- 1Vienna Institute of Archaeological Science (VIAS), University of Vienna, Vienna, Austria
- 2Human Evolution and Archaeological Sciences (HEAS), University of Vienna, Vienna, Austria
- 3Austrian Archaeological Institute, Austrian Academy of Sciences, Vienna, Austria
- 4Department of Earth Sciences, Durham University, Durham, United Kingdom
- 5Agronomy and Agriculture Institute, University of the Highlands and Islands (UHI) Orkney, Orkney, United Kingdom
- 6Department of Archaeology, Durham University, Durham, United Kingdom
Introduction: Stable sulfur isotope ratios (δ34S) in bone collagen are often employed to study the consumption of marine and freshwater fish, wetland grazing, marine foraging patterns, and the possible geographic origins of food sources. However, a recent small-scale crop experiment showed that biofertilisation with seaweed can elevate δ34S in Celtic beans by ca. 10 ‰. Consumption of this food could erroneously suggest a marine diet and therefore has important implications for the reconstruction of past diets and dietary origins. However, limited research has so far been undertaken on cereals.
Methods: To address this issue, a large-scale field trial was undertaken on the Orkney Islands, whereby bere barley (a Scottish landrace, Hordeum vulgare L.) was biofertilised with seaweed at different dosages (25 t/ha, 50 t/ha), with a mineral NPK fertiliser, and left unfertilised as a control.
Results: The total barley biomass yield was higher and barley grains were enlarged following all fertilisation treatments compared to the control barley. Barley grain and straw from seaweed-fertilised crops had more elevated δ34S values by around 2–3 ‰ compared to unfertilised plants, while the NPK-fertilised grains and plants had δ34S values 1 ‰ lower.
Discussion: These results confirm previous hypotheses that seaweed fertilisation can elevate cereal δ34S values. The comparatively small δ34S difference between control and seaweed fertilised crops in this field trial is likely due to background elevated δ34S values in the soil (+12.7 ‰), which in turn may be due to long-term exposure to oceanic-influenced rain and sea spray and/or possible historical application of seaweed, or the underlying bedrock composition. The results of this study show that seaweed fertilisation can increase barley grain sizes and δ34S values, and thus should be considered when reconstructing land management and dietary practices in the archaeological record.
1 Introduction
The analysis of stable isotope ratios of archaeological plant material and skeletal remains has proven to be a valuable tool to study past plant husbandry practises, and to reconstruct past human and animal diets. The analysis of stable isotope ratios of carbon (δ13C) and nitrogen (δ15N) are frequently used to study past plant irrigation and fertilisation practises, and the extent of marine food and meat consumption by humans and animals (Bogaard et al., 2007; Makarewicz and Sealy, 2015; Schoeninger and Moore, 1992). Additionally, sulfur isotope ratio (δ34S) analyses of bone collagen enable the study of freshwater and marine fish consumption, wetland habitat use by grazing animals, marine animal foraging patterns, and the estimation of possible geographic origins of food sources, among other uses (e.g., Guiry et al., 2024, 2022; Lamb et al., 2023; Nehlich, 2015; Sayle et al., 2019; Szpak and Buckley, 2020; Vika, 2009).
These palaeodietary reconstructions make use of collagen δ34S, δ13C, and δ15N values to model dietary stable isotope ratios based on predictable offsets. However, plant carbon, nitrogen and sulfur isotope ratios can vary considerably, even within a single plant or leaf due to plant metabolism, and many environmental variables, such as climate and nutrient status, sea spray effects and oceanic influenced rain, amongst other factors (see Craine et al., 2015; Gröcke, 2020; Nehlich, 2015; Szpak, 2014; Tcherkez and Tea, 2013). Understanding this baseline variability is crucial for accurate dietary reconstructions, which can be achieved (at least in principle) through the stable isotope analysis of preserved plant material from the same site and time period, and/or by estimating the plant stable isotope ratio using modern reference data (if no suitable material is preserved). When plant remains are preserved in archaeological contexts, this usually occurs due to carbonisation, which may alter the original isotopic values in the plant (Beuning and Scott, 2002; Fraser et al., 2013; Nitsch et al., 2019). A greater understanding of agricultural-management practises that affect the isotopic composition of crop plants prior to carbonisation is required in order to produce more accurate palaeodietary reconstructions, especially in humans (Gröcke et al., 2021).
Factors affecting crop stable isotope ratios include manuring and other soil amendment strategies. For example, cereal δ15N values are generally elevated following fertilisation with terrestrial animal dung (Bogaard et al., 2007; Fraser et al., 2011; Kanstrup et al., 2011), seaweed, fish, and seabird guano (Blanz et al., 2019; Gröcke et al., 2021; Szpak et al., 2019; though other factors can also affect δ15N; Craine et al., 2015; Szpak, 2014). Where archaeological plant remains are preserved, commonly through charring, fertilisation-induced changes in the isotopic composition of crops can be assessed to study past fertilisation practises, including the investigation of when fertilisation first became an established part of agriculture (Bogaard and Outram, 2013). This also has important implications for the reconstruction of past diets, as consumption of biofertilised grain may lead to elevated consumer δ15N values that could be interpreted as the consumption of more meat and dairy. If the possibility of biofertilisation is not taken into account (and plant remains are not analysed directly), the amount of meat/dairy consumption is in danger of being overestimated (see Gröcke et al., 2021).
While the effects of fertilisation on δ15N are well-studied today, there has been considerably less work on crop sulfur isotope ratios. Archaeological grains are rarely analysed for δ34S, possibly in part because the factors affecting δ34S values in crops are not fully understood (e.g., Nitsch et al., 2019). Field studies have shown that synthetic and organic fertilisers may affect δ34S values of crops (Georgi et al., 2005; Gröcke et al., 2021; Mizota and Sasaki, 1996; Szpak et al., 2019), particularly when these are marine-derived, such as seabird guano, marine fish, and seaweed (Gröcke et al., 2021; Szpak et al., 2019). Historical accounts describe the frequent use of seaweed as a fertiliser particularly in coastal areas of the British Isles, in north-western France and Denmark, among others (e.g., Fenton, 1997; Hendrick, 1898; Neill, 1970; Russell, 1910; Sauvageau, 1920; Stephenson, 1968). Initial experimental work on Celtic beans showed an increase in stable sulfur isotope ratios (δ34S) due to fertilisation with seaweed, as well as when fertilising with marine fish (at an experimental site at a distance of ~10 km from the ocean; Gröcke et al., 2021). However, no such experimental research has been undertaken on δ34S in cereals.
δ34S values are often employed to study the consumption of marine and freshwater fish and to study the proximity of food sources to the coast (with higher collagen δ34S values generally indicating a marine influence; seawater δ34S ≈ +22 ‰ ± 1 ‰; Bottrell and Newton, 2006). Consumption of marine-fertilised (e.g., with seaweed, fish, shellfish) terrestrial crops may therefore lead to systematic overestimations of the consumption of marine foods if archaeobotanical remains are not analysed from the site. This also affects sites further inland due to transport of seaweed-fertilised crops and dried seaweed to be used as fertiliser. Thus, to enable more robust interpretations of plant δ34S values, further research is required.
In addition to the effects of seaweed-fertilisation on the crop's stable isotope ratios, the effects of seaweed fertilisation may include variation in the number, size and mass of individual grains (as previously indicated in the study on Celtic beans; Gröcke et al., 2021). The most common cereal macrofossils recovered from archaeological sites are grain, and simple measurements (length, width, depth, mass) have been used to infer agricultural innovation, crop development and productivity from the past 10,000 years across the world (cf. Bishop et al., 2022; Fuller et al., 2017; Larsson and Bergman, 2023). Therefore, the overall aim of this study is to determine the effects of seaweed-fertilisation on barley δ34S values and cereal grain sizes in an experimental field trial. The results of this work will help to interpret δ34S data from archaeological crops (e.g., to identify marine biofertilisation), as well as to refine modelling of human and animal diets and archaeological cereal grain weights.
