamination was removed using a gentle acid wash (0.5 M HCl at 80°C for 30 min), nitrate was removed with three rinses in deionised water, and humic acid was removed with a gentle base-acid wash (0.1 M NaOH and 0.5 M HCl at 80°C, 60 min base treatment, 30 min acid treatment). This and various other studies have observed relatively small (< 1.0‰ for δ13C and < 1.5‰ for δ15N in 96% of cases; Brinkkemper et al., 2018) but variable changes in the isotope values of charred archaeological plant remains after varying combinations and concentrations of acid and base washes (Brinkkemper et al., 2018; Fraser et al., 2013a; Kanstrup et al., 2014; Vaiglova et al., 2014). It is very difficult to determine whether these changes in isotopic composition are due to removal of contaminants, or due to preferential removal of certain parts of the plant material that have different isotope values. Moreover, multiple washes of plant material results in its loss, with sample weight losses of 22 ± 9% with acid-only treatment, 37 ± 22% for base-acid treatment and 50 ± 17% for acid-base-acid treatment having been observed (Brinkkemper et al., 2018). In samples with low starting mass (such as single grains/seeds) pre-treatment could result in insufficient material remaining for isotopic analysis.
Given the uncertainties associated with the isotopic effect of pre-treatment methods on uncontaminated charred grains and seeds, and the relatively small changes that contaminants (and particularly humic acids) have on crop δ13C and δ15N values (Figure 2), three strategies have been proposed: (1) perform chemical pre-treatment on all samples, (2) screen a portion of archaeological crop samples from a range of context types and levels of preservation/visible dirtiness for potential contamination and only apply pre-treatment protocols if contamination is observed to be present, or (3) perform no chemical pre-treatment but undertake the physical removal of visible sediment, and report larger isotope value uncertainties to account for the unknown effect of contamination (cf. Brinkkemper et al., 2018). FT-IR spectroscopy is a good screening method as it uses minimal material, is very quick, and the spectra of contaminated crop material have been published (see Vaiglova et al., 2014; Figure 1). Researchers could also use their knowledge of the geology of an archaeological site to judge the likelihood of carbonate contamination, in particular. The only sites where carbonates have been detected in archaeological crop remains thus far are located on limestone geologies (e.g., Greece, northern Syria, south-east England, southern France; Alagich et al., 2018; Stroud, 2022; Styring et al., 2022; Vaiglova et al., 2020). Ultimately, more experimental studies on samples with known levels of contamination would be valuable to more conclusively determine how to effectively remove contamination in a wider range of situations.
4 Recommendations for planning and conducting stable isotope analysis of archaeological crop remains
The aim of this paper is to set out the challenges associated with crop stable isotope analysis and present potential strategies to mitigate against them. It is the intention that this format allows the reader to engage critically with the issues rather than follow a set protocol, and that this is the start rather than culmination of a discussion regarding best practice in stable isotope analysis of archaeological crop remains. In this regard, we propose the following recommendations for researchers planning to conduct an isotopic study of archaeological crop remains.
4.1 Set out the research question
The most successful isotopic studies are those that have a clear hypothesis to test, involving the comparison of two or more groups of interest or exploring the relationship between isotope values and an independent variable such as date. In archaeological studies, we are generally interested in how human actions have affected the isotope values of plants, to gain insights into the type and intensity of agricultural management. Given the multitude of environmental factors (e.g., soil type, climate, topography), aside from agricultural practices such as watering and fertilization, that can influence plant δ13C and δ15N values, comparisons of plant isotope values within sites are likely to be most robust because it can generally be assumed that these environmental factors are more consistent across the site. The isotope values of different crops recovered from the same archaeological contexts can be compared to investigate differences in management practices among crops; the isotope values of one or more crops can be determined through time to detect changing agricultural practices; and the isotope values of crops can be compared between/among discrete periods of occupation.
When comparing crop isotope values among sites, there is a greater chance that the isotope values will vary according to environmental differences rather than management practices per se. It is therefore useful to have a point of reference unlikely to be influenced by human management to act as an isotopic “base-interval” (cf. Vaiglova et al., 2022) for comparison with the crop isotope values (see Section 3.3).
4.2 Select appropriate samples
If plant remains derive from a secondary context (e.g., floor, midden), it cannot be assumed that multiple seeds come from the same growing condition and therefore isotopic analysis of single seeds will conserve the true variation among contexts. If plant remains derive from primary storage contexts (e.g., storage bins, pots), it might be the case that all items came from the same cultivation plot. In this case, isotopic analysis of a “bulk sample” of multiple grains will average out the inherent isotopic variability within the growing condition, providing a more precise estimate of the mean isotope value for that growing condition when budget and time are limited. Large primary deposits (e.g., large pits, granaries), however, could comprise grains sourced and pooled from multiple fields, or even from multiple settlements, meaning that analysis of single grains is actually more appropriate. Regardless, it is important that archaeological context is recorded for each sample (e.g., in Supplementary information) so that the isotope values of samples (whether individual grains or “bulk samples” of multiple grains) can be interpreted correctly in terms of expected variability and context. This information could include the total number of grains/pulses recovered from an archaeological context (but not necessarily isotopically analyzed), since this would indicate whether a deposit was large and therefore more likely to have derived from multiple sources.
Given the variability in individual cereal grain isotope values within a cultivation plot (Table 4), it is necessary that sufficient samples are analyzed in order to robustly identify any meaningful differences in isotope values among the groups of interest that cannot be accounted for by this inherent variability. Determining the number of samples necessary to determine these differences depends on the research question, the estimated effect size of a management regime of interest and the expected variability within a management regime. Although it is notoriously difficult to estimate effect sizes and variability, the availability of isotope data from modern experimental farming sites means that we can make a reasonable attempt at estimating the number of samples required, following the approach in Section 3.2. Care should be taken to ensure comparability between the groups of interest, so if, for example, the research question is whether there is a difference in manuring practice between the Neolithic and Bronze Age at a particular site, the crop species sampled should be consistent for both time periods.
4.3 Assess the suitability of samples for isotopic analysis
Well-preserved grains/seeds should be selected for isotopic analysis to minimize the isotopic offset resulting from charring and to minimize the risk of internal contamination with soil. Photographs of the dorsal and lateral views of intact grains provide a record of their external morphology that can be used for future geometric morphometric analysis (e.g., Bonhomme et al., 2017; Portillo et al., 2019; Wallace et al., 2019), and a photograph of the cross-section of each grain/pulse provides a record of the internal morphology which could be used to cross-reference to reference material charred under different conditions. It is possible that future research will enable better constraint of the isotopic changes associated with different preservation conditions and so having a visual record of the crop remains that have been analyzed (and ultimately destroyed) could be useful for refining interpretations.
Archaeobotanical remains could be screened for potential contamination and the spectra reported alongside isotopic data. FT-IR spectroscopy is a good method for this and we recommend that at least 10% of samples are screened in this way. This represents a balance between screening all samples (with the risk of sample loss that will preclude subsequent isotopic analysis) and screening enough to capture any potential contamination. If screening a sub-set of samples, it is advisable to screen samples from a range of context types and levels of preservation/visible dirtiness so that they are as representative as possible.
4.4 Prepare samples for isotopic analysis
There is a lack of consensus on whether to pre-treat all samples, regardless of contamination, pre-treat only those with evidence of contamination, or not to pre-treat at all. We leave it up to the reader to judge whether the potential level of contamination is likely to affect the isotope values more than pre-treatment to remove any potential contamination. Nevertheless, we recommend in all cases to scrape any visible dirt from the surface of grains/seeds using a scalpel under a low-power microscope or magnifying glass and to rinse grains/seeds in deionised water prior to any analyses to remove substantial amounts of soil and any surface debris. Samples should then be dried in a low temperature oven (~40°C) or lyophilised. Samples can then be crushed using an agate mortar and pestle and the resulting powder stored wrapped in aluminum foil inside microcentrifuge tubes to reduce the effect of static that can disperse the sample.
4.5 Conduct isotope measurements on archaeobotanical remains
Approximately 2 mg of charred cereal grain will yield enough C and N for accurate and precise δ13C and δ15N value measurements, although this depends on the EA-IRMS. The carbon dioxide peak will likely need to be diluted relative to the nitrogen gas peak if δ13C and δ15N values are to be determined simultaneously on the same sample. Best practice in terms of two-point normalization of isotopic data has been covered comprehensively in other publications (see Szpak et al., 2017). Wheat flour and sorghum flour IRMS reference materials from Elemental Microanalysis and millet flour (USGS90) and rice flour (USGS91) from Arndt Schimmelmann, Indiana University, are useful “check” standards as they are matrix-matched to plants, but other in-house reference materials could be used.
4.6 Report isotopic measurements of archaeobotanical remains
Again, best practice as to the reporting of isotope values has been discussed in other publications (e.g., Roberts et al., 2018; Vaiglova et al., 2022), but we recommend that the following information is published alongside the isotopic data when analyzing archaeobotanical remains: (1) archaeological context information for each sample, including the number of grains/pulses represented by each sample and the total number of that species recovered from the context, (2) photographs of external and internal morphology of each grain/seed, (3) FT-IR spectra where available, (4) detailed information of any pre-treatment of samples, and (5) elemental and isotopic data for all samples and reference materials included alongside these, including mass of plant sample analyzed.
5 Conclusion and future directions
In the last four decades or so, stable isotope analysis in archaeological research has moved from a technological innovation to an intellectual revolution. Many new research questions have been asked and addressed, and new interdisciplinary collaborations have been forged. Stable isotopic studies are situated naturally between numerous subfields of modern archaeology, including zooarchaeology, bioarchaeology, geoarchaeology, archaeobotany and genetic research, bringing great synergies to these related communities. The more recent application of stable isotope analysis to archaeobotanical macro-remains is one example of such collaboration—between archaeobotanists and isotope communities—that has enabled and continues to enable novel research questions to be addressed, in turn allowing us to develop new, compelling and relevant narratives.
While initial investigations have focused on large-grained cereals and pulses that originated in southwest Asia and spread to Europe, there has been considerable momentum in understanding ancient farming systems in regions beyond western Eurasia using these methods. One such example is the recent development in understanding the carbon and nitrogen isotopic compositions and the growing conditions of millet crops (e.g., Lightfoot et al., 2016; Reid et al., 2018; Sanborn et al., 2021; Styring et al., 2019). This new development has the potential to expand plant isotope research into regions historically less investigated, such as east, south and central Asia and sub-Saharan Africa, where more than twenty taxa of small-grained and ecologically hardy crops collectively known as millets were domesticated and sustained ancient populations. Moreover, stable sulfur isotope (δ34S) values of archaeological plant remains have the potential to track anaerobic conditions in waterlogged soil (Lamb et al., 2023; Nitsch et al., 2019) and even identify non-local plants whose δ34S values don't align with baseline δ34S variability. With such future developments in mind, this paper outlines the main issues to be considered when planning and conducting an isotopic study of archaeobotanical remains, in the hope of facilitating the proliferation of future research efforts in this field.
Author contributions
AS: Conceptualization, Formal analysis, Investigation, Visualization, Writing – original draft, Writing – review & editing. PV: Formal analysis, Investigation, Visualization, Writing – original draft, Writing – review & editing. AB: Writing – review & editing. MC: Writing – original draft, Writing – review & editing. DG: Writing – review & editing. ML: Writing – review & editing. XL: Writing – review & editing. ES: Conceptualization, Writing – review & editing. PS: Writing – review & editing. MW: Writing – review & editing.
Funding
The author(s) declare that no financial support was received for the research, authorship, and/or publication of this article.
Acknowledgments
We would like to thank two reviewers for their insightful comments that have improved the manuscript.
Conflict of interest
The authors declare that the research was conducted in the absence of any commercial or financial relationships that could be construed as a potential conflict of interest.
The author(s) declared that they were an editorial board member of Frontiers, at the time of submission. This had no impact on the peer review process and the final decision.
Publisher's note
All claims expressed in this article are solely those of the authors and do not necessarily represent those of their affiliated organizations, or those of the publisher, the editors and the reviewers. Any product that may be evaluated in this article, or claim that may be made by its manufacturer, is not guaranteed or endorsed by the publisher.
Supplementary material
The Supplementary Material for this article can be found online at: https://www.frontiersin.org/articles/10.3389/fearc.2024.1470375/full#supplementary-material
Supplementary Table 1 | Summary data on intra-ear/pod variability in δ13C and δ15N values of single cereal grains/pulses collated from Bogaard et al. (2007), Heaton et al. (2009), Larsson et al. (2019), McKerracher et al. (2023), Fraser et al. (2013a), Treasure et al. (2016) and Szpak et al. (2014). Data was used to calculate pooled variability shown in Table 3.
Supplementary Table 2 | Summary data on intra-plot variability in δ13C and δ15N values of single cereal grains collated from Heaton et al. (2009), Larsson et al. (2019) and McKerracher et al. (2023). Data was used to calculate pooled variability shown in Table 4.
References
Aguilera, M., Araus, J. L., Voltas, J., Rodríguez-Ariza, M. O., Molina, F., Rovira, N., et al. (2008). Stable carbon and nitrogen isotopes and quality traits of fossil cereal grains provide clues on sustainability at the beginnings of Mediterranean agriculture. Rapid Commun. Mass Spectrom. 22, 1653–1663. doi: 10.1002/rcm.3501
Aguilera, M., Zech-Matterne, V., Lepetz, S., and Balasse, M. (2018). Crop fertility conditions in North-Eastern Gaul during the la tène and roman periods: a combined stable isotope analysis of archaeobotanical and archaeozoological remains. Environ. Archaeol. 23, 323–337. doi: 10.1080/14614103.2017.1291563
Alagich, R., Gardeisen, A., Alonso, N., Rovira, N., and Bogaard, A. (2018). Using stable isotopes and functional weed ecology to explore social differences in early urban contexts: the case of Lattara in mediterranean France. J. Archaeol. Sci. 93, 135–149. doi: 10.1016/j.jas.2018.03.006
Amrhein, V., Greenland, S., and McShane, B. (2019). Scientists rise up against statistical significance. Nature 567, 305–307. doi: 10.1038/d41586-019-00857-9
Araus, J. L., Febrero, A., Buxó, R., Rodriguez-Ariza, M. O., Molina, F., Camalich, M. D., et al. (1997). Identification of ancient irrigation practices based on the carbon isotope discrimination of plant seeds: a case study from the South-East Iberian Peninsula. J. Archaeol. Sci. 24, 729–740. doi: 10.1006/jasc.1997.0154
Araus, J. L., Ferrio, J. P., Voltas, J., Aguilera, M., and Buxó, R. (2014). Agronomic conditions and crop evolution in ancient Near East agriculture. Nat. Commun. 5:3953. doi: 10.1038/ncomms4953
Bishop, R. R., Gröcke, D. R., Ralston, I., Clarke, D., Lee, D. H. J., Shepherd, A., et al. (2022). Scotland's first farmers: new insights into early farming practices in North-west Europe. Antiquity 96, 1087–1104. doi: 10.15184/aqy.2022.107
Blanz, M., Ascough, P., Mainland, I., Martin, P., Taggart, M. A., Dieterich, B., et al. (2019). Seaweed fertilisation impacts the chemical and isotopic composition of barley: implications for analyses of archaeological skeletal remains. J. Archaeol. Sci. 104, 34–44. doi: 10.1016/j.jas.2019.02.003
Bogaard, A., Fraser, R., Heaton, T. H. E., Wallace, M., Vaiglova, P., Charles, M., et al. (2013). Crop manuring and intensive land management by Europe's first farmers. PNAS 110, 12589–12594. doi: 10.1073/pnas.1305918110
Bogaard, A., Heaton, T. H. E., Poulton, P., and Merbach, I. (2007). The impact of manuring on nitrogen isotope ratios in cereals: archaeological implications for reconstruction of diet and crop management practices. J. Archaeol. Sci. 34, 335–343. doi: 10.1016/j.jas.2006.04.009
Bonhomme, V., Forster, E., Wallace, M., Stillman, E., Charles, M., Jones, G., et al. (2017). Identification of inter- and intra-species variation in cereal grains through geometric morphometric analysis, and its resilience under experimental charring. J. Archaeol. Sci. 86, 60–67. doi: 10.1016/j.jas.2017.09.010
Brinkkemper, O., Braadbaart, F., van Os, B., van Hoesel, A., van Brussel, A. A. N., and Fernandes, R. (2018). Effectiveness of different pre-treatments in recovering pre-burial isotopic ratios of charred plants. Rapid Commun. Mass Spectrom. 32, 251–261. doi: 10.1002/rcm.8033
Byrd, J. K. (2007). A call for statistical reform in EAQ. Educ. Adm. Q. 43, 381–391. doi: 10.1177/0013161X06297137
Cernusak, L. A., Ubierna, N., Winter, K., Holtum, J. A. M., Marshall, J. D., Farquhar, G. D., et al. (2013). Environmental and physiological determinants of carbon isotope discrimination in terrestrial plants. New Phytol. 200, 950–965. doi: 10.1111/nph.12423
Christensen, B. T., Jensen, J. L., Dong, Y., and Bogaard, A. (2022). Manure for millet: grain δ15N values as indicators of prehistoric cropping intensity of Panicum miliaceum and Setaria italica. J. Archaeol. Sci. 139:105554. doi: 10.1016/j.jas.2022.105554
Cohen, J. (1990). Things I have learned (so far). Am. Psychol. 45, 1304–1312. doi: 10.1037/10109-028
Craine, J. M., Elmore, A. J., Aidar, M. P. M., Bustamante, M., Dawson, T. E., Hobbie, E. A., et al. (2009). Global patterns of foliar nitrogen isotopes and their relationships with climate, mycorrhizal fungi, foliar nutrient concentrations, and nitrogen availability. New Phytol. 183, 980–992. doi: 10.1111/j.1469-8137.2009.02917.x
Cumming, G. (2014). The new statistics: Why and how. Psychol. Sci. 25, 7–29. doi: 10.1177/0956797613504966
Cumming, G., and Calin-Jagerman, R. (2024). Introduction to the New Statistics: Estimation, Open Science, and Beyond, 2nd Edn. New York, NY: Routledge. doi: 10.4324/9781032689470
DeNiro, M. J., and Hastorf, C. A. (1985). Alteration of 15N14N and 13C12C ratios of plant matter during the initial stages of diagenesis: studies utilizing archaeological specimens from Peru. Geochim. Cosmochim. Acta 49, 97–115. doi: 10.1016/0016-7037(85)90194-2
Dong, Y., Bi, X., Wu, R., Belfield, E. J., Harberd, N. P., Christensen, B. T., et al. (2022). The potential of stable carbon and nitrogen isotope analysis of foxtail and broomcorn millets for investigating ancient farming systems. Front. Plant Sci. 13:1018312. doi: 10.3389/fpls.2022.1018312
Ehleringer, J., Hall, A., and Farquhar, G. (1993). Stable Isotope and Plant Carbon-Water Relations. London: Academic Press.
Ehleringer, J. R., Field, C. B., Lin, Z. F., and Kuo, C. Y. (1986). Leaf carbon isotope and mineral composition in subtropical plants along an irradiance cline. Oecologia 70, 520–526. doi: 10.1007/BF00379898
Ehleringer, J. R., Lin, Z. F., Field, C. B., Sun, G. C., and Kuo, C. Y. (1987). Leaf carbon isotope ratios of plants from a subtropical monsoon forest. Oecologia 72, 109–114. doi: 10.1007/BF00385053
Ehrmann, O., Biester, H., Bogenrieder, A., and Rösch, M. (2014). Fifteen years of the Forchtenberg experiment—results and implications for the understanding of Neolithic land use. Veget. Hist. Archaeobot. 23, 5–18. doi: 10.1007/s00334-014-0452-4
Farquhar, G. D., Ball, M. C., von Caemmerer, S., and Roksandic, Z. (1982a). Effect of salinity and humidity on δ13C value of halophytes—evidence for diffusional isotope fractionation determined by the ratio of intercellular/atmospheric partial pressure of CO2 under different environmental conditions. Oecologia 52, 121–124. doi: 10.1007/BF00349020
Farquhar, G. D., Ehleringer, J. R., and Hubick, K. T. (1989). Carbon isotope discrimination and photosynthesis. Annu. Rev. Plant Physiol. Plant Mol. Biol. 40, 503–537. doi: 10.1146/annurev.pp.40.060189.002443
Farquhar, G. D., O'Leary, M. H., and Berry, J. A. (1982b). On the relationship between carbon isotope discrimination and the intercellular carbon dioxide concentration in leaves. Aust. J. Plant Physiol. 9, 121–137. doi: 10.1071/PP9820121
Fidler, F., Geoff, C., Mark, B., and Neil, T. (2004). Statistical reform in medicine, psychology and ecology. J. Socio-Econ. 33, 615–630. doi: 10.1016/j.socec.2004.09.035
Finlay, J. C., and Kendall, C. (2008). “Stable isotope tracing of temporal and spatial variability in organic matter sources to freshwater ecosystems,” in Stable Isotopes in Ecology and Environmental Science, eds. R. Michener, and K. Lajtha (Oxford: Blackwell Publishing Ltd.), 283–333. doi: 10.1002/9780470691854.ch10
Fiorentino, G., Ferrio, J. P., Bogaard, A., Araus, J. L., and Riehl, S. (2015). Stable isotopes in archaeobotanical research. Veget Hist. Archaeobot. 24, 215–227. doi: 10.1007/s00334-014-0492-9
Fraser, R. A., Bogaard, A., Charles, M., Styring, A. K., Wallace, M., Jones, G., et al. (2013a). Assessing natural variation and the effects of charring, burial and pre-treatment on the stable carbon and nitrogen isotope values of archaeobotanical cereals and pulses. J. Archaeol. Sci. 40, 4754–4766. doi: 10.1016/j.jas.2013.01.032
Fraser, R. A., Bogaard, A., Heaton, T., Charles, M., Jones, G., Christensen, B. T., et al. (2011). Manuring and stable nitrogen isotope ratios in cereals and pulses: towards a new archaeobotanical approach to the inference of land use and dietary practices. J. Archaeol. Sci. 38, 2790–2804. doi: 10.1016/j.jas.2011.06.024
Fraser, R. A., Bogaard, A., Schäfer, M., Arbogast, R., and Heaton, T. H. E. (2013b). Integrating botanical, faunal and human stable carbon and nitrogen isotope values to reconstruct land use and palaeodiet at LBK Vaihingen an der Enz, Baden-Württemberg. World Archaeol. 45, 492–517. doi: 10.1080/00438243.2013.820649
Goodman, S. (2008). A dirty dozen: Twelve P-Value misconceptions. Semin. Hematol. 45, 135–140. doi: 10.1053/j.seminhematol.2008.04.003
Greenland, S., Senn, S. J., Rothman, K. J., Carlin, J. B., Poole, C., Goodman, S. N., et al. (2016). Statistical tests, P values, confidence intervals, and power: a guide to misinterpretations. Eur. J. Epidemiol. 31, 337–350. doi: 10.1007/s10654-016-0149-3
Gron, K. J., Larsson, M., Gröcke, D. R., Andersen, N. H., Andreasen, M. H., Bech, J.-H., et al. (2021). Archaeological cereals as an isotope record of long-term soil health and anthropogenic amendment in southern Scandinavia. Quat. Sci. Rev. 253:106762. doi: 10.1016/j.quascirev.2020.106762
Handley, L. L., Austin, A. T., Stewart, G. R., Robinson, D., Scrimgeour, C. M., Raven, J. A., et al. (1999). The 15N natural abundance (δ15N) of ecosystem samples reflects measures of water availability. Funct. Plant Biol. 26, 185–199. doi: 10.1071/PP98146
Hart, J. P., and Feranec, R. S. (2020). Using Maize δ15N values to assess soil fertility in fifteenth- and sixteenth-century ad Iroquoian agricultural fields. PLoS ONE 15:e0230952. doi: 10.1371/journal.pone.0230952
Hartman, G., Brittingham, A., Gilboa, A., Hren, M., Maas, K., Pilver, J., et al. (2020). Post-charring diagenetic alteration of archaeological lentils by bacterial degradation. J. Archaeol. Sci. 117:105119. doi: 10.1016/j.jas.2020.105119
Hartman, G., and Danin, A. (2010). Isotopic values of plants in relation to water availability in the Eastern Mediterranean region. Oecologia 162, 837–852. doi: 10.1007/s00442-009-1514-7
Heaton, T. H., Jones, G., Halstead, P., and Tsipropoulos, T. (2009). Variations in the 13C/12C ratios of modern wheat grain, and implications for interpreting data from Bronze Age Assiros Toumba, Greece. J. Archaeol. Sci. 36, 2224–2233. doi: 10.1016/j.jas.2009.06.007
Heaton, T. H. E. (1987). The 15N/14N ratios of plants in South Africa and Namibia: relationship to climate and coastal/saline environments. Oecologia 74, 236–246. doi: 10.1007/BF00379365
Högberg, P. (1997). Tansley review No. 95. 15N natural abundance in soil-plant systems. New Phytol. 137, 179–203. doi: 10.1046/j.1469-8137.1997.00808.x
Hubbard, R. N. L. B., and al Azm, A. (1990). Quantifying preservation and distortion in carbonized seeds; and investigating the history of friké production. J. Archaeol. Sci. 17, 103–106. doi: 10.1016/0305-4403(90)90017-Y
Hudson, B. D. (1994). Soil organic matter and available water capacity. J. Soil Water Conserv. 49, 189–194.
Ioannidis, J. P. A. (2005). Why most published research findings are false. PLOS Med. 2:e124. doi: 10.1371/journal.pmed.0020124
Isaakidou, V., Halstead, P., Stroud, E., Sarpaki, A., Hatzaki, E., Nitsch, E., et al. (2022). Changing land use and political economy at neolithic and bronze age Knossos, Crete: stable carbon (δ13C) and nitrogen (δ15N) isotope analysis of charred crop grains and faunal bone collagen. Proc. Prehist. Soc. 88, 155–191. doi: 10.1017/ppr.2022.4
Kanstrup, M., Holst, M. K., Jensen, P. M., Thomsen, I. K., and Christensen, B. T. (2014). Searching for long-term trends in prehistoric manuring practice. δ15N analyses of charred cereal grains from the 4th to the 1st millennium BC. J. Archaeol. Sci. 51, 115–125. doi: 10.1016/j.jas.2013.04.018
Kanstrup, M., Thomsen, I. K., Andersen, A. J., Bogaard, A., and Christensen, B. T. (2011). Abundance of 13C and 15N in emmer, spelt and naked barley grown on differently manured soils: towards a method for identifying past manuring practice. Rapid Commun. Mass Spectrom. 25, 2879–2887. doi: 10.1002/rcm.5176
Knipper, C., Rihuete-Herrada, C., Voltas, J., Held, P., Lull, V., Micó, R., et al. (2020). Reconstructing Bronze Age diets and farming strategies at the early Bronze Age sites of La Bastida and Gatas (southeast Iberia) using stable isotope analysis. PLoS ONE 15:e0229398. doi: 10.1371/journal.pone.0229398
Kohn, M. J. (2010). Carbon isotope compositions of terrestrial C3 plants as indicators of (paleo)ecology and (paleo)climate. Proc. Nat. Acad. Sci. 107, 19691–19695. doi: 10.1073/pnas.1004933107
Lamb, A. L., Chenery, C. A., Madgwick, R., and Evans, J. A. (2023). Wet feet: developing sulfur isotope provenance methods to identify wetland inhabitants. R. Soc. Open Sci. 10:230391. doi: 10.1098/rsos.230391
Larsson, M., Bergman, J., and Lagerås, P. (2019). Manuring practices in the first millennium AD in southern Sweden inferred from isotopic analysis of crop remains. PLoS ONE 14:e0215578. doi: 10.1371/journal.pone.0215578
Larsson, M., Bergman, J., and Olsson, P. A. (2024). Soil, fertilizer and plant density: exploring the influence of environmental factors to stable nitrogen and carbon isotope composition in cereal grain. J. Archaeol. Sci. 163:105935. doi: 10.1016/j.jas.2024.105935
Li, H., Sun, Y., Yang, Y., Cui, Y., Ren, L., Li, H., et al. (2022). Water and soil management strategies and the introduction of wheat and barley to northern China: an isotopic analysis of cultivation on the Loess Plateau. Antiquity 96, 1478–1494. doi: 10.15184/aqy.2022.138
Lightfoot, E., Przelomska, N., Craven, M., O′Connell, T. C., He, L., Hunt, H. V., et al. (2016). Intraspecific carbon and nitrogen isotopic variability in foxtail millet (Setaria italica). Rapid Commun. Mass Spectrom. 30, 1475–1487. doi: 10.1002/rcm.7583
Lin, S. C., Rezek, Z., and Dibble, H. L. (2018). Experimental design and experimental inference in stone artifact archaeology. J Archaeol Method Theory 25, 663–688. doi: 10.1007/s10816-017-9351-1
McKerracher, M., Bogaard, A., Ramsey, C. B., Charles, M., Forster, E., Hamerow, H., et al. (2023). Digital archive for feeding anglo-saxon England (FeedSax): The Bioarchaeology of an Agricultural Revolution, 2017-2022. Oxford: University of Oxford.
Metcalfe, J. Z., and Mead, J. I. (2018). Do uncharred plants preserve original carbon and nitrogen isotope compositions? J. Archaeol. Method Theory. 26, 844–872. doi: 10.1007/s10816-018-9390-2
Nitsch, E. K., Charles, M., and Bogaard, A. (2015). Calculating a statistically robust δ13C and δ15N offset for charred cereal and pulse seeds. STAR 1, 1–8. doi: 10.1179/2054892315Y.0000000001
Nitsch, E. K., Lamb, A. L., Heaton, T. H. E., Vaiglova, P., Fraser, R., Hartman, G., et al. (2019). The preservation and interpretation of δ34S values in charred archaeobotanical remains. Archaeometry 61, 161–178. doi: 10.1111/arcm.12388
Omoto, E., Taniguchi, M., and Miyake, H. (2012). Adaptation responses in C4 photosynthesis of maize under salinity. J. Plant Physiol. 169, 469–477. doi: 10.1016/j.jplph.2011.11.009
Outram, A. K. (2008). Introduction to experimental archaeology. World Archaeol. 40, 1–6. doi: 10.1080/00438240801889456
Poole, I., Braadbaart, F., Boon, J. J., and van Bergen, P. F. (2002). Stable carbon isotope changes during artificial charring of propagules. Org. Geochem. 33, 1675–1681. doi: 10.1016/S0146-6380(02)00173-0
Portillo, M., Ball, T. B., Wallace, M., Murphy, C., Pérez-Díaz, S., Ruiz-Alonso, M., et al. (2019). Advances in morphometrics in archaeobotany. Environ. Archaeol. 25, 246–256. doi: 10.1080/14614103.2019.1569351
Reid, R. E. B., Lalk, E., Marshall, F., and Liu, X. (2018). Carbon and nitrogen isotope variability in the seeds of two African millet species: Pennisetum glaucum and Eleusine coracana. Rapid Commun. Mass Spectrom. 32, 1693–1702. doi: 10.1002/rcm.8217
Reynolds, P. J. (1999). “The nature of experiment in archaeology,” in Experiment and Design: Archaeological Studies in Honour of John Coles, ed. A. F. Harding (Oxford: Oxbow), 156–162.
Riehl, S., Bryson, R., and Pustovoytov, K. (2008). Changing growing conditions for crops during the Near Eastern Bronze Age (3000–1200 BC): the stable carbon isotope evidence. J. Archaeol. Sci. 35, 1011–1022. doi: 10.1016/j.jas.2007.07.003
Roberts, P., Fernandes, R., Craig, O. E., Larsen, T., Lucquin, A., Swift, J., et al. (2018). Calling all archaeologists: guidelines for terminology, methodology, data handling, and reporting when undertaking and reviewing stable isotope applications in archaeology. Rapid Commun. Mass Spectrom. 32, 361–372. doi: 10.1002/rcm.8044
Rock, L., Ellert, B. H., and Mayer, B. (2011). Tracing sources of soil nitrate using the dual isotopic composition of nitrate in 2 M KCl-extracts. Soil Biol. Biochem. 43, 2397–2405. doi: 10.1016/j.soilbio.2011.08.016
Sanborn, L. H., Reid, R. E. B., Bradley, A. S., and Liu, X. (2021). The effect of water availability on the carbon and nitrogen isotope composition of a C4 plant (pearl millet, Pennisetum glaucum). J. Archaeol. Sci. Rep. 38:103047. doi: 10.1016/j.jasrep.2021.103047
Schidlowski, M. (2001). Carbon isotopes as biogeochemical recorders of life over 3.8 Ga of Earth history: evolution of a concept. Precambrian Res. 106, 117–134. doi: 10.1016/S0301-9268(00)00128-5
Schulze, E.-D., Ellis, R., Schulze, W., Trimborn, P., and Ziegler, H. (1996). Diversity, metabolic types and δ13C carbon isotope ratios in the grass flora of Namibia in relation to growth form, precipitation and habitat conditions. Oecologia 106, 352–369. doi: 10.1007/BF00334563
Steele, K. W., and Daniel, R. M. (1978). Fractionation of nitrogen isotopes by animals: a further complication to the use of variations in the natural abundance of 15N for tracer studies. J. Agric. Sci. 90, 7–9. doi: 10.1017/S002185960004853X
Stroud, E., Charles, M., Bogaard, A., and Hamerow, H. (2023a). Turning up the heat: assessing the impact of charring regime on the morphology and stable isotopic values of cereal grains. J. Archaeol. Sci. 153:105754. doi: 10.1016/j.jas.2023.105754
Stroud, E., Charles, M., Bogaard, A., Nitsch, E., and Hamerow, H. (2023b). The experimental heating of rye, oat, spelt, wheat and barley between 215 and 300°C: the stable carbon and nitrogen isotope data and the photographic evidence of changes to the morphology of the grains. Data Brief 50:109544. doi: 10.1016/j.dib.2023.109544
Stroud, E. A. (2022). “Understanding early medieval crop and animal husbandry through isotopic analysis,” in New Perspectives on the Medieval ‘Agricultural Revolution': Crop, Stock and Furrow, eds. M. McKerracher, and H. Hamerow (Liverpool: Liverpool University Press), 41–60. doi: 10.2307/j.ctv333ktnp.10
Styring, A. K., Ater, M., Hmimsa, Y., Fraser, R., Miller, H., Neef, R., et al. (2016). Disentangling the effect of farming practice from aridity on crop stable isotope values: a present-day model from Morocco and its application to early farming sites in the eastern Mediterranean. Anthropocene Rev. 3, 2–22. doi: 10.1177/2053019616630762
Styring, A. K., Carmona, C. U., Isaakidou, V., Karathanou, A., Nicholls, G. K., Sarpaki, A., et al. (2022). Urban form and scale shaped the agroecology of early ‘cities' in northern Mesopotamia, the Aegean and Central Europe. J. Agrar. Change 22, 831–854. doi: 10.1111/joac.12497
Styring, A. K., Charles, M., Fantone, F., Hald, M. M., McMahon, A., Meadow, R. H., et al. (2017a). Isotope evidence for agricultural extensification reveals how the world's first cities were fed. Nat. Plants 3:17076. doi: 10.1038/nplants.2017.76
Styring, A. K., Diop, A. M., Bogaard, A., Champion, L., Fuller, D. Q., Gestrich, N., et al. (2019). Nitrogen isotope values of Pennisetum glaucum (pearl millet) grains: towards a reconstruction of past cultivation conditions in the Sahel, West Africa. Veget. Hist. Archaeobot. 28, 663–678. doi: 10.1007/s00334-019-00722-9
Styring, A. K., Knipper, C., Müller-Scheeßel, N., Grupe, G., and Bogaard, A. (2018). The proof is in the pudding: crop isotope analysis provides direct insights into agricultural production and consumption. Environ. Archaeol. 27, 61–72. doi: 10.1080/14614103.2018.1497832
Styring, A. K., Rösch, M., Stephan, E., Stika, H.-P., Fischer, E., Sillmann, M., et al. (2017b). Centralisation and long-term change in farming regimes: comparing agricultural practices in Neolithic and Iron Age south-west Germany. Proc. Prehist. Soc. 83, 357–381. doi: 10.1017/ppr.2017.3
Szpak, P. (2014). Complexities of nitrogen isotope biogeochemistry in plant-soil systems: implications for the study of ancient agricultural and animal management practices. Front. Plant Sci. 5. doi: 10.3389/fpls.2014.00288
Szpak, P., and Chiou, K. L. (2019). A comparison of nitrogen isotope compositions of charred and desiccated botanical remains from northern Peru. Veget. Hist. Archaeobot. 29, 527–538. doi: 10.1007/s00334-019-00761-2
Szpak, P., Longstaffe, F. J., Millaire, J.-F., and White, C. D. (2014). Large variation in nitrogen isotopic composition of a fertilized legume. J. Archaeol. Sci. 45, 72–79. doi: 10.1016/j.jas.2014.02.007
Szpak, P., Metcalfe, J. Z., and Macdonald, R. A. (2017). Best practices for calibrating and reporting stable isotope measurements in archaeology. J. Archaeol. Sci. Rep. 13, 609–616. doi: 10.1016/j.jasrep.2017.05.007
Tao, D., Xu, J., Wu, Q., Gu, W., Wei, Q., Zhou, Y., et al. (2022). Human diets, crop patterns, and settlement hierarchies in third millennium BC China: bioarchaeological perspectives in Zhengluo region. J. Archaeol. Sci. 145:105647. doi: 10.1016/j.jas.2022.105647
Teira-Brión, A., Kaal, J., and Charles, M. (2024). On the formation of charred millet aggregates in archaeological assemblages. Archaeometry. 1–17. doi: 10.1111/arcm.12983
Tian, D., Sun, Y., Ritchey, M. M., Xi, Ren, T., Ma, M., Wang, J. X., et al. (2022). Varying cultivation strategies in eastern Tianshan corresponded to growing pastoral lifeways between 1300 BCE and 300 CE. Front. Ecol. Evolut. 10:966366. doi: 10.3389/fevo.2022.966366
Tieszen, L. L., and Boutton, T. W. (1989). “Stable carbon isotopes in terrestrial ecosystem research,” in Stable Isotopes in Ecological Research, Ecological Studies, eds. P. W. Rundel, J. R. Ehleringer, and K. A. Nagy (New York, NY: Springer), 167–195. doi: 10.1007/978-1-4612-3498-2_11
Treasure, E. R., Church, M. J., and Gröcke, D. R. (2016). The influence of manuring on stable isotopes (δ13C and δ15N) in Celtic bean (Vicia faba L.): archaeobotanical and palaeodietary implications. Archaeol. Anthropol. Sci. 8, 555–562. doi: 10.1007/s12520-015-0243-6
Vaiglova, P., Gardeisen, A., Buckley, M., Cavanagh, W., Renard, J., Lee-Thorp, J., et al. (2020). Further insight into Neolithic agricultural management at Kouphovouno, southern Greece: expanding the isotopic approach. Archaeol. Anthropol. Sci. 12:43. doi: 10.1007/s12520-019-00960-y
Vaiglova, P., Lazar, N. A., Stroud, E. A., Loftus, E., and Makarewicz, C. A. (2022). Best practices for selecting samples, analyzing data, and publishing results in isotope archaeology. Quat. Int. 650, 86–100. doi: 10.1016/j.quaint.2022.02.027
Vaiglova, P., Snoeck, C., Nitsch, E., Bogaard, A., and Lee-Thorp, J. (2014). Impact of contamination and pre-treatment on stable carbon and nitrogen isotopic composition of charred plant remains. Rapid Commun. Mass Spectrom. 28, 2497–2510. doi: 10.1002/rcm.7044
Varalli, A., D'Agostini, F., Madella, M., Fiorentino, G., and Lancelotti, C. (2023). Charring effects on stable carbon and nitrogen isotope values on C4 plants: inferences for archaeological investigations. J. Archaeol. Sci. 156:105821. doi: 10.1016/j.jas.2023.105821
Wallace, M., Bonhomme, V., Russell, J., Stillman, E., George, T. S., Ramsay, L., et al. (2019). Searching for the origins of bere barley: a geometric morphometric approach to cereal landrace recognition in archaeology. J. Archaeol. Method Theory 26, 1125–1142. doi: 10.1007/s10816-018-9402-2
Wallace, M., Jones, G., Charles, M., Fraser, R., Halstead, P., Heaton, T. H. E., et al. (2013). Stable carbon isotope analysis as a direct means of inferring crop water status and water management practices. World Archaeol. 45, 388–409. doi: 10.1080/00438243.2013.821671
Keywords: carbon, nitrogen, δ13C, δ15N, agriculture, archaeobotany
Citation: Styring AK, Vaiglova P, Bogaard A, Church MJ, Gröcke DR, Larsson M, Liu X, Stroud E, Szpak P and Wallace MP (2024) Recommendations for stable isotope analysis of charred archaeological crop remains. Front. Environ. Archaeol. 3:1470375. doi: 10.3389/fearc.2024.1470375
Received: 25 July 2024; Accepted: 04 October 2024;
Published: 29 October 2024.
Edited by:
Yaowu Hu, Fudan University, ChinaReviewed by:
Andrew Somerville, Iowa State University, United StatesYimin Yang, University of Chinese Academy of Sciences, China
Copyright © 2024 Styring, Vaiglova, Bogaard, Church, Gröcke, Larsson, Liu, Stroud, Szpak and Wallace. This is an open-access article distributed under the terms of the Creative Commons Attribution License (CC BY). The use, distribution or reproduction in other forums is permitted, provided the original author(s) and the copyright owner(s) are credited and that the original publication in this journal is cited, in accordance with accepted academic practice. No use, distribution or reproduction is permitted which does not comply with these terms.
*Correspondence: Amy K. Styring, YW15LnN0eXJpbmcmI3gwMDA0MDthcmNoLm94LmFjLnVr