- Department of World Languages and Cultures, Iowa State University, Ames, IA, United States
Introduction: We assess the feasibility of white-tailed deer (Odocoileus virginianus) antlers to serve as archives of information on paleoseasonality by analyzing stable isotope values from four modern white-tailed deer collected in central Iowa, USA. Because antlers develop from early spring to early fall, they may serve as an archive for intra-annual seasonal variations and provide snapshots of past climatic and environmental conditions.
Methods: Intra-antler samples were collected from the proximal end to distal end along the main beam of each antler and analyzed for carbon and nitrogen stable isotope values from collagen (δ13Ccol, δ15Ncol) and carbon and oxygen stable isotope values in bioapatite (δ13Capa, δ18Oapa). Stable isotope data were then correlated with local climate data (precipitation and temperature) from the months leading up to the date of death of each deer and with the 50-year averages of the region.
Results: No consistent seasonal patterning was observed between local climate data and isotopic variables across the antlers. δ13Capa values from each antler, however, do show a trend of being negatively correlated with precipitation variables and mean temperature.
Discussion: The results of this exploratory study suggest that individual deer feeding behaviors, mobility, and habitat preferences make it difficult to infer seasonal environmental conditions from antler stable isotope values. We suggest, however, that intra-antler stable isotope data may be useful for wildlife management and conservation studies.
1 Introduction
Stable isotope values of animal bones can serve as paleoclimatic and paleoenvironmental proxies if the diet, feeding and drinking ecology, and habitat size of the organism are well understood (Hedges et al., 2004; Kohn and Law, 2006). Archaeologists are increasingly applying such studies in efforts to reconstruct the past environments in which humans and their ancestors lived. Because animal species of the Cervidae family, including deer, were commonly hunted and consumed by humans, their remains are often encountered in archaeological middens or trash deposits, thus serving as an available archive of past paleoclimatic and paleoenvironmental information. Indeed, previous studies on cervid bone and teeth have found significant relationships between the stable isotope values obtained from skeletal tissues and aspects of the local climate and environment in which they lived (Luz et al., 1990; Cormie and Schwarcz, 1996; Stevens et al., 2006; Rivera-Araya and Pilaar Birch, 2018), indicating their utility in paleoenvironmental studies.
In this paper, we build on this previous research and explore the use of antler bone as an archive of environmental information. We present new data from an intra-antler stable isotope analysis of four white-tailed deer (Odocoileus virginianus) antlers collected from known locations and months within central Iowa, USA. We take advantage of the known local precipitation and temperature data available for the region and assess how intra-antler stable isotope values changed over a well-documented season of development. White-tailed deer is a widespread species across the Americas with a fossil record dating back to the early Pliocene epoch, making its remains abundant and thus an attractive proxy for past environmental conditions. To explore intra-antler variation in isotopic values, we took 10 samples across the primary beam of four different antlers and analyzed them for stable isotope ratios of carbon and nitrogen from antler collagen (δ13Ccol, δ15Ncol) and carbon and oxygen from antler bioapatite (δ13Capa, δ13Oapa). Antler stable isotope values are assessed relative to seasonal climatic changes (precipitation and temperature) obtained from local meteorological stations. The results are discussed within the context of white-tailed deer behavioral ecology and developmental antler biology.
2 White-tailed deer
2.1 Diet and mobility
White-tailed deer are large, generalized herbivores with a widespread distribution across southern Canada, North America, and northern South America (Halls, 1984). While individual deer may live up to 20–25 years, they rarely live longer than 2–3 years in the wild (Halls, 1984; Smith, 1991). Primary food items include forbs, grasses, shrubs, fruit, acorns, and leaves (Vangilder et al., 1982; Fritzell, 2017; McGovern et al., 2020). Although they are dietary generalists, white-tailed deer will narrow their diet to preferred food species contingent on the season (Zagata and Haugen, 1973; Huegel et al., 1985; Fritzell, 2017). Deer from central Iowa also feed within agricultural fields, including cultivated crops during the growing season from June through October and agricultural residue during the winter. Major crops in Iowa include maize (Zea mays) and soybeans (Glycine max).
White-tailed deer tend to remain within a well-defined home range. The size of their home range and the extent of their mobility, however, depends on local habitat, water, and food availability and quality (Huegel et al., 1985; McGovern et al., 2020). While buck fawns and adult bucks tend to have a greater home range than female fawns and does, the general habitat range of deer in Iowa is typically around 80 hectares with daily movement of ~2 km, depending on the season (Zagata and Haugen, 1973). Scarcity of food and breeding activity increases home ranges for fall and winter, while they remain rather small in the spring and summer because of abundant food supplies and fawn rearing. Even though deer tend to remain in the area where they were born, spring extension is observed, with some individuals being documented to travel as far as 80 km away from their place of birth. Furthermore, deer are willing to move to a different core area if danger is felt, generally because of hunting, predator-prey dynamics, and infrastructural expansion (Zagata and Haugen, 1973; Huegel et al., 1985; Fritzell, 2017; McGovern et al., 2020).
2.2 Antlers
Typically, only white-tailed deer males grow antlers. They occur in a variety of shapes and sizes from small and unbranched to large and branched (approximate range of length from 7 to 70 cm) (French et al., 1956; Grasman and Hellgren, 1993; Price et al., 2005; Dryden, 2016; Asleson et al., 2017). The size of antlers is determined by their phase of development, genetics, and by the age of the individual (Scribner et al., 1989). Generally, the size of antlers increases each year of an individual's life until about 5 years of age, at which point the antlers have reached their maximum size (Hewitt et al., 2014). Unlike horns that are made of bone covered by a layer of keratin and are permanently part of the animal, antlers are made of bone, temporarily covered in a velvet membrane, and are shed and regrown annually (Moen et al., 1999; Price et al., 2005; Kierdorf and Kierdorf, 2010). Trabecular bone (transporting nutrients and growth-regulating hormones) and cortical bone (compact bone that forms the hard outer structure) comprise the two types of bone within an antler (Banks, 1974; Gomez et al., 2013). Both bone types are composed of organic collagen and mineral hydroxyapatite.
The process of antler growth is defined as modified endochondral ossification and is described as the remodeling of cartilage by osteoclasts at the tip of each branch (Allen et al., 2002; Kierdorf and Kierdorf, 2010; Rössner et al., 2021). This regeneration process is later constituted of micro-lamellar bone and then primary osteons (Faucheux et al., 2001; Price et al., 2005). Antlers grow from pedicles as an extension of the frontal bone following an annual growth cycle starting and ending around late February to early March. During the growth phase, antlers are covered by a fine and soft membrane called velvet that supplies blood and nutrients to the antler. A steady antler growth from the tip (~1.3 cm per week) during April and May contrasts with the rapid growth that occurs in June and July (~5 cm per week). This inconsistent growth rate is marked by a circulating testosterone increase during the mineralization process from late summer to early fall, causing the shed of velvet (French et al., 1956; Fennessy and Suttie, 1985; Asleson et al., 2017). As the antler mineralizes, chondroclast cells resorb cartilage while osteoblast cells secrete new bone tissue. During the winter, osteoclast cells demineralize along the abscission line situated on the coronet, allowing the casting of antlers. Importantly, compact cortical bone is the most resistant to diagenesis, and thus mature antlers can preserve over long periods of time in the archaeological record (Moen et al., 1999).
3 Stable isotope analysis
3.1 Principles of isotopic analysis and cervid bone
Because white-tailed deer antlers are made of bone, they may be analyzed for stable isotope ratios of carbon, nitrogen, and oxygen in a similar manner as other skeletal elements. For carbon, the primary factor that produces variation in δ13C values within a given environment is the differences in photosynthetic pathways of the plants at the base of the ecosystem. Atmospheric CO2 is fixed in plants through C3, C4, and Crassulacean acid metabolism (CAM) photosynthetic pathways. These photosynthetic pathways result in varying δ13C values within plant tissues, with C3 plants exhibiting relatively low values, C4 plants exhibiting relatively high (less negative) values, and CAM plants varying between these two depending on environmental circumstances (Bender, 1968; Smith and Epstein, 1971; Borland et al., 2011). Within C3 plants, additional variation in δ13C values can occur due to the influences of precipitation, humidity, temperature, amount of sunlight, and salinity of the soil (Tieszen, 1991; Kohn, 2010; Bonafini et al., 2013; Schoeninger et al., 2016). The δ13C values of herbivore bone reflect the δ13C values of consumed plants, where lower δ13C values are indicative of greater C3 dietary intake and higher δ13C values indicative of greater C4 and or CAM plant intake (DeNiro and Epstein, 1978). Stable carbon isotope values can be obtained from two phases of antler bone: the organic collagen phase (δ13Ccol) and the mineral bioapatite phase (δ13Capa). δ13Capa values reflect the δ13C values of dietary inputs from all macronutrient sources (i.e., carbohydrate, protein, lipids), while δ13Ccol values are biased toward the δ13C values of dietary protein sources (Ambrose and Norr, 1993; Froehle et al., 2010). Within white-tailed deer tissues, stable carbon isotope values in both collagen and apatite are thus the product of the amount of maize (predominant agricultural byproduct of Iowa) relative to non-maize plant consumption as well as the seasonal fluctuation in C3 plant δ13C values. While native C4 grasses do occur in Iowa, the vast areas of land dedicated to maize agriculture lead us to believe that any C4 signal detected within antler stable isotope values likely originated with consumption of agricultural maize.