2 Materials and methods
2.1 Field trial
In order to assess the effect of seaweed-fertilisation on barley δ34S values, a field trial was conducted. As the aim of this field trial was to gain information relevant to the reconstruction of past diets and farming practises, the field trial was designed to follow historically documented traditional farming practises as far as possible and practicable (rather than using e.g., present-day seaweed-extracts as fertiliser). The trial was undertaken with barley, as this was one of the earliest crops to be domesticated and has been of particular importance for both human and animal consumption until today (Fuller and Weisskopf, 2020), and historical evidence indicates it was frequently fertilised with seaweed (Fenton, 1997; Martin, 1716; Russell, 1910; Sauvageau, 1920). The traditional barley variety “bere barley” (Hordeum vulgare L.), a hulled lax-eared six-row landrace of barley (Martin et al., 2008), was chosen for this field trial rather than a modern barley variety, making greater similarity to barley varieties from archaeological sites more likely. A review of the historical literature on seaweed-fertilisation practises, and more detailed reasoning behind the field trial design choices are given in a previous publication on the same field trial (Blanz et al., 2019).
The field trial was conducted in 2017 on the Orkney islands (Scotland) at an agronomic experimental site ca. 100 m north of Orkney College and ca. 250 m south of the nearest coastline (58° 59′ N and 2° 57′ W; grid reference HY 456 114; Figure 1). This coastal site was exposed to oceanic-influenced rain and sea spray in windy weathers, which, with an δ34S value of ca. +20 ‰, can elevate δ34S (Richards et al., 2001; Zazzo et al., 2011). As seaweed-fertilisation is also expected to elevate δ34S values in the barley (cf. Gröcke et al., 2021; Szpak et al., 2019), this might obscure fertilisation effects on δ34S. However, proximity to the ocean was likely frequently the case when fertilising crops with seaweed (cf. Fenton, 1997), so that this coastal site location offers a realistic perspective on the extent of the effects of seaweed fertilisation on δ34S. The trial site was used for growing bere barley from 2002 and was likely mainly used for grazing through most of the twentieth century as part of Weyland Farm. The earliest mention of this farm dates back to 1595 (Peterkin, 1820), so that it is likely that the trial site has a long history of being used for grazing and also growing bere barley. The field consisted of an acidic clay loam soil that was previously cultivated and fertilised with a low-level NPK mineral fertiliser (50 kg N/ha). It had not been subjected to other fertilisation-based agronomic field trials before, ensuring soil homogeneity throughout the trial area. A randomised block design was chosen with five replicate plots per fertilisation treatment, each measuring 3 m × 3 m (9 m2) and spaced 1 m apart.
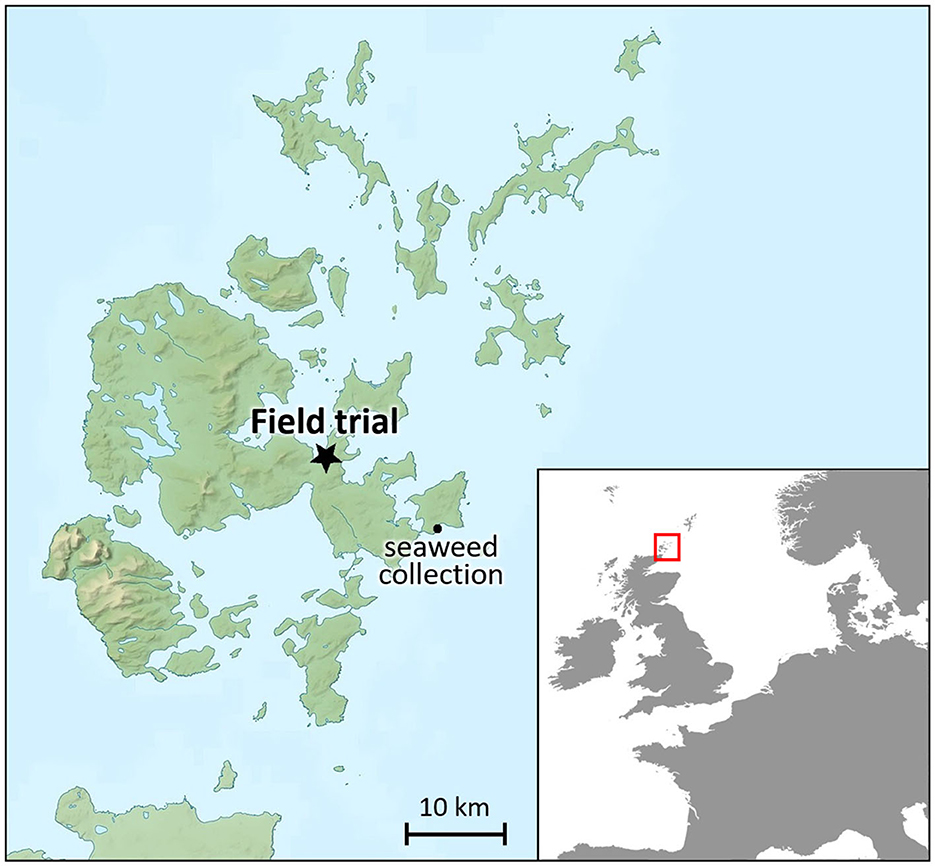
Figure 1. Location of field trial and seaweed collection on the Orkney Islands, Scotland, United Kingdom. Image contains Ordnance Survey data, licenced under UK Open Government Licence v3.0, and map data from Natural Earth II from www.NaturalEarthData.com (public domain).
Stranded seaweed of various species (including Laminaria spp., Fucus spp., and Ascophyllum nodosum; all with evidence for historical use as a fertiliser: Fenton, 1997; Hendrick, 1898; Neill, 1970; Russell, 1910; Sauvageau, 1920) was collected from the shore at Newark Bay, Mainland, Orkney (Grid reference: HY 567 041). As historical documents indicate that seaweed was frequently applied composted at the time of seeding (Fenton, 1997; Sauvageau, 1920), the seaweed in this trial was composted for 1.5 months in aerated plastic bags. After ploughing and power-harrowing, the seaweed was applied to the seaweed-treated plots at two rates: 25 t seaweed/ha and 50 t seaweed/ha (wet weight; Figure 2). A third set of plots was fertilised with a conventional mineral 14-14-21 NPK fertiliser (YaraMila MAINCROP 14-14-21; Yara UK Ltd, Belfast, UK) at a rate of 50 kg N/ha. A fourth set of plots (control plots) was left unfertilised. This gave a total of 20 plots: 5 unfertilised, 5 with 25 t seaweed/ha, 5 with 50 t seaweed/ha, and 5 NPK-fertilised. The fertilisers in each plot were mixed into the soil by power-harrowing. The following day (early May 2017), bere barley was sown at a rate of ~16 g/m2 with a thousand grain weight of 30.3 g, using a tractor drawn seeder (width 3 m). A Cambridge roller was then used to flatten the soil surface. In early June 2017, an herbicide mixture was applied to all plots. Additional information on the field trial is available in Blanz et al. (2019) and Brown et al. (2020).
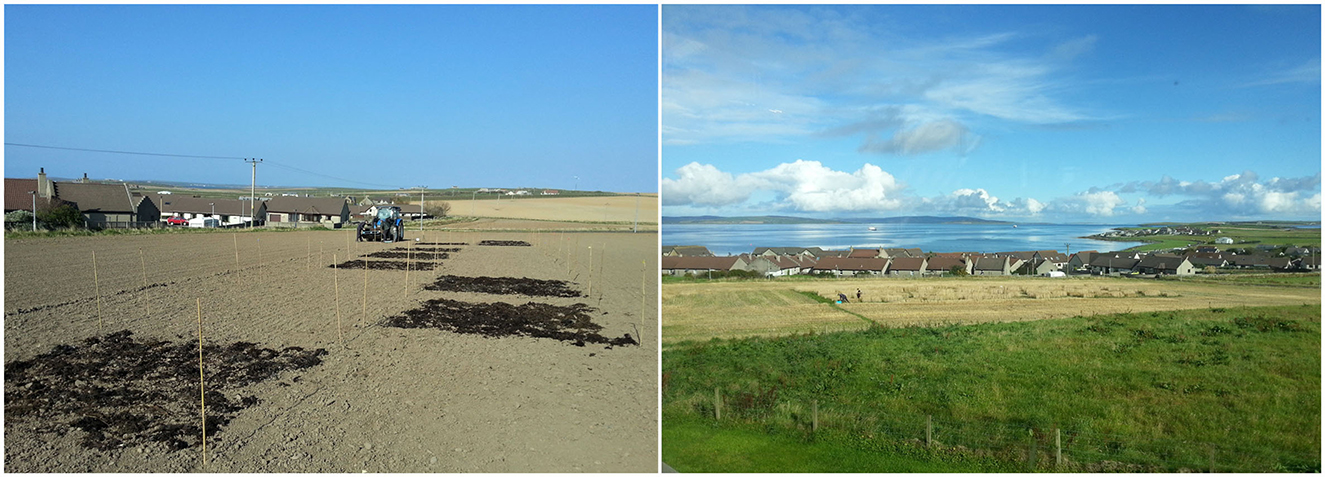
Figure 2. The seaweed-fertilisation trial site north of Orkney College. (Left) at time of sowing - early May 2017; (Right) at time of harvest - September 2017.
2.2 Sampling, yield, grain size measurements, and sulfur isotope analysis
In early September 2017 (i.e., after 4 months of growth), the bere barley was harvested from a 1 m × 1 m square at the centre of each 3 m × 3 m plot (to avoid edge effects, fertiliser run-off, and effects from tractor wheels). The barley was dried at 30 °C for 48 h (until constant weight) and weighed for yield evaluation (previously published in Brown et al., 2020). Thousand grain weights (TGWs) were estimated using 20 g grain subsamples using a MARVIN grain analyser (GTA Sensorik GmbH, Germany; i.e., 20 g grain was weighed, and then counted by the analyser). In archaeological contexts, there are frequently only few grains available, and whole grains need to be selected manually. Therefore, archaeological grains are usually weighed individually and measured for their size, but in much smaller numbers. To mirror these measurement conditions, this more detailed, small-scale approach was therefore also imitated in this study in parallel to the present-day agricultural yield evaluation. Fifteen bere barley plants were subsampled from each plot's central 1 m × 1 m square from which 10 individual grains per plot were randomly sub-sampled and archived. Five of these grains were randomly sub-sampled for this study, using random.org (Haahr, 2010). Each grain selected had a large proportion of the inner glume material (lemma and palea, ca. 90%) removed by scalpel, prior to biomass and isotopic measurements. This was undertaken as most hulled barley carbonised plant macrofossils found in the archaeological record in Atlantic Scotland have had the lemma and palea burnt off by the carbonisation process (except in rare circumstances such as the low temperature, reducing thermal environments in some parts of conflagrations; Church, 2002). The length, width and depth of each grain was then measured to the nearest 0.1 mm using the internal graticule of a Leica M80 stereomicroscope under × 7.5–60 magnification and the mass of each grain was measured to the nearest 0.001 g using a Mettler PM480 Delta Range balance. Two of the measured grains were then randomly sub-sampled for isotope analysis, ground individually using an agate mortar and pestle and weighed into tin capsules.
Additionally, 10 g of straw, including both stems and leaves, were taken from the 15 bere barley plants that were subsampled from each plot's central 1 m × 1 m square, homogenised using a spice and nut grinder (Model SG20U, Cuisinart Corp., Greenwich, USA), and sieved to 1 mm using a plastic mesh. The stems and leaves likely had different δ34S values (Tcherkez and Tea, 2013), but were homogenised to gain an overview—future studies should ideally analyse these separately.
For the analysis of the fertilisers, a pooled sample (120 g dry weight) of the composted seaweed as it was at the time of application in May was dried, ground and sieved as described for the straw samples. An aliquot of 1.5 g of the conventional NPK fertiliser was homogenised to a fine powder using a mortar and pestle. The top 0–25 cm of soil were sampled in three random spots in each plot using an augur, both before fertilisation, and after the trial, and pooled within each treatment group. The material was then sieved through a metal 1 mm mesh sieve.
Sulfur stable isotope ratio measurements were undertaken on the ground individual grains (2 grains per plot, equalling 10 grains per treatment), the homogenised straw (one sample each per replicate plot), the soils (before and after the trial), and fertilisers (two replicates each). Sulfur isotope analysis of the samples were performed using a Thermo Scientific™ EA IsoLink IRMS Delta V instrument in the Stable Isotope Biogeochemistry Laboratory (SIBL) at Durham University. Isotopic accuracy was monitored through repeated analysis of in-house standards (e.g., sulfanilamide) and international standards (e.g., IAEA-SO-5, IAEA-SO-6, NBS-127). These analytical standards provided an isotopic range from −31 ‰ to +20.3 ‰ in δ34S. Analytical uncertainty in δ34S for replicate analyses of the international standards was ± 0.2 ‰ (2 σ) and ± 0.3 ‰ (2 σ) for in-house standards and replicate sample analysis. Total sulfur concentrations were obtained as part of the isotopic analysis using sulfanilamide (sulfur = 18.62 wt %).
The results of this study were assessed by one-way ANOVA followed by post-hoc Tukey using RStudio software (R Core Team, 2021). The statistical significance threshold was set at α = 0.05. In a previous study, δ13C and δ15N of crop material from this field trial had already been measured (Blanz et al., 2019), the results of which are also discussed in this study with relation to the newly measured δ34S values.
3 Results
3.1 Yield and grain measurements
Total biomass yields (both straw and grains) were highest for barley grown with 50 t seaweed/ha, and lowest for the unfertilised plants, as exemplified in Figure 3. The individual fertilised grains were heavier on average than the unfertilised grains by 10% following 25 t/ha seaweed fertilisation, 15% heavier with 50 t/ha seaweed, and 9% heavier using the NPK mineral fertiliser (based on thousand grain weights measured with present-day agronomic measurement methods using 20 g subsamples; previously published in Brown et al., 2020). Additional yield results (including straw yields) from this trial are reported in detail in Brown et al. (2020).
The grain measurements of randomly selected grains in this study, meant to simulate grain measurements on small numbers of preserved archaeological grains, matched this pattern (see Table 1; Figure 4): all three fertilised sets of grains were demonstrably larger in length, width and depth measurements and heavier than the unfertilised grains. ANOVA followed by post-hoc Tukey indicated significant differences in grain length [F(3, 96) = 16.7, p < 0.001], width [F(3, 96) = 13.1, p < 0.001], depth [F(3, 96) = 16.8, p <0.001], and mass [F(3, 96) = 13.1, p < 0.001] for all comparisons of fertilised grain with unfertilised grain.
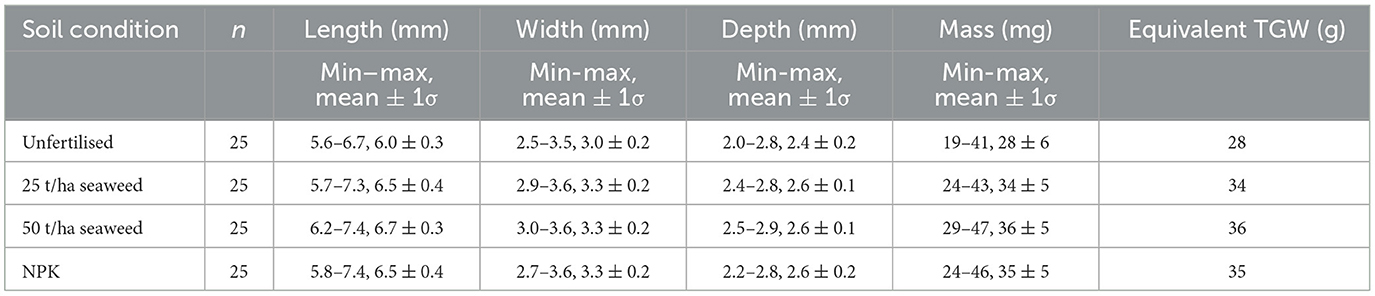
Table 1. Summary of the biomass measurements of the grains from all four treatments, reported as minimum, maximum, mean and standard deviation (σ).
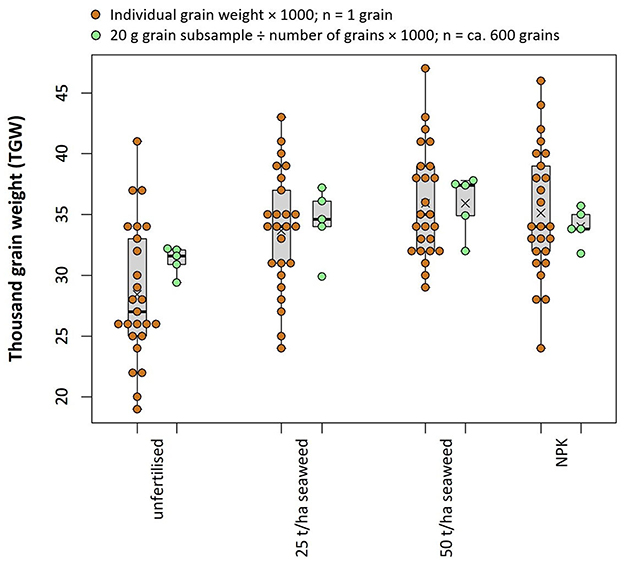
Figure 4. Comparison of thousand grain weight estimates using typical archaeobotanical methods (brown—individual grains weights) and present-day agronomic methods (green—20 g grain aliquot counted using a MARVIN grain analyser). Means are indicated by crosses. All grain originated from the same field trial; data from this study and Brown et al. (2020).
3.2 Stable sulfur isotope ratios
Sulfur isotope ratios of straw and grains from the seaweed-fertilised barley plants ranged between +13.2 ‰ and +16.0 ‰, with the 50 t/ha seaweed fertilised plants having on average slightly higher δ34S values compared to those fertilised with 25 t/ha seaweed (by 0.3 ‰ for grains, and 0.8 ‰ for straw). These δ34S values are higher than for both unfertilised and NPK-fertilised barley (range +10.8 ‰ to +13.4 ‰; Figure 5 and Table 2; all results are provided in the Supplementary material). For grains, ANOVA followed by post-hoc Tukey indicated the δ34S differences to be significant [F(3, 36) = 87.5, p < 0.001, n = 10 per treatment] for all comparisons of seaweed-fertilised grain with unfertilised or NPK fertilised grain (all p < 0.001), whereas differences between the two seaweed-treatments were not found to be significant (p = 0.69), and neither were differences in δ34S between NPK fertilised and unfertilised grains (p = 0.09). Similar results were found for straw following a separate ANOVA followed by post-hoc Tukey [F(3, 16) = 25.1, p < 0.001, n = 5 per treatment], with all comparisons of seaweed-fertilised straw with unfertilised or NPK fertilised grain being significantly different (all p < 0.001). Differences between the two seaweed-treatments were not found to be significant (p = 0.29), and neither were differences in δ34S values between NPK fertilised and unfertilised straw (p = 0.37).
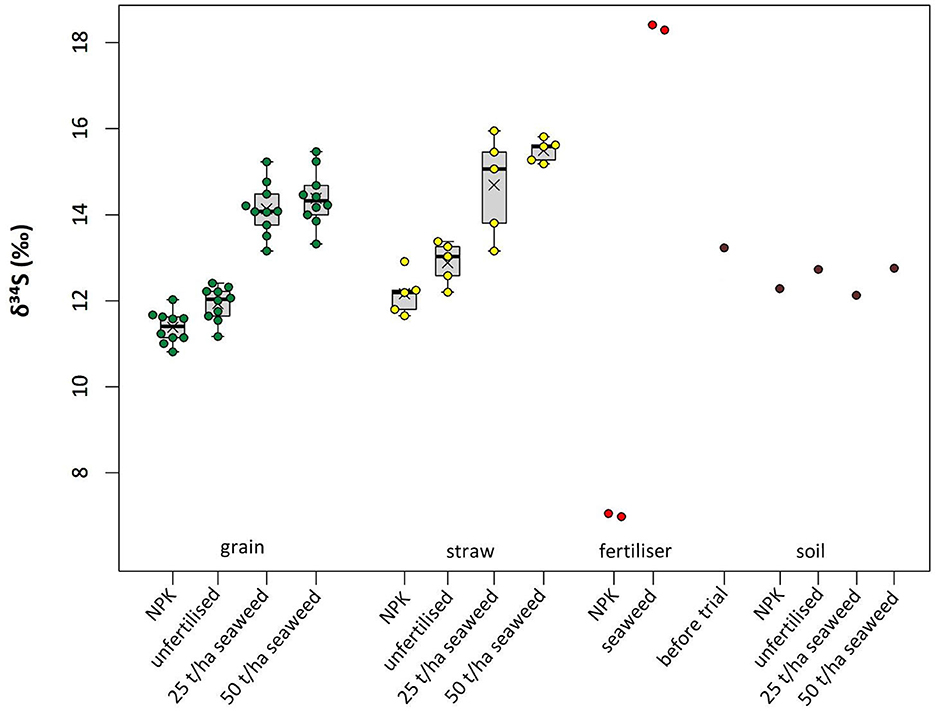
Figure 5. Stable sulfur isotope ratios of bere barley, fertiliser and soil from this field trial. Means are indicated by crosses. The five soil values are from pooled samples taken before (Left) and after the trial (four values on the Right).
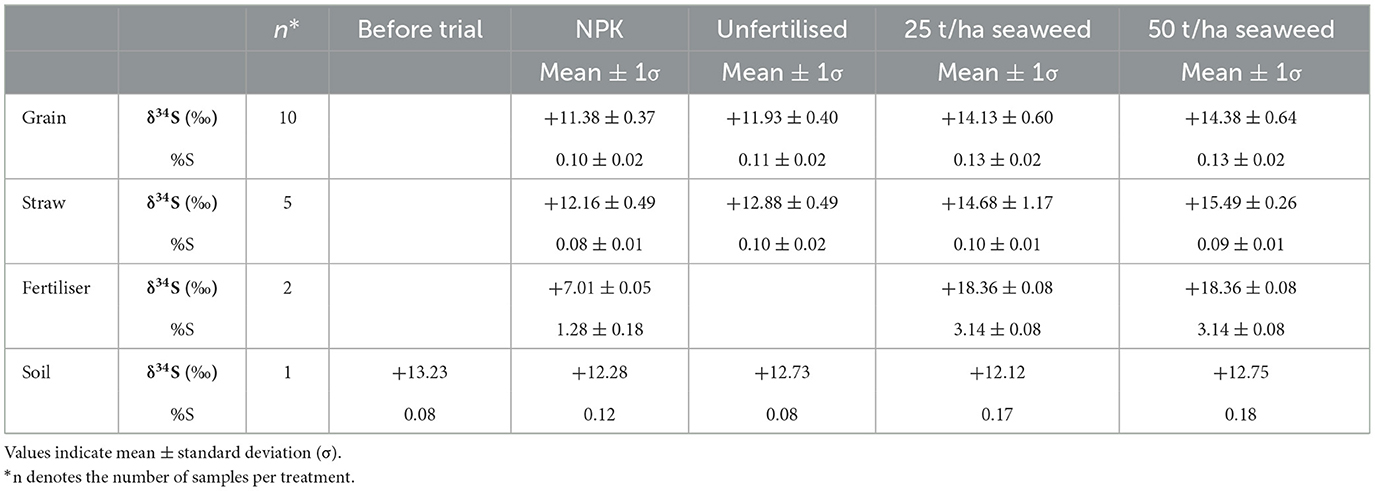
Table 2. Summary of the stable sulfur isotope ratios and concentration sulfur in bere barley, fertilisers and soil from this field trial.
On average, straw tended to have higher δ34S values than grains, except for the 25 t/ha seaweed treatment, where the straw results were variable. The NPK fertiliser had the lowest δ34S value (+7.0 ‰) in this study, and the seaweed used as fertiliser had the most positive (+18.4 ‰). Soils had δ34S values between +12.1 ‰ and +13.2 ‰. By comparison, seaweed-fertilised grain and straw had higher δ34S values than the soil after the field trial (an increase of +2.4 ‰ on average), whereas NPK-fertilised and unfertilised grains had lower δ34S values than the soil (a decrease of −0.8 ‰ on average), and unfertilised straw and NPK-fertilised straw had similar values to the soil after the field trial. A comparison to δ13C and δ15N values of bere barley grains from the same field trial (previously published in Blanz et al., 2019) is shown in Figure 6, and indicates a moderate correlation between δ34S and δ15N [statistically significant with r(18) = 0.67, p = 0.001], but no correlation with δ13C [r(18) = 0.06, p = 0.8].
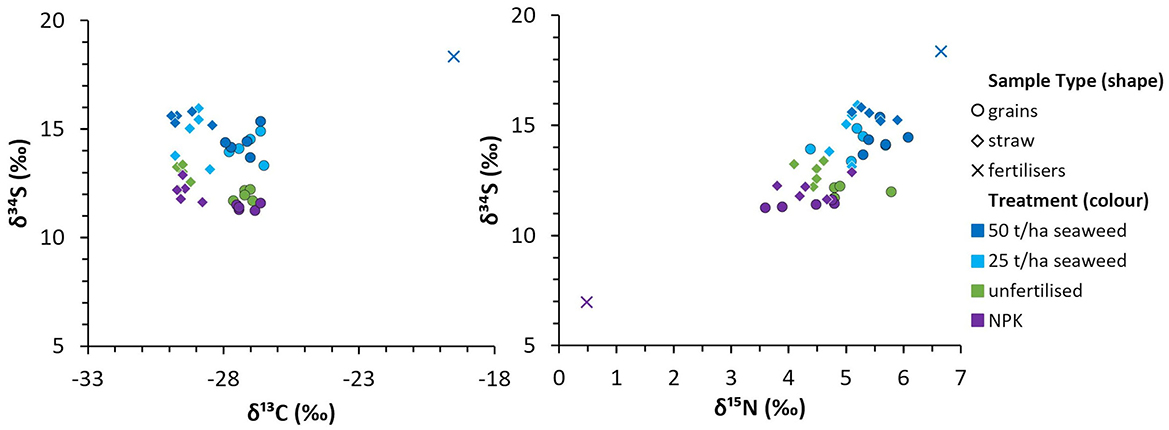
Figure 6. Comparison of δ34S with δ13C and δ15N values from the same trial; δ13C and δ15N data from Blanz et al. (2019).
4 Discussion
4.1 Grain measurements
In this field trial, fertilisation with seaweed and NPK fertiliser both led to an increase in grain size. However, increased grain size is not in itself diagnostic of fertilisation: increased fertilisation frequently leads to larger number of tillers and ears, which may then result in a larger overall yield, but smaller individual grain sizes (see Bingham et al., 2007; Shah et al., 2017). Additionally, droughts, for example, can also lead to small grain sizes despite fertilisation, whereas a low seeding density (including growth on edges of fields) can lead to larger amounts of nutrients being available per plant and thus, larger grains despite a lack of fertilisation. Therefore, grain size itself is not sufficient to determine the nutritional status of a plant.
Nevertheless, these results show that fertilisation can increase size and mass of hulled bere barley grains and that an equivalent thousand grain weight (TGW) can be estimated based on the average mass of 25 individual grains multiplied by 1,000 (see Table 1). This estimate compares very well to the TGWs estimated using 20 g grain samples from each plot in this field trial using present-day agronomic standard methods (see Figure 4; Brown et al., 2020). These results indicate that in archaeological contexts, where it is frequently impossible to recover, identify, weigh and count similarly large amounts of complete grains, it should still be possible to arrive at reasonable estimations of TGW using smaller sample sizes (here n = 25), albeit with larger associated uncertainties.
Ferrio et al. (2004) have outlined a method to estimate individual grain weights from carbonised archaeological cereal grains, based on furnace experimentation of modern grains of wheat and barley. By combining the estimated individual weights of carbonised cereal grain assemblages, it is therefore possible to convert the averages of the grain weights of the individual archaeological cereal grains into TGW estimations, allowing direct comparison to the modern agronomic metric of TGW to the archaeological assemblages. This has clear research implications for understanding long-term trajectories of cereal productivity over thousands of years in different parts of the world (cf. Gron et al., 2021).
4.2 Sulfur stable isotope ratios
The δ34S values of the seaweed-fertilised barley were elevated by ~2 ‰ compared to unfertilised barley. This was most likely caused by the elevated δ34S value of the seaweed fertiliser (+18.4 ‰) compared to the soil prior to the trial (+12.7 ‰), as sulfur from decaying seaweed is incorporated into the growing bere barley. The absence of a statistically significant difference between the two seaweed treatments is likely due to the barley only taking up a limited amount of sulfur, and thus more available sulfur did not directly lead to a greater increase in δ34S.
For the NPK fertilised barley, the opposite effect, i.e., a lowering of δ34S compared to the unfertilised barley, was expected due to the low δ34S value of the NPK fertiliser (+7.0 ‰); however, this difference was found to be less pronounced than for the seaweed fertilised barley (see Figure 5). This is likely because the NPK fertiliser application introduced less sulfur to the soil owing to the lower application rate and sulfur content of the mineral fertiliser (ca. 5 kg sulfur ha−1) compared to seaweed fertilisation (ca. 785 kg sulfur ha−1 and 1,570 kg sulfur ha−1 for the treatments of 25 t/ha seaweed and 50 t/ha seaweed, respectively—although we hypothesise that not all of this sulfur would have been bioavailable during the course of this field trial).
The results of this study are similar to those previously reported for Celtic beans (Gröcke et al., 2021), where seaweed fertilisation led to ~10 ‰ higher δ34S values compared to unfertilised beans (i.e., +16.2 ‰ in seaweed-fertilised beans and +6.6 ‰ in unfertilised beans in 2017). The much smaller difference in δ34S between seaweed-fertilised and unfertilised barley in the present study is likely in part due to the comparatively high initial soil δ34S value in the present study of +12.7 ‰. As the soil for the field trial in this study already had relatively high δ34S values, the fertilisation of barley with seaweed likely had a smaller effect on δ34S than it would have had in a soil with low δ34S values. Additionally, the soil had a higher wt% sulfur concentration after the trial compared to Gröcke et al. (2021). Thus, when a sulfur-rich biofertiliser is added to a soil with low background sulfur concentrations (a macronutrient) a plant will more effectively incorporate and fractionate sulfur.
The origin of the higher soil δ34S values in the field trial on Orkney is most likely its close proximity to the ocean, with its turbulent weather causing the frequent occurrence of sea spray and oceanic-influenced rain. This is supported by a δ34S isoscape of Northern Ireland showing areas sheltered from oceanic influence to have lower δ34S values than more exposed areas, and elevated δ34S values in sheep from the Orkney Island of North Ronaldsay (Guiry and Szpak, 2020). Historical seaweed-fertilisation in the field trial area may have also elevated soil δ34S values, as ethnographic and historical descriptions from the sixteenth to early twentieth century indicate seaweed fertilisation was common on Orkney (Fenton, 1997). Past elevated sulfur isotope ratios might have been preserved to some extent in the acidic clay loam soil (see work on clayey peat in Bottrell and Coulson, 2003). If seaweed-fertilisation does indeed increase soil δ34S values in the long-term, this might be useful as a marker for seaweed-fertilisation in non-coastal areas (possibly together with elevated soil δ15N values; Blanz et al., 2019; Commisso and Nelson, 2010, 2007, 2006; Gröcke et al., 2021). Although we show in this study seaweed-fertilisation can elevate soil δ34S, detecting such a difference in practise might prove difficult. Additional sulfur isotope research on modern coastal environments and islands is required in order to develop more robust interpretations from δ34S of archaeological grains and soils.
5 Conclusion: implications for archaeological studies and future directions
Fertilisation with seaweed and NPK fertiliser both led to increased grain sizes and the individual measurements of grain sizes corresponded to the TGW for each treatment. It is therefore possible to convert the averages of the estimated grain weights of the individual archaeological cereal grains (following the methodology of Ferrio et al., 2004) into TGW estimations, allowing direct comparison to the modern agronomic metric of TGW to archaeological assemblages. This has important implications for estimating long-term trajectories of cereal productivity over thousands of years in different parts of the world.
Furthermore, this study showed that seaweed fertilisation can increase δ34S values of cereal crops. Where archaeological crops are sufficiently well-preserved to measure δ34S (as described in Nitsch et al., 2019), the use of seaweed [and/or fish (Gröcke et al., 2021) and/or guano (Szpak et al., 2019)] as a biofertiliser as opposed to terrestrial animal manure may therefore be identified by elevated (i.e., marine) δ34S values. However, in a cautionary tale, Guiry and Szpak (2020) reported elevated bone collagen δ34S values from present-day sheep from (Orkney) and thus, δ34S could not be used to discriminate seaweed-consumption. Other studies are also showing the complexity of modern coastal environments and its impact on not only sulfur, but also nitrogen and carbon (e.g., Göhring et al., 2023). More isotopic research on water, soils, plants and animals from island environments is required. This work has shown that archaeological studies relying on δ34S values, for example in palaeodietary studies to identify marine and freshwater wetland grazing (e.g., Guiry et al., 2021, 2022; Lamb et al., 2023), to quantify consumption of freshwater and/or marine fish (e.g., Nehlich et al., 2011), or to study geographic origin (e.g., Vika, 2009), need to take into account the possibility of seaweed fertilisation and/or consumption.
Additional field experimental studies on different soils will provide more information on the impact of seaweed and/or fish (i.e., marine) biofertilisation on δ34S in plants, and how long the input of seaweed remains in the soil. Other aquatic biofertilisers (e.g., shells, fish, and seawater) and their impact on δ34S in wild plants and crops also need to be investigated. While seaweed was likely the most common marine biofertiliser in the European North Atlantic area in the last few centuries (cf. Blanz et al., 2019), historical sources from the nineteenth and twentieth centuries also attest to the use of seagrasses (which differ from seaweeds by having true roots, stems, and the ability to produce flowers and seeds) and freshwater plants (Fernández et al., 2022; Sharma, 1971; Wyllie-Echeverria and Cox, 1999). In some cases, these plants have much lower δ34S values—e.g., eelgrass (Zostera marina) leaves are reported to have δ34S values around +4 ‰ (likely due to uptake of sulfide; Holmer and Hasler-Sheetal, 2014): other studies have shown benthic organisms in tidal flats can produce low and even negative δ34S values (e.g., Yamanaka et al., 2003). Biofertilisation with such plants, and/or growing crops in coastal regions where sulfide processes occur, can be expected to produce low or negative crop δ34S values. Similarly, biofertiliser mixtures of seaweed (sulfate-influenced) and seagrass (sulfide-influenced) may counteract each other and have very limited impact on crop δ34S values: this will primarily depend on the original soil δ34S value.
Data availability statement
The original contributions presented in the study are included in the article/Supplementary material, further inquiries can be directed to the corresponding authors.
Author contributions
MB: Conceptualisation, Data curation, Formal analysis, Investigation, Methodology, Resources, Software, Validation, Visualisation, Writing – original draft, Writing – review & editing. DRG: Conceptualisation, Data curation, Funding acquisition, Investigation, Methodology, Resources, Validation, Writing – review & editing. PM: Investigation, Methodology, Resources, Writing – review & editing, Conceptualisation, Funding acquisition. MJC: Data curation, Investigation, Methodology, Writing – original draft, Writing – review & editing.
Funding
The author(s) declare financial support was received for the research, authorship, and/or publication of this article. This research was partially funded by the European Social Fund and Scottish Funding Council as part of Developing Scotland's Workforce in the Scotland 2014–2020 European Structural and Investment Fund Programme. The contribution of staff from the University of the Highlands and Islands' Agronomy Institute and the James Hutton Institute to the field trial was supported by Rural and Environment Science and Analytical Services (RESAS) funding from the Scottish Government. DRG also gratefully acknowledges a Natural Environmental Research Council (NERC) Strategic Environmental Science Capital Call grant (#CC018) that provided funding for the purchase of a dedicated sulfur isotope ratio mass spectrometer in SIBL. Open access was funded by a UKRI Open Access Block Award awarded to the University of Durham.
Acknowledgments
This manuscript was improved considerably thanks to comments from Eric Guiry and Jennifer R. Jones as part of peer-review. The authors would like to thank Ingrid Mainland and her family for their help collecting and storing the decomposing seaweed, and John Wishart and Burkart Dietrich for their help in undertaking the field trial.
Conflict of interest
The authors declare that the research was conducted in the absence of any commercial or financial relationships that could be construed as a potential conflict of interest.
Publisher's note
All claims expressed in this article are solely those of the authors and do not necessarily represent those of their affiliated organizations, or those of the publisher, the editors and the reviewers. Any product that may be evaluated in this article, or claim that may be made by its manufacturer, is not guaranteed or endorsed by the publisher.
Supplementary material
The Supplementary Material for this article can be found online at: https://www.frontiersin.org/articles/10.3389/fearc.2024.1465082/full#supplementary-material
References
Beuning, K. R. M., and Scott, J. E. (2002). Effects of charring on the carbon isotopic composition of grass (Poaceae) epidermis. Palaeogeogr. Palaeoclimatol. Palaeoecol. 177, 169–181. doi: 10.1016/S0031-0182(01)00358-3
Bingham, I. J., Blake, J., Foulkes, M. J., and Spink, J. (2007). Is barley yield in the UK sink limited? II. Factors affecting potential grain size. F. Crop. Res. 101, 212–220. doi: 10.1016/j.fcr.2006.11.004
Bishop, R. R., Gröcke, D. R., Ralston, I., Clarke, D., Lee, D. H. J., Shepherd, A., et al. (2022). Scotland's first farmers: new insights into early farming practices in North-west Europe. Antiquity 96, 1087–1104. doi: 10.15184/aqy.2022.107
Blanz, M., Ascough, P., Mainland, I., Martin, P., Taggart, M. A., Dieterich, B., et al. (2019). Seaweed fertilisation impacts the chemical and isotopic composition of barley: implications for analyses of archaeological skeletal remains. J. Archaeol. Sci. 104, 34–44. doi: 10.1016/j.jas.2019.02.003
Bogaard, A., Heaton, T. H. E., Poulton, P., and Merbach, I. (2007). The impact of manuring on nitrogen isotope ratios in cereals: archaeological implications for reconstruction of diet and crop management practices. J. Archaeol. Sci. 34, 335–343. doi: 10.1016/j.jas.2006.04.009
Bogaard, A., and Outram, A. K. (2013). Palaeodiet and beyond: stable isotopes in bioarchaeology. World Archaeol. 45, 333–337. doi: 10.1080/00438243.2013.829272
Bottrell, S. H., and Coulson, J. P. (2003). Preservation of environmental sulfur isotope records in maritime peats: a test of baseline pre-anthropogenic signal and diagenetic effects in a mid-Pleistocene peat. Chem. Geol. 201, 185–190. doi: 10.1016/S0009-2541(03)00163-3
Bottrell, S. H., and Newton, R. J. (2006). Reconstruction of changes in global sulfur cycling from marine sulfate isotopes. Earth-Science Rev. 75, 59–83. doi: 10.1016/j.earscirev.2005.10.004
Brown, L. K., Blanz, M., Wishart, J., Dieterich, B., Schmidt, S. B., Russell, J., et al. (2020). Is bere barley specifically adapted to fertilisation with seaweed as a nutrient source? Nutr. Cycl. Agroecosyst. 118, 149–163. doi: 10.1007/s10705-020-10090-w
Church, M. J. (2002). “The archaeological and archaeobotanical implications of a destruction layer in Dun Bharabhat, Lewis,” in In the Shadow of the Brochs: The Iron Age in Scotland, eds. B. Ballin-Smith and I. Banks (Stroud: Tempus), 67–75.
Commisso, R. G., and Nelson, D. E. (2006). Modern plant δ15N values reflect ancient human activity. J. Archaeol. Sci. 33, 1167–1176. doi: 10.1016/j.jas.2005.12.005
Commisso, R. G., and Nelson, D. E. (2007). Patterns of plant δ15N values on a Greenland Norse farm. J. Archaeol. Sci. 34, 440–450. doi: 10.1016/j.jas.2006.06.012
Commisso, R. G., and Nelson, D. E. (2010). Stable nitrogen isotopic examination of Norse sites in the Western settlement of Greenland. J. Archaeol. Sci. 37, 1233–1240. doi: 10.1016/j.jas.2009.12.023
Craine, J. M., Brookshire, E. N. J., Cramer, M. D., Hasselquist, N. J., Koba, K., Marin-Spiotta, E., et al. (2015). Ecological interpretations of nitrogen isotope ratios of terrestrial plants and soils. Plant Soil 396, 1–26. doi: 10.1007/s11104-015-2542-1
Fernández, E., Barañano, C., Villasante, S., and Méndez, G. (2022). Historical evolution of the social perception on ecosystem services provided by seagrasses through analysis of the written press in North West Spain (1860-2020). Ocean Coast. Manag. 216:105983. doi: 10.1016/j.ocecoaman.2021.105983
Ferrio, J. P., Alonso, N., Voltas, J., and Araus, J. L. (2004). Estimating grain weight in archaeological cereal crops: a quantitative approach for comparison with current conditions. J. Archaeol. Sci. 31, 1635–1642. doi: 10.1016/j.jas.2004.04.006
Fraser, R. A., Bogaard, A., Charles, M., Styring, A. K., Wallace, M., Jones, G., et al. (2013). Assessing natural variation and the effects of charring, burial and pre-treatment on the stable carbon and nitrogen isotope values of archaeobotanical cereals and pulses. J. Archaeol. Sci. 40, 4754–4766. doi: 10.1016/j.jas.2013.01.032
Fraser, R. A., Bogaard, A., Heaton, T., Charles, M., Jones, G., Christensen, B. T., et al. (2011). Manuring and stable nitrogen isotope ratios in cereals and pulses: towards a new archaeobotanical approach to the inference of land use and dietary practices. J. Archaeol. Sci. 38, 2790–2804. doi: 10.1016/j.jas.2011.06.024
Fuller, D., Colledge, S., Murphy, C., and Stevens, C. J. (2017). “Sizing up cereal variation: patterns in grain evolution revealed in chronological and geographical comparisons,” in Scelánea En Homenaje a Lydia Zapata Peña (1965-2015), eds. J. F. Eraso, J. A. M. Alustiza, and Á. A. Valbuena (Bilbao: Servicio Editorial Universidad Del País Vasco), 133–148.
Fuller, D. Q., and Weisskopf, A. (2020). Barley: origins and development. Encyclop. Glob. Archaeol. 63, 1302–1306.
Georgi, M., Voerkelius, S., Rossmann, A., Graßmann, J., and Schnitzler, W. H. (2005). Multielement isotope ratios of vegetables from integrated and organic production. Plant Soil 275, 93–100. doi: 10.1007/s11104-005-0258-3
Göhring, A., Hölzl, S., Mayr, C., and Strauss, H. (2023). Multi-isotope fingerprints of recent environmental samples from the Baltic coast and their implications for bioarchaeological studies. Sci. Tot. Environ. 874:162513. doi: 10.1016/j.scitotenv.2023.162513
Gröcke, D. R. (2020). Carbon isotope stratigraphy—principles and applications. Stratigr. Timescales 5, 1–40. doi: 10.1016/bs.sats.2020.08.002
Gröcke, D. R., Treasure, E. R., Lester, J. J., Gron, K. J., and Church, M. J. (2021). Effects of marine biofertilisation on Celtic bean carbon, nitrogen and sulphur isotopes: implications for reconstructing past diet and farming practices. Rapid Commun. Mass Spectrom. 35:8985. doi: 10.1002/rcm.8985
Gron, K. J., Larsson, M., Gröcke, D. R., Andersen, N. H., Andreasen, M. H., Bech, J. H., et al. (2021). Archaeological cereals as an isotope record of long-term soil health and anthropogenic amendment in southern Scandinavia. Quat. Sci. Rev. 253:106762. doi: 10.1016/j.quascirev.2020.106762
Guiry, E., Kennedy, J. R., Malcom, C., Miller, M., Hall, O., Buckley, M., et al. (2024). Archaeological evidence for long-term human impacts on sea turtle foraging behaviour. R. Soc. Open Sci. 11:240120. doi: 10.1098/rsos.240120
Guiry, E., Noël, S., and Fowler, J. (2021). Archaeological herbivore δ13C and δ34S provide a marker for saltmarsh use and new insights into the process of 15N-enrichment in coastal plants. J. Archaeol. Sci. 125:105295. doi: 10.1016/j.jas.2020.105295
Guiry, E. J., Orchard, T. J., Needs-Howarth, S., and Szpak, P. (2022). Freshwater wetland-driven variation in sulfur isotope compositions: implications for human paleodiet and ecological research. Front. Ecol. Evol. 10, 1–12. doi: 10.3389/fevo.2022.953042
Guiry, E. J., and Szpak, P. (2020). Seaweed-eating sheep show that δ34S evidence for marine diets can be fully masked by sea spray effects. Rapid Commun. Mass Spectrom. 34, 1–10. doi: 10.1002/rcm.8868
Haahr, M. (2010). Random.org: True Random Number Service. Dublin: Sch. Comput. Sci. Stat. Trinity Coll.
Hendrick, J. (1898). The use and value of seaweed as manure. Trans. Highl. Agric. Soc. Scotl. 1898, 119–134.
Holmer, M., and Hasler-Sheetal, H. (2014). Sulfide intrusion in seagrasses assessed by stable sulfur isotopes-a synthesis of current results. Front. Mar. Sci. 1, 1–12. doi: 10.3389/fmars.2014.00064
Kanstrup, M., Thomsen, I. K., Andersen, A. J., Bogaard, A., and Christensen, B. T. (2011). Abundance of 13C and 15N in Emmer, Spelt and Naked Barley Grown on differently manured soils: towards a method for identifying past manuring practice. Rapid Commun. Mass Spectrom. 25, 2879–2887. doi: 10.1002/rcm.5176
Lamb, A. L., Chenery, C. A., Madgwick, R., and Evans, J. A. (2023). Wet Feet: developing sulfur isotope provenance methods to identify wetland inhabitants. R. Soc. Open Sci. 10, 1–19. doi: 10.1098/rsos.230391
Larsson, M., and Bergman, J. (2023). Experimental approach to evaluate the effect of growing conditions on cereal grain size and its relevance for interpreting archaeological cereal grain assemblages. J. Archaeol. Sci. 152:105752. doi: 10.1016/j.jas.2023.105752
Makarewicz, C. A., and Sealy, J. (2015). Dietary reconstruction, mobility, and the analysis of ancient skeletal tissues: expanding the prospects of stable isotope research in archaeology. J. Archaeol. Sci. 56, 146–158. doi: 10.1016/j.jas.2015.02.035
Martin, P., Wishart, J., Cromarty, A., and Chang, X. (2008). “Orkney Bere—developing new markets for an old crop,” in New Crops and Uses: Their Role in a Rapidly Changing World, eds. J. Smartt and N. Haq (Southampton: Centre for Underutilised Crops), 359–372.
Mizota, C., and Sasaki, A. (1996). Sulfur isotope composition of soils and fertilizers: differences between Northern and Southern Hemispheres. Geoderma 71, 77–93. doi: 10.1016/0016-7061(95)00091-7
Nehlich, O. (2015). The application of sulphur isotope analyses in archaeological research: a review. Earth-Sci. Rev. 142, 1–17. doi: 10.1016/j.earscirev.2014.12.002
Nehlich, O., Fuller, B. T., Jay, M., Mora, A., Nicholson, R. A., Smith, C. I., et al. (2011). Application of sulphur isotope ratios to examine weaning patterns and freshwater fish consumption in Roman Oxfordshire, UK. Geochim. Cosmochim. Acta 75, 4963–4977. doi: 10.1016/j.gca.2011.06.009
Neill, T. P. O. (1970). Some Irish techniques of collecting seaweed. Folk Life 8:54. doi: 10.1179/043087770798241454
Nitsch, E. K., Lamb, A. L., Heaton, T. H. E., Vaiglova, P., Fraser, R., Hartman, G., et al. (2019). The preservation and interpretation of δ34S values in charred archaeobotanical remains. Archaeometry 61, 161–178. doi: 10.1111/arcm.12388
Peterkin, A. (1820). Rentals of the Ancient Earldom and Bishoprick of Orkney; With Some Other Explanatory and Relative Documents. Edinburgh: John Moir.
R Core Team (2021). R: A Language and Environment for Statistical Computing. Vienna: R Found. Stat. Comput.
Richards, M. P., Fuller, B. T., and Hedges, R. E. M. (2001). Sulphur isotopic variation in ancient bone collagen from Europe: implications for human palaeodiet, residence mobility, and modern pollutant studies. Earth Planet. Sci. Lett. 191, 185–190. doi: 10.1016/S0012-821X(01)00427-7
Sayle, K. L., Brodie, C. R., Cook, G. T., and Hamilton, W. D. (2019). Sequential measurement of δ15N, δ13C and δ34S values in archaeological bone collagen at the Scottish Universities Environmental Research Centre (SUERC): a new analytical frontier. Rapid Commun. Mass Spectrom. 33, 1258–1266. doi: 10.1002/rcm.8462
Schoeninger, M. J., and Moore, K. (1992). Bone stable isotope studies in archaeology. J. World Prehistory 6, 247–296. doi: 10.1007/BF00975551
Shah, S. S. M., Chang, X., and Martin, P. (2017). Effect of nitrogen, phosphorous, potassium, plant growth regulator and artificial lodging on grain yield and grain quality of a landrace of barley. Int. J. Environ. Agric. Biotechnol. 2, 2020–2032. doi: 10.22161/ijeab/2.4.60
Szpak, P. (2014). Complexities of nitrogen isotope biogeochemistry in plant-soil systems: implications for the study of ancient agricultural and animal management practices. Front. Plant Sci. 5, 1–19. doi: 10.3389/fpls.2014.00288
Szpak, P., and Buckley, M. (2020). Sulfur isotopes (δ34S) in Arctic marine mammals: indicators of benthic vs. pelagic foraging. Mar. Ecol. Prog. Ser. 653, 205–216. doi: 10.3354/meps13493
Szpak, P., Longstaffe, F. J., Macdonald, R., Millaire, J. F., White, C. D., and Richards, M. P. (2019). Plant sulfur isotopic compositions are altered by marine fertilizers. Archaeol. Anthropol. Sci. 11, 2989–2999. doi: 10.1007/s12520-018-0716-5
Tcherkez, G., and Tea, I. (2013). 32S/34S isotope fractionation in plant sulphur metabolism. New Phytol. 200, 44–53. doi: 10.1111/nph.12314
Vika, E. (2009). Strangers in the grave? Investigating local provenance in a Greek Bronze Age mass burial using δ34S analysis. J. Archaeol. Sci. 36, 2024–2028. doi: 10.1016/j.jas.2009.05.022
Wyllie-Echeverria, S., and Cox, P. A. (1999). The seagrass (Zostera marina [Zosteraceae]) industry of nova scotia (1907–1960). Econ. Bot. 53, 419–426. doi: 10.1007/BF02866721
Yamanaka, T., Mizota, C., and Shimoyama, S. (2003). Sulfur isotopic variations in soft tissues of five benthic animals from the reductive, tidal-flat sediments in Northern Kyushu, Japan. Mar. Biol. 142, 327–331. doi: 10.1007/s00227-002-0946-y
Keywords: sulfur stable isotope ratios (δ34S), seaweed, fertilisation, barley, archaeobotany, SDG: life on land, thousand grain weight (TGW)
Citation: Blanz M, Gröcke DR, Martin P and Church MJ (2024) The effect of seaweed fertilisation on sulfur isotope ratios (δ34S) and grain size in barley: implications for agronomy and archaeological research. Front. Environ. Archaeol. 3:1465082. doi: 10.3389/fearc.2024.1465082
Received: 15 July 2024; Accepted: 16 October 2024;
Published: 25 November 2024.
Edited by:
Richard Madgwick, Cardiff University, United KingdomReviewed by:
Eric Guiry, University of Leicester, United KingdomJennifer R. Jones, University of Central Lancashire, United Kingdom
Copyright © 2024 Blanz, Gröcke, Martin and Church. This is an open-access article distributed under the terms of the Creative Commons Attribution License (CC BY). The use, distribution or reproduction in other forums is permitted, provided the original author(s) and the copyright owner(s) are credited and that the original publication in this journal is cited, in accordance with accepted academic practice. No use, distribution or reproduction is permitted which does not comply with these terms.
*Correspondence: Magdalena Blanz, bWFnZGFsZW5hLmJsYW56QHVuaXZpZS5hYy5hdA==; Darren R. Gröcke, ZC5yLmdyb2NrZUBkdXJoYW0uYWMudWs=