δ15N values of mammalian bone are primarily influenced by the δ15N values from dietary protein sources (DeNiro and Epstein, 1981; Schoeninger and DeNiro, 1984). δ15N values of animal tissues reflect the position of an individual within the trophic system, with plants having the lowest values and carnivores having the highest values (Minagawa and Wada, 1984; Robinson, 2001; Bocherens and Drucker, 2003). For herbivores, stable nitrogen isotope values in skeletal tissue can be used to reflect aspects of the local environment (Somerville et al., 2018, 2020). δ15N values of organic tissues of herbivores reflect the δ15N values of the plants they consume, which are themselves influenced by soil δ15N values. Globally, δ15N values of soil and the plants that grow from it are influenced by temperature and precipitation (Murphy and Bowman, 2006, 2009; Hartman, 2011; Craine et al., 2015a,b), and such differences in δ15N values between environmental zones is incorporated into the tissues of organisms that consume them. Generally, organisms feeding in cool, wet environments exhibit the lowest δ15N values while organisms feeding in hot, dry environments exhibit the highest δ15N values (Ambrose, 1991; Murphy and Bowman, 2009; Hartman, 2011; Somerville et al., 2018). An additional source of variation in nitrogen values comes from human inputs to the soil. Plants growing out of soil supplemented by manuring and fertilizers show an increase in δ15N values, which can then be transferred to the tissues of animals that consume them (Fraser et al., 2011; Szpak et al., 2012).
δ18O values in mammalian bone tissue reflect the δ18O values of the water consumed by the organism (Luz et al., 1984; Ayliffe and Chivas, 1990; Huertas et al., 1995). Because the δ18O value of oxygen from the air is relatively constant worldwide (Kroopnick and Craig, 1972), the primary source of variation in δ18O values of mammalian skeletal tissue is the variation of δ18O values of consumed water, either imbibed or consumed with food (Huertas et al., 1995; Levin et al., 2006). Factors such as altitude, distance from the sea, temperature, precipitation, and relative humidity can influence the δ18O values of precipitation and hence meteoric water sources (Longinelli, 1984; Ayliffe and Chivas, 1990; Poage and Chamberlain, 2001). For meteoric water in a given location, the strongest influence on δ18O values is the local temperature, which implies that δ18O values in the skeletal tissue of obligate drinking organisms will correlate with local temperature variation (Dansgaard, 1964; Iacumin et al., 1996; Hallin et al., 2012). For non-obligate drinking organisms that obtain water primarily from the plants they consume, skeletal δ18O values correlate more strongly with local relative humidity and precipitation levels than temperature as leaf water is subject to the additional oxygen isotope fractionation process of evapotranspiration that results in 18O enrichment in leaf water during dryer/warmer periods (Burk and Stuiver, 1981; Ayliffe and Chivas, 1990; Levin et al., 2006). The magnitude of the change in oxygen isotope ratios is influenced by relative humidity and precipitation, with higher humidity and greater precipitation leading to lower δ18O values in leaf water and hence tissues of animals that consume them (Huertas et al., 1995; Levin et al., 2006; Somerville et al., 2018). White-tailed deer's primary source of water is leaf water, but they will drink surface water on occasion, particularly when temperatures are high (Michael, 1968). δ18O values from deer bone then are influenced both by changes in both local moisture and temperature (Luz et al., 1990).
3.2 Previous isotopic studies on cervids
Previous studies have demonstrated the potential of the isotopic ratios of C, N, and O in bone and enamel of cervids to reflect aspects of diet and the environmental context in which they lived. For δ13C values, early analyses of bone collagen of modern Odocoileus virginianus specimens from North America found a positive relationship between mean temperature and δ13C values of bone collagen, presumably due to the greater consumption of C4 plants during warm summer months (Cormie and Schwarcz, 1996). Research on red deer (Cervus elaphus) populations in European environments that do not contain C4 plants, however, observed a negative relationship between temperature and δ13C values (Stevens et al., 2006). Despite the ambiguous general relationship between temperature and stable carbon isotope values, studies on red deer δ13C values across the late Pleistocene to early Holocene find significantly higher values during the cool/dry Pleistocene than in the warm/wet Holocene, demonstrating the ability of these values to reflect general changes in properties of the landscape over time (Drucker et al., 2003; Hedges et al., 2004).
For δ15N values, a negative correlation between precipitation levels and δ15N values in bone collagen of modern Odocoileus virginianus of North America was observed, but only when individual deer had consumed > 10% C4 plants (Cormie and Schwarcz, 1996). Analyses of δ15N values from red deer bone collagen from Europe, however, did not find a significant relationship with precipitation levels; instead they found a strong positive correlation between δ15N values and temperature from each site (Stevens et al., 2006). Such varying results highlight the complexity of interpreting mammalian nitrogen isotope values and the challenges inherent to cross-regional comparisons.
In addition to bone, previous studies have documented the potential of cervid enamel to serve as an archive of paleoenvironmental information. Both studies of white-tailed deer (Rivera-Araya and Pilaar Birch, 2018) and red deer (Stevens et al., 2011) have found correlations between patterns of change in δ18O values from intra-tooth sub-samples and those of seasonal change in δ18O values of local precipitation, which are themselves strongly influenced by changes in temperature. In deer, enamel mineralizes during the first 1–3 years of development and captures the isotopic signature of the food and water consumed during that time. This process, however, necessitates caution when interpreting enamel δ18O values as developmental changes such as nursing and weaning can be captured in the enamel signature and thus obscure environmental signals, particularly in the first molar (Malasek et al., 2023).
Isotopic values of cervid antlers, like bone and enamel, reflect aspects of their diet and environment. Research on white-tailed deer with known diets shows a consistent offset between diet and antler δ13C and δ15N values of +4.5 ± 0.16‰ and +4.29 ± 0.29‰, respectively (Darr and Hewitt, 2008). Research that conducted inter-antler analysis of collagen stable carbon and nitrogen isotope values found that bone isotope values and antler isotope values are not in equilibrium and that considerable variation could exist within antlers (Stevens and O'Connell, 2016). The present study attempts to explain to what extent intra-antler variation of white-tail deer antlers can be explained by seasonal variation in diet, temperature, and precipitation variables.
4 Materials and methods
4.1 Samples
The sampled population is comprised of four antlers from wild deer from central Iowa (Figure 1). Three of the specimens were collected by the Iowa Department of Natural Resources (DNR) and one was collected opportunistically from a deer discovered by the authors. Each of the sampled antlers was collected during the fall months and was still attached to the skull at the time of the death. The locations of specimens are displayed in Figure 1. The first antler, PEL-0090, was collected from a hunted white-tailed deer in Jasper County, Iowa. The length (measured along the posterior side) and weight are 50.7 cm and 191 g, respectively. The deer was a juvenile of ~1.5 years of age at its time of death (December 5th, 2016). The antler was donated to this research project by the Iowa DNR.
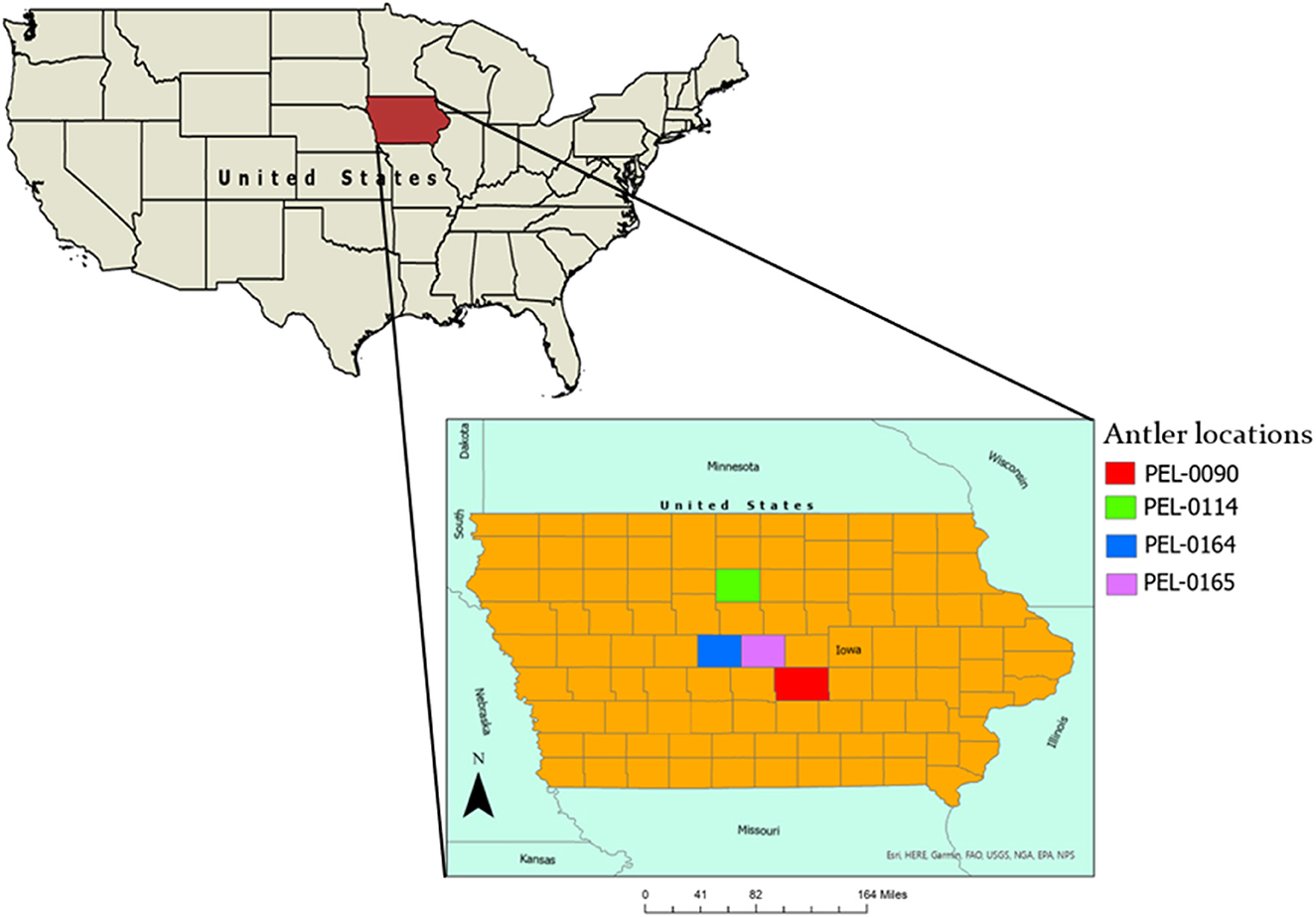
Figure 1. Map of Iowa, USA, displaying the locations from where the analyzed antler specimens were sampled.
Antler PEL-0114 was collected from a hunted white-tailed deer in Wright County, Iowa. The length (measured along the posterior side) and weight are 50.5 cm and 185 g, respectively. The deer was a juvenile of ~1 years old at its time of death (December 3rd, 2016). The antler was donated to this research project by the Iowa DNR.
Antler PEL-0164 was collected from a hunted white-tailed deer in Boone County, Iowa. The length (measured along the posterior side) and weight are 68.6 cm and 366.7 g, respectively. The deer was an adult of ~2.5 years of age at its time of death (late October 2019). The antler was donated to this research project by the Iowa DNR.
Finally, antler PEL-0165 was collected from a discovered dead white-tailed deer from Story County, Iowa by ADS during November 2020. The length (measured along the posterior side) and weight are 21 cm and 63.2 g, respectively. The deer was a juvenile at its time of death.
4.2 Sample preparation
Ten samples of collagen and apatite were obtained for each antler, for a total of 80 samples. Collagen and apatite samples each yielded two isotope variables (δ13Ccol and δ15Ncol for collagen and δ13Capa and δ18Oapa for apatite), for a total of 160 data points that were investigated in this study. To extract powdered antler tissue, the outer layer of the antler that was in direct contact with the external environment was first removed by ablating the surface with a hand-held Dremel tool equipped with a diamond engraving bit before sample extraction. All samples for analysis were then collected from these cleaned areas by extracting powdered samples using a Dremel tool with a clean diamond engraving bit along the posterior side of the antlers from the proximal end to the distal end.
The samples were prepared for the procedure of carbon and nitrogen isotope analysis of collagen by modifying established methods (Longin, 1971; Sealy et al., 2014). Samples of ~50 mg of cortical antler bone were powdered and weighed out into 15 mL polypropylene tubes. Approximately 10 mL of 0.25 M HCl was added to each tube for demineralization, which were then mixed on the touch mixer for 45–60 s. The caps were then loosened, and the tubes sat at room temperature for 48 h. Samples were then rinsed with ultrapure H2O to neutralize. Because all samples were modern, the alkaline soak in sodium hydroxide (NaOH) solution and the filtration steps were skipped. Tubes with collagen gelatin were placed with closed caps in an oven at 85°C for 24 h to solubilize. Finally, the collagen samples were frozen and lyophilized in a freeze dryer for 24 h.
The samples were prepared for the procedure of carbon and oxygen isotope analysis of carbonate in bone mineral apatite (Ca10([PO4, CO3])6(OH)2) by following a modified version of established procedures that are commonly used on archaeological specimens (Koch et al., 1997; Crowley and Wheatley, 2014). Although the antler specimens are not likely to have suffered diagenetic alteration, we used the same treatment protocol as archaeological specimens to make data from potential future studies of ancient specimens comparable to those of this modern sample. Sample sizes of ~30 mg were weighed. Then, 1,200 μL of 2% bleach (NaOCl) was added to the samples, mixed, and left to sit at room temperature for 24 h. The bleach was then decanted and samples were rinsed three times with ultrapure water. One thousand two hundred microliter of 1 M acetic acid (CH3COOH) buffered to a pH of 5 was added to the samples, which were then mixed and left to sit at room temperature for 24 h. The water rinse process was once again applied and then the samples, placed in a tray uncapped, were put in a laboratory oven to dry at 60°C for 24 h.
Mass spectrometry of collagen and apatite samples occurred in the Stable Isotope Laboratory in the Department of Geological and Atmospheric Sciences at Iowa State University. Collagen samples were analyzed for carbon and nitrogen isotopes using a Costech Elemental Analyzer coupled to a ThermoFinnigan Delta Plus XL mass spectrometer. Reference standards [Caffeine(USGS-62), Caffeine (IAEA-600), Cellulose (IAEA-CH-3), and Acetanilide (laboratory standard)] were used for isotopic corrections, and to assign the data to the appropriate isotopic scale. Corrections were done using a three-point regression method and isotope results are reported in parts per thousand (per mil, ‰). Percent concentration (%) was calculated using the peak area of the sample. The combined uncertainty (analytical uncertainty and average correction factor) for δ13C is ± 0.1‰ (VPDB) and δ15N is ± 0.2‰ (AIR). For the carbonate apatite samples, carbon and oxygen isotope ratios were analyzed with a Gas Bench with a CombiPAL autosampler coupled to the ThermoFinnigan Delta Plus XL mass spectrometer. Reference standards (NBS-18, IAEA 603) were used for isotopic corrections, and to assign the data to the appropriate isotopic scale. Corrections were done using a two-point regression method and isotope results are reported in parts per thousand (per mil, ‰). The analytical uncertainty for δ13C is ± 0.1‰ (VPDB) and δ18O is ± 0.1‰ (VPDB).
4.3 Climate data
As part of our intra-antler analysis of the relationship between seasonal climatic variables and stable isotope data, we tested correlations between two scales of analysis. First, we used the available monthly climate data from local weather stations made available by the National Oceanic and Atmospheric Administration (NOAA). We obtained monthly mean precipitation, and monthly mean maximum, average, and minimum temperature using weather data from NOAA. This dataset from weather stations was provided by the NOAA climate data online under the local climatological data search tool. Access to the historical weather data allowed us to associate the unique seasonal conditions of the habitat of each individual in the study (NOAA, 2021). The Des Moines international airport station historical data of 2016 is associated with PEL-0090; the Fort Dodge Duncombe school area station historical data of 2016 is associated with PEL-0114; the Fort Dodge RGNL Station historical data of 2019 is associated with PEL-0164 and PEL-0165 (Steremberg et al., 1995). Antlers PEL-0164 and PEL-0165 were found in relatively proximity to each other and therefore share the same local weather station and thus local climate data. Because the maximum and minimum temperature variables were highly correlated with the mean monthly temperatures, we narrowed the analysis to only mean monthly temperature for the temperature variables. Second, we assessed the 50-year mean monthly data from each antler location because monthly rainfall totals can be quite variable at the local level (Figure 2). We obtained the multi-decadal mean monthly precipitation totals from the study regions using the geographic information system software DIVA-GIS (Hijmans et al., 2001, 2005) and the publicly available WorldClim dataset (Hijmans et al., 2005). The WorldClim data represent the interpolated mean monthly values across the period of AD 1950–2000 at each antler location.
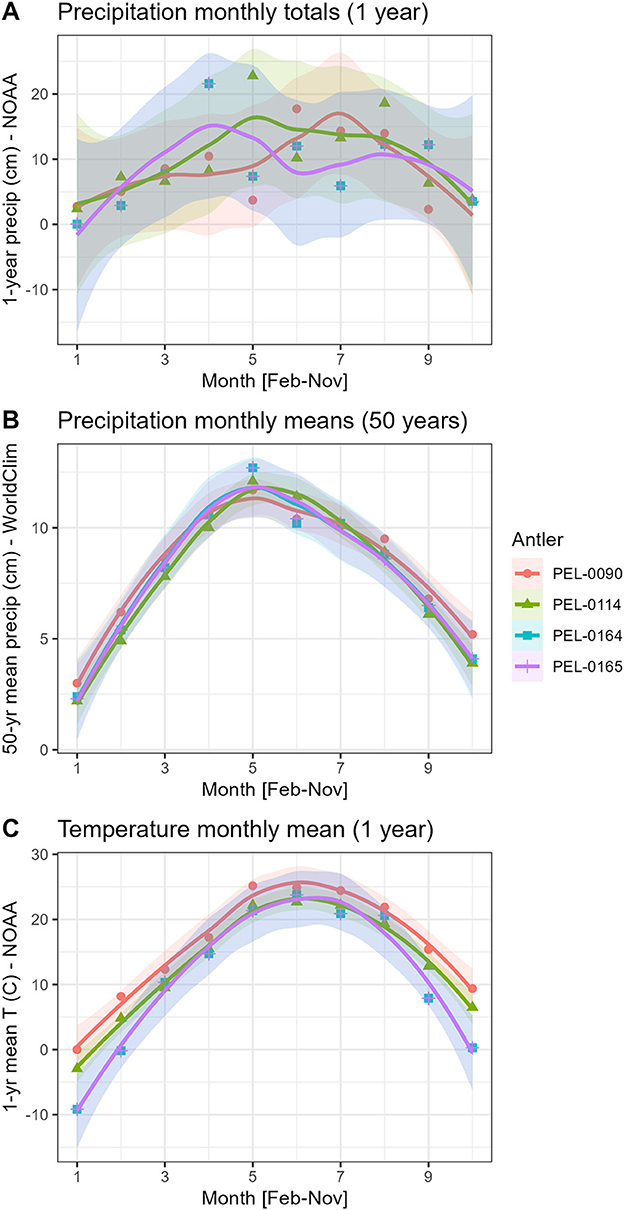
Figure 2. Patterns of seasonal change in precipitation and temperature in central Iowa, USA. The data represent two scales: a multi-decade scale based on 50 year monthly averages (DIVA-GIS/WorldClim data) and a monthly scale with monthly totals (NOAA data). (A) Monthly precipitation totals; (B) mean monthly precipitation totals over 50-year period; (C) mean monthly temperatures. Note that PEL-0164 and PEL-0165 share the same climate station and hence the same climate data.
4.4 Statistical analysis
Analysis of variance tests (ANOVA) were used to compare the mean stable isotope values between antlers, and linear regression models were used to analyze the relationship between the dependent isotope values and the independent variables of temperature and precipitation. Each antler had 10 evenly spaced samples taken from along the main beam. Resulting stable isotope values from each of these sub samples were associated with monthly mean environmental variables obtained from the closest weather stations (Figure 1). Based on the knowledge of antler growth patterns, which begin in February, and the knowledge of the date of death of each deer specimen, we matched the 1–10 sample sequence from each antler with the calendar dates of February-November to assess how seasonal changes in the local climate are reflected in the isotopic values of the antlers. This association of a month of development with a stable isotope value is conducted with the acknowledgment that this is not a precise pairing because antler velvet tends to be shed a month or two prior to November. Nevertheless, we believe that assessing the data in this way captures the patterns of seasonal change through time, with cooler periods at the beginning and ending of antler development. By analyzing the data in this manner we may assess how the extreme seasonal changes in environmental conditions of central Iowa might influence the intra-antler stable isotope data. Indeed, all analyzed antlers should have the earliest (most proximal) values representing climatic conditions of around February, samples from the middle of the antlers should correlate to the summer months, and the most distal samples should represent the early fall months (see Figure 2). Statistical analyses and vizualizations were conducted in the R computing environment (version R-4.0.2) (R Core Team, 2021). In addition to statistical tests of mean differences and intra-antler isotope/climate correlations, we also qualitatively assess the isotopic patterning of the isotopic data within the antlers. If seasonal changes strongly influence deer isotope values, then we would expect to see a similar U-shaped pattern within each antler reflecting the beginning and end of the summer season.
5 Results
Results of the stable isotope analyses of the antler collagen and apatite phases are available in the Supplementary material. The results demonstrated strong separation in stable isotope values between antlers (Figure 3; Table 1). Separate one-way analysis of variance (ANOVA) tests confirmed that significant differences exist between the isotopic values of each antler (P < 0.001), except for δ18Oapa values which were not significantly different between antlers (P = 0.098). Additionally, we assessed the patterns of change over time within each antler. These can be viewed in Figure 4, which shows the isotope values as a function of their distance in cm from the pedicle, and in Figure 5, which shows the isotope values as a function of their relative position (1–10) from the pedicle, with 1 representing the most proximal sample and 10 representing the most distal sample. For antler PEL-0164, the fifth δ18Oapa value from the pedicle (PEL-0164.134), which corresponds to the month of June in our analyses, exhibited an abnormally high value of −1.5‰ relative to the overall average of −6.8‰ for rest of the intra-antler samples of this individual (see Figure 3). Because this may have been due to an error in sample processing, analysis, or due to a highly abnormal water acquisition strategy for the individual deer that month, we excluded this singular point for the statistical analysis.
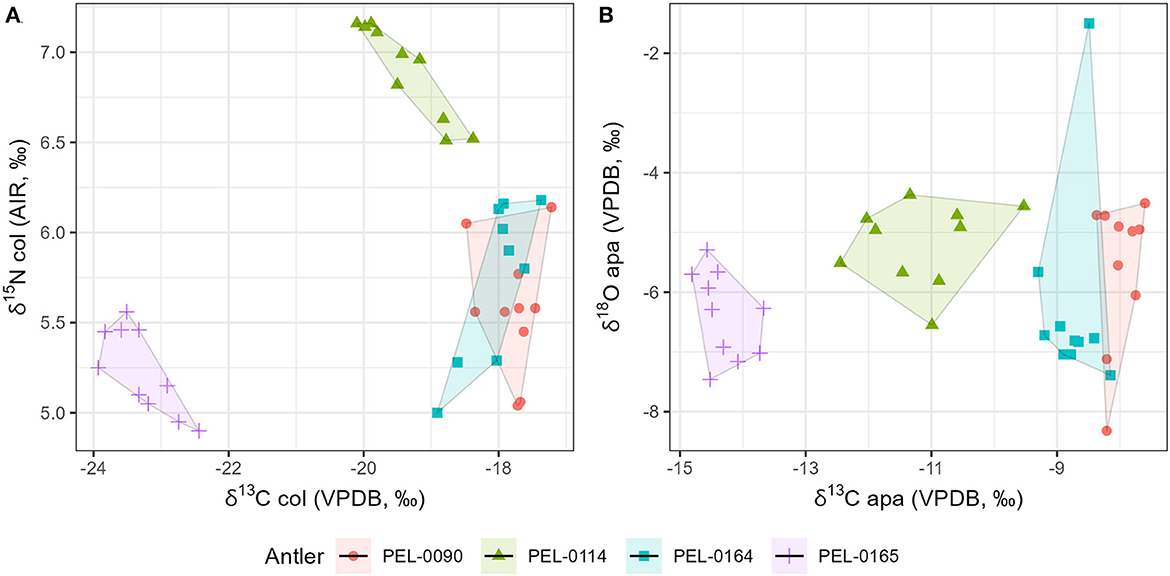
Figure 3. Scatterplot of stable isotope data from intra-antler samples. The data include stable carbon and nitrogen values from collagen samples (A), and stable carbon and oxygen isotope values from antler apatite (B). Sample shapes and colors vary according to each antler specimen analyzed.
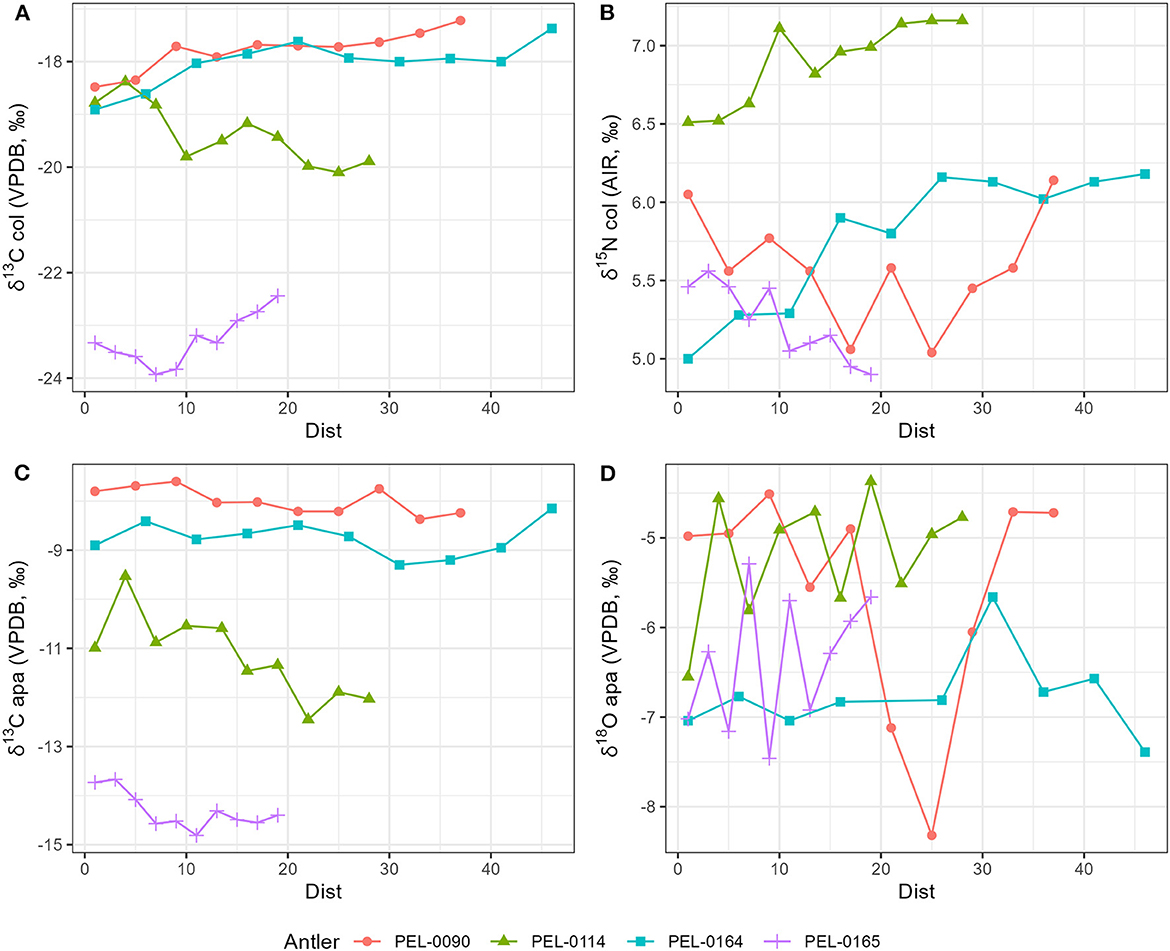
Figure 4. Stable isotope data plotted as a function of the sample's distance in centimeters (dist) from the proximal base of each antler. The most distal samples represent the most recent period of antler development and the most proximal samples represent the oldest portion of the antlers. (A) Stable carbon isotope values from antler collagen; (B) stable nitrogen isotope values from antler collagen; (C) stable carbon isotope values from antler apatite; (D) stable oxygen isotope values from antler apatite.
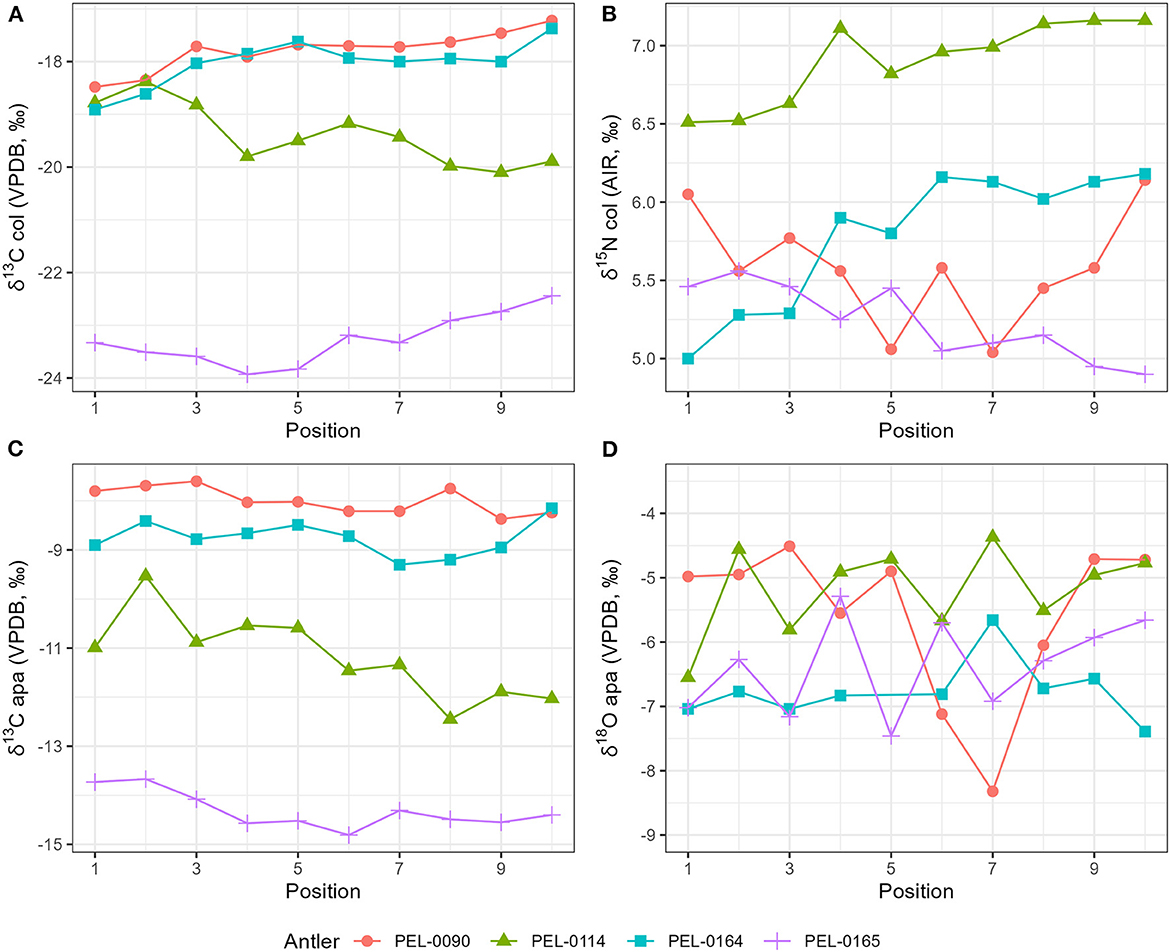
Figure 5. Stable isotope data plotted as a function of the sample position along the main beam of each antler. The 1 position represents the most proximal sample and the 10 position represents the most distal sample. The period of development corresponds roughly to the calendar months of February through November. (A) Stable carbon isotope values from antler collagen; (B) stable nitrogen isotope values from antler collagen; (C) stable carbon isotope values from antler apatite; (D) stable oxygen isotope values from antler apatite.
To explore intra-antler variation in stable isotope values (δ13Ccol, δ15Ncol, δ13Capa, δ18Oapa) with reference to known seasonal changes of the regions in which they lived, we compared stable isotope data with known local weather data from the stations closest to each antler. For all antlers, the relationships between isotopic values and climate are visualized in Figure 6 for precipitation and Figure 7 for temperature. First, we examined the carbon and nitrogen isotope data from antler collagen with reference to the climate data. No significant correlations were observed between δ13Ccol antler values and any climate variable. For δ15N values, moderate to strong correlations were found with the 1-year (NOAA) mean temperature data within antlers PEL-0090 (R = −0.80), PEL-0114 (R = 0.54), and PEL-0164 (R = 0.62), but not PEL-0165 (R = −0.25). Notably however, while the correlation in δ15N values and temperature for PEL-0090 is negative, the relationship is positive for PEL-0114 and PEL-0164. Additionally, δ15N values of PEL-0090 were strongly correlated with the 50-year precipitation data (WorldClim; R = −0.79), but not in any other antler.
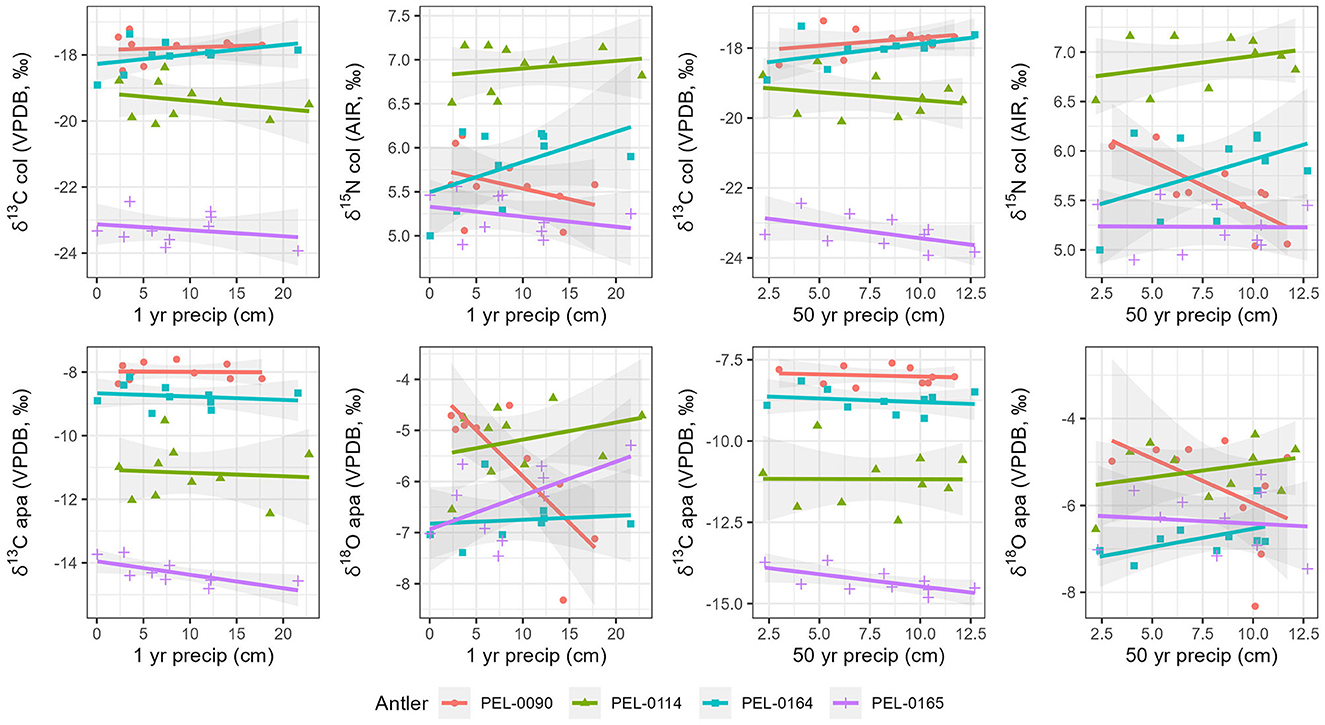
Figure 6. Scatterplots displaying relationships between local precipitation variables and stable isotope values from intra-antler samples. Marker color and shape differentiate the sampled antlers.
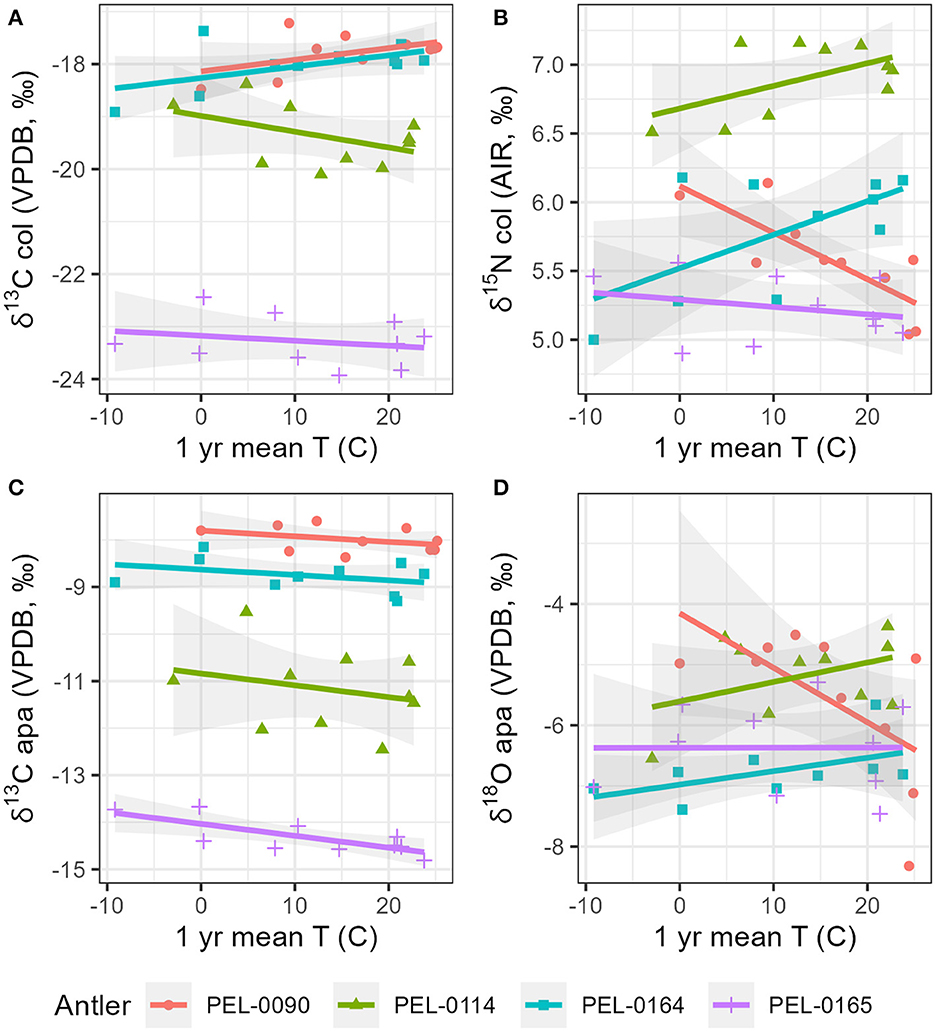
Figure 7. Scatterplots displaying correlations between local mean monthly temperatures and stable isotope values from intra-antler shape differentiate the sampled antlers. (A) Stable carbon isotope values from antler collagen; (B) stable nitrogen isotope values from antler collagen; (C) stable carbon isotope values from antler apatite; (D) stable oxygen isotope values from antler apatite.
Secondly, we explored the relationships between local climate variables and the stable carbon and oxygen values from antler mineral apatite. For the δ13Capa values, only PEL-0165 displayed any moderate to strong correlations with climate variables. Antler PEL-0165 had moderate negative correlations between δ13Capa values and the 1-year monthly temperature means (NOAA; R = −0.76), the 1-year precipitation means (NOAA; R = −0.70), and the 50-year precipitation means (WorldClim; R = −0.65). Notably all correlations between stable carbon isotope values from antler apatite and climate variables were negatively related, indicating a consistent response of this variable to seasonal change. For the δ18Oapa data, the only notable correlations were observed in antler PEL-0090, which had moderate to strong correlations between δ18Oapa and the 1-year temperature monthly means (NOAA; R = −0.61) and with the 50-year monthly precipitation means (WorldClim; R = −0.81). No other antler displayed significant correlations between δ18Oapa values and climate variables.
6 Discussion
The results of this study found significant differences between the means of the intra-antler stable isotope values of carbon and nitrogen from bone collagen and stable isotope values of carbon from antler apatite from each of four white-tailed deer antlers. These differences suggest that, although intra-antler variation in diet did occur, the individual deer consumed distinct diets during the season of antler growth. Antler PEL-0090, for example, exhibited a mean δ13Capa value of −8.0 ± 0.3‰, suggesting a diet relatively high in C4 plants, such as maize, while the antler of PEL-0165 exhibited a mean δ13Capa value of −14.3 ± 0.4‰, suggesting a diet more consistent with natural C3 browse. The clear inter-individual differences in stable isotope values from the individuals sampled from four areas of Iowa suggest that isotopic values of antler tissue can reflect differences across a landscape, or at least the patches to which deer had access.
Within each antler, we investigated the relationship between the four stable isotope variables and local climatic variables from the nearby weather stations. Most isotopic variables did not exhibit clear correlations with the local climatic data. Isotopic values from PEL-0090, however, did exhibit significant negative correlations between stable nitrogen and oxygen isotope variables and precipitation and temperature variables, respectively. Other antlers, however, exhibited positive correlations between these variables, indicating the opposite relationship between δ18Oapa and δ15Ncol values and precipitation and temperature. The only consistent trend to emerge from the isotope-climate correlations was the relationship between δ13Capa values and all climate variables. The intra-antler δ13Capa values from each individual studied exhibited negative correlations with the two precipitation variables and with mean temperature (Figure 5). While these correlations are only significant in PEL-0165, the pattern is consistent across all four antlers. This suggests that among the four isotope variables, δ13Capa values most consistently track seasonal changes in the diet of the deer. It remains unclear, however, whether this trend is driven by seasonal changes in precipitation and temperature or whether it may be a factor of changes in diet of the deer over the course of the period of antler growth. For example, the deer may have fed more on C4 agricultural refuse during the cold winter and fall months but on more natural browse during the warm and wet summer months.
The results of this study in combination with those of previous research highlight both opportunities for future areas of research utilizing intra-antler stable isotope analysis and drawbacks to the method that would need to be accounted for in future studies. We first consider the positive aspects of intra-antler analyses. The results of this study found that intra-antler isotope values of four deer consistently clustered together and that they reflect different diet and ecologies of each individual. This suggests the potential use of isotope values from antlers as proxies to characterize aspects of the local environment in which the deer lived. The intra-antler changes in isotopic values from the proximal to distal ends, moreover, indicate changes in feeding strategies over time. Temporally sensitive data such as these may be particularly useful to wildlife management and conservation efforts. Future isotopic studies of antlers could be used to track the extent of crop raiding by deer and to track how they exploit different environmental patches over the course of antler development.
This study also highlights several aspects of antler analysis that may limit their use in paleoenvironmental studies. Except for a trend of negative correlations between antler δ13Capa values and all climate variables, this study found no consistent patterns between seasonal changes in temperature and precipitation and the intra-antler isotope values across the primary beams. This is especially clear, when the antler values are compared on the same scale (Figure 5) and no consistent patterning is observed. This lack of clear patterning may be due to several factors. The fragmented landscape of Iowa contains large areas dedicated to the farming of maize, a C4 plant, and soybean, a leguminous C3 plant, in addition to areas of more natural riverine and wooded habitat. The movement of deer between such isotopically diverse patches could have resulted in larger changes in the isotopic composition of deer antlers over time than did the seasonal changes in temperature and precipitation.
The inconsistent rate of growth of antlers over time, moreover, presents a challenge to their use as a paleoclimate archive. During the period of peak antler development, which occurs from June to mid-July, the rate of growth can more than triple. This may complicate interpretations of paleo-seasonality as the summer months may be overrepresented in the antler sampling relative to the spring and fall months. Indeed, this irregular growth makes correlating seasonal changes in the climate with isotopic values, such as we have attempted in this study, a difficult task. Unless the differential growth rate across the antler beam can be controlled, the timing of specific changes in diet or environment is prone to errors.
Finally, the movement of male deer can be another factor influencing the utility of the method. While deer generally stay in fairly small habitat ranges, male deer can disperse many kilometers from their place of birth and can move between regions seasonally. This movement can result in deer isotope values not being reflective of the environment in which they were found, especially for carbon and nitrogen stable isotope values, which can vary dramatically between feeding patches. Therefore, because deer are mobile and are selective foragers, they cannot be considered passive reflections of local environmental conditions, and thus any interpretation of paleoclimatic or paleoenvironmental conditions must be made with consideration of these behavioral factors.
While the results overall suggest that intra-antler analyses may not be useful to reconstruct precise changes in climate variables such as precipitation and temperature across the year, they do reflect general differences in the diet and environment in which the individual deer lived. A productive avenue for future paleoclimate studies would be to focus on the intra-antler magnitude of variation across the season. Alternatively, dividing the antler samples into the three broad categories of proximal, intermediate, and distal, could provide general information on conditions during late winter/early spring, summer, and early fall, respectively. With larger sample sizes, such studies may permit the characterization of how pronounced the differences were between the seasons during the period in which the deer were living and growing their antlers.
7 Conclusion
This study analyzed four antlers from white-tailed deer specimens in central Iowa, USA, taking 10 intra-antler samples for four stable isotope variables (δ13Cap, δ18Oap, δ13Ccol, δ15Ncol) from each specimen. The results of the study showed clear differences between the individuals, but, despite the strong seasonal changes across the year within the study region, few meaningful patterns were observed when attempting to correlate intra-antler isotopic variations with known seasonal changes in precipitation and temperature. It is likely that diet and selective feeding strategies had a greater influence on intra-antler stable isotope values than did seasonal changes in climate. Uneven rates of growth of antlers and deer mobility, moreover, likely serve as additional sources of error when attempting to correlate climatic and isotopic variables. To be useful for paleoclimatic or paleoenvironmental research, future studies would likely need large sample sizes or would need to focus on other characteristics of the data that may reflect aspects of the past climate while not assuming a steady rate of growth, such as the within-antler amplitude of variation in isotopic values as proxies for the degree of paleoseasonality. It also remains possible that deer from more C3 dominated landscapes with less human modification may more faithfully track changes in the seasons within their antlers.
Despite the complications of using antlers in paleoclimate research, this study adds to our knowledge about how white-tailed deer diet, behavior, and ecology interact to influence the stable isotope ratios of their antlers. Isotopic studies of antlers seem particularly well-suited for studies of wildlife management and conservation. Intra-antler stable isotope analysis is a non-invasive method that can be performed on already shed antlers. Isotope values of carbon, nitrogen, and oxygen can reflect the extent to which individual deer were consuming human agricultural products over spring, summer, and fall months, and can be used to make inferences about patterns of dietary change over time. Antlers from differing deer population, moreover, could be compared to assess group-level differences in ecology and feeding behavior.
Data availability statement
The original contributions presented in the study are included in the article/Supplementary material, further inquiries can be directed to the corresponding author.
Ethics statement
Ethical approval was not required for the study involving animal remains in accordance with the local legislation and institutional requirements.
Author contributions
JR and AS: project conception and design, data analysis and visualization, and article writing and editing. All authors contributed to the article and approved the submitted version.
Funding
Funding was made possible by professional development funds available to AS from the Department of World Languages and Cultures at Iowa State University.
Acknowledgments
This research was part of the Anthropology MA thesis research of JR in the Department of World Languages and Cultures at Iowa State University. We thank the committee members Matthew G. Hill and Alan Wannamaker for comments on an earlier version of the manuscript. We thank Jeff Barnes and Todd Gosselink of the Iowa Department of Natural Resources for donating three of the antlers for research. Finally, we thank Suzanne Ankerstjerne for support in the stable isotope laboratory.
Conflict of interest
The authors declare that the research was conducted in the absence of any commercial or financial relationships that could be construed as a potential conflict of interest.
AS declared that they were an editorial board member of Frontiers, at the time of submission.
Publisher's note
All claims expressed in this article are solely those of the authors and do not necessarily represent those of their affiliated organizations, or those of the publisher, the editors and the reviewers. Any product that may be evaluated in this article, or claim that may be made by its manufacturer, is not guaranteed or endorsed by the publisher.
Supplementary material
The Supplementary Material for this article can be found online at: https://www.frontiersin.org/articles/10.3389/fearc.2023.1221143/full#supplementary-material
References
Allen, S. P., Maden, M., and Price, J. S. (2002). A role for retinoic acid in regulating the regeneration of deer antlers. Dev. Biol. 251, 409–423. doi: 10.1006/dbio.2002.0816
Ambrose, S. H. (1991). Effects of diet, climate and physiology on nitrogen isotope abundances in terrestrial foodwebs. J. Archaeol. Sci. 18, 293–317. doi: 10.1016/0305-4403(91)90067-Y
Ambrose, S. H., and Norr, L. (1993). “Experimental evidence for the relationship of the carbon isotope ratios of whole diet and dietary protein to those of bone collagen and carbonate,” in Prehistoric Human Bone (Springer), 1–37. doi: 10.1007/978-3-662-02894-0_1
Asleson, M. A., Hellgren, E. V., and Varner, L. W. (2017). Nitrogen requirements for antler growth and maintenance in white-tailed deer. J. Wildl. Manage. 60, 744–752. doi: 10.2307/3802373
Ayliffe, L. K., and Chivas, A. R. (1990). Oxygen isotope composition of the bone phosphate of australian kangaroos: potential as a palaeoenvironmental recorder. Geochim. Cosmochim. Acta 54, 2603–2609. doi: 10.1016/0016-7037(90)90246-H
Banks, W. J. (1974). The ossification process of the developing antler in the white-tailed deer (Odocoileus virginianus). Calcif. Tissue Res. 14, 257–274. doi: 10.1007/BF02060300
Bender, M. M. (1968). Spectrometric studies and theoretical calculations of some β-ketonitriles. Mass Spectr. Stud. Carbon 13 Variat. Corn Other Grasses 10, 468–472. doi: 10.1017/S0033822200011103
Bocherens, H., and Drucker, D. (2003). Trophic level isotopic enrichment of carbon and nitrogen in bone collagen: case studies from recent and ancient terrestrial ecosystems. Int. J. Osteoarchaeol. 13, 46–53. doi: 10.1002/oa.662
Bonafini, M., Pellegrini, M., Ditchfield, P., and Pollard, A. M. (2013). Investigation of the ‘canopy effect'in the isotope ecology of temperate woodlands. J. Archaeol. Sci. 40, 3926–3935. doi: 10.1016/j.jas.2013.03.028
Borland, A. M., Andrea Barrera Zambrano, V., Ceusters, J., and Shorrock, K. (2011). The Photosynthetic plasticity of crassulacean acid metabolism: an evolutionary innovation for sustainable productivity in a changing world. New Phytol. 191, 619–633. doi: 10.1111/j.1469-8137.2011.03781.x
Burk, R. L., and Stuiver, M. (1981). Oxygen isotope ratios in trees reflect mean annual temperature and humidity. Science 211, 1417–1419. doi: 10.1126/science.211.4489.1417
Cormie, A. B., and Schwarcz, H. P. (1996). Effects of climate on deer bone δ15N and δ13C: lack of precipitation effects on δ15N for animals consuming low amounts of C4 plants. Geochim. Cosmochim. Acta 60, 4161–4166. doi: 10.1016/S0016-7037(96)00251-7
Craine, J. M., Brookshire, E. N. J., Cramer, M. D., Hasselquist, N. J., Koba, K., et al. (2015a). Ecological interpretations of nitrogen isotope ratios of terrestrial plants and soils. Plant Soil 396, 1–26. doi: 10.1007/s11104-015-2542-1
Craine, J. M., Elmore, A. J., Wang, L., Augusto, L., Baisden, W. T., et al. (2015b). Convergence of soil nitrogen isotopes across global climate gradients. Sci. Rep. 5, 8280. doi: 10.1038/srep08280
Crowley, B. E., and Wheatley, P. V. (2014). To bleach or not to bleach? Comparing treatment methods for isolating biogenic carbonate. Chem. Geol. 381, 234–242. doi: 10.1016/j.chemgeo.2014.05.006
Dansgaard, W. (1964). Stable isotopes in precipitation. Tellus 16, 436–468. doi: 10.1111/j.2153-3490.1964.tb00181.x
Darr, R. L., and Hewitt, D. G. (2008). Stable isotope trophic shifts in white-tailed deer. J. Wildl. Manage. 72, 1525–1531. doi: 10.2193/2006-293
DeNiro, M. J., and Epstein, S. (1978). Influence of diet on the distribution of carbon isotopes in animals. Geochim. Cosmochim. Acta 42, 495–506. doi: 10.1016/0016-7037(78)90199-0
DeNiro, M. J., and Epstein, S. (1981). Influence of diet on the distribution of nitrogen isotopes in animals. Geochim. Cosmochim. Acta 45, 341–351. doi: 10.1016/0016-7037(81)90244-1
Drucker, D., Bocherens, H., Bridault, A., and Billiou, D. (2003). Carbon and nitrogen isotopic composition of red deer (Cervus elaphus) collagen as a tool for tracking palaeoenvironmental change during the late-glacial and early holocene in the Northern Jura (France). Palaeogeogr. Palaeoclimatol. Palaeoecol. 195, 375–388. doi: 10.1016/S0031-0182(03)00366-3
Dryden, L. G. (2016). Nutrition of antler growth in deer. Anim. Prod Sci. 56, 962–970. doi: 10.1071/AN15051
Faucheux, C., Nesbitt, S. A., Horton, M. A., and Price, J. S. (2001). Cells in regenerating deer antler cartilage provide a microenvironment that supports osteoclast differentiation. J. Exp. Biol. 204, 443–455. doi: 10.1242/jeb.204.3.443
Fennessy, P. F., and Suttie, J. M. (1985). Antler growth: nutritional and endocrine factors. R. Soc. N. Z. Bull. 22, 239–250.
Fraser, R. A., Bogaard, A., Heaton, T., Charles, M., Jones, G., et al. (2011). Manuring and stable nitrogen isotope ratios in cereals and pulses: towards a new archaeobotanical approach to the inference of land use and dietary practices. J. Archaeol. Sci. 38, 2790–2804. doi: 10.1016/j.jas.2011.06.024
French, E. C., McEwen, L. C., Magruder, N. D., Ingram, R. H., and Swift, R. W. (1956). Nutrient requirements for growth and antler development in the white-tailed deer. J. Wildl. Manage. 20, 221. doi: 10.2307/3796954
Fritzell, P. (2017). Trends in Iowa Wildlife Populations and Harvest 2016–2017. Des Moines, IA: Iowa Department of Natural Resources.
Froehle, A. W., Kellner, C. M., and Schoeninger, M. J. (2010). FOCUS: effect of diet and protein source on carbon stable isotope ratios in collagen: follow up to. J. Archaeol. Sci. 37, 2662–2670. doi: 10.1016/j.jas.2010.06.003
Gomez, S., Garcia, A. J., Luna, S., Kierdorf, U., Kierdorf, H., Gallego, L., et al. (2013). Labeling studies on cortical bone formation in the antlers of red deer (Cervus elaphus). Bone 52, 506–515. doi: 10.1016/j.bone.2012.09.015
Grasman, B. T., and Hellgren, E. C. (1993). Phosophorus nutrition in white-tailed deer : nutrient balance, physiological responses, and antler growth. Ecology 74, 2279–2296. doi: 10.2307/1939581
Hallin, K. A., Schoeninger, M. J., and Schwarcz, H. P. (2012). Paleoclimate during neandertal and anatomically modern human occupation at Amud and Qafzeh, Israel: the stable isotope data. J. Hum. Evol. 62, 59–73. doi: 10.1016/j.jhevol.2011.09.005
Hartman, G. (2011). Are elevated δ15N values in herbivores in hot and arid environments caused by diet or animal physiology? Funct. Ecol. 25, 122–131. doi: 10.1111/j.1365-2435.2010.01782.x
Hedges, R. M., Stevens, R. E., and Richards, M. P. (2004). Bone as a stable isotope archive for local climatic information. Quat. Sci. Rev. 23, 959–65. doi: 10.1016/j.quascirev.2003.06.022
Hewitt, D. G., Hellickson, M. W., Lewis, J. S., Wester, D. B., and Bryant, F. C. (2014). Age-related patterns of antler development in free-ranging white-tailed deer. J. Wildl. Manage. 78, 976–984. doi: 10.1002/jwmg.741
Hijmans, R. J., Cameron, S. E., Parra, J. L., Jones, P. G., and Jarvis, A. (2005). Very high resolution interpolated climate surfaces for global land areas. Int. J. Climatol. 25, 1965–1978. doi: 10.1002/joc.1276
Hijmans, R. J., Guarino, L., Cruz, M., and Rojas, E. (2001). Computer tools for spatial analysis of plant genetic resources data: 1. DIVA-GIS. Plant Genet. Resour. Newsl. 127, 15–19.
Huegel, C. N., Dahlgren, R. B., and Gladfelter, H. L. (1985). Use of doe behavior to capture white-tailed deer fawns. Wildl. Soc. Bull. 13, 287–289.
Huertas, D. A., Iacumin, P., Stenni, B., Chillón, B. S., and Longinelli, A. (1995). oxygen isotope variations of phosphate in mammalian bone and tooth enamel. Geochim. Cosmochim. Acta 59, 4299–4305. doi: 10.1016/0016-7037(95)00286-9
Iacumin, P., Bocherens, H., Mariotti, A., and Longinelli, A. (1996). Oxygen isotope analyses of co-existing carbonate and phosphate in biogenic apatite: a way to monitor diagenetic alteration of bone phosphate? Earth Planet. Sci. Lett. 142, 1–6. doi: 10.1016/0012-821X(96)00093-3
Kierdorf, U., and Kierdorf, H. (2010). Deer antlers - a model of mammalian appendage regeneration: an extensive review. Gerontology 57, 53–65. doi: 10.1159/000300565
Koch, L. P., Noreen, T., and Marilyn, L. F. (1997). The effects of sample treatment and diagenesis on the isotopic integrity of carbonate in biogenic hydroxylapatite. J. Archaeol. Sci. 5, 417–429. doi: 10.1006/jasc.1996.0126
Kohn, M. J. (2010). Carbon isotope compositions of terrestrial C3 plants as indicators of (paleo)ecology and (paleo)climate. Proc. Natl. Acad. Sci. U. S. A. 107, 19691–19695. doi: 10.1073/pnas.1004933107
Kohn, M. J., and Law, J. M. (2006). Stable isotope chemistry of fossil bone as a new paleoclimate indicator. Geochim. Cosmochim. Acta 70, 931–946. doi: 10.1016/j.gca.2005.10.023
Kroopnick, P., and Craig, H. (1972). Atmospheric oxygen: isotopic composition and solubility fractionation. Science 175, 54–55. doi: 10.1126/science.175.4017.54
Levin, N. E., Cerling, T. E., Passey, B. H., Harris, J. M., and Ehleringer, J. R. (2006). A stable isotope aridity index for terrestrial environments. Proc. Natl. Acad. Sci. U. S. A. 103, 11201–11205. doi: 10.1073/pnas.0604719103
Longin, R. (1971). New method of collagen extraction for radiocarbon dating. Nature 230, 241–242. doi: 10.1038/230241a0
Longinelli, A. (1984). Oxygen isotopes in mammal bone phosphate: a new tool for paleohydrological and paleoclimatological research? Geochim. Cosmoch. Acta 48, 385–390. doi: 10.1016/0016-7037(84)90259-X
Luz, B., Cormie, B. A., and Schwarcz, H. P. (1990). oxygen isotope variations in phosphate of deer bones. Geochim. Cosmochim. Acta 54, 1723–1728. doi: 10.1016/0016-7037(90)90403-8
Luz, B., Kolodny, Y., and Horowitz, M. (1984). Fractionation of oxygen isotopes between mammalian bone-phosphate and environmental drinking water. Geochim. Cosmochim. Acta 48, 1689–1693. doi: 10.1016/0016-7037(84)90338-7
Malasek, T., Barding, E., Bender, J. M., Bonham, A., Mead, A. J., Pilgrim, Z., et al. (2023). Evaluating the fidelity of enamel isotopic data and environmental variation in paleoecological studies: a case study in wild, known-aged, modern white-tailed deer (Odocoileus virginianus). Palaeogeogr. Palaeoclimatol. Palaeoecol. 622, 111587. doi: 10.1016/j.palaeo.2023.111587
McGovern, G. P., Dinsmore, S. J., and Blanchong, J. A. (2020). Survival of white-tailed deer fawns in Central Iowa. PLoS ONE 15, 1–16. doi: 10.1371/journal.pone.0229242
Michael, E. D. (1968). Drinking habits of white-tailed deer in South Texas. Proc. Southeast. Assoc. Game Fish Commission. 21, 51–57. doi: 10.2307/3896137
Minagawa, M., and Wada, E. (1984). Stepwise enrichment of 15N along food chains: further evidence and the relation between δ15N and animal age. Geochim. Cosmochim. Acta 48, 1135–1140. doi: 10.1016/0016-7037(84)90204-7
Moen, R. A., Pastor, J., and Cohen, Y. (1999). Antler growth and extinction of Irish Elk. Evol. Ecol. Res. 1, 235–249.
Murphy, B. P., and Bowman, D. M. (2006). Kangaroo metabolism does not cause the relationship between bone collagen δ15N and water availability. Funct. Ecol. 20, 1062–1069. doi: 10.1111/j.1365-2435.2006.01186.x
Murphy, B. P., and Bowman, D. M. J. S. (2009). The carbon and nitrogen isotope composition of australian grasses in relation to climate. Funct. Ecol. 23, 1040–1049. doi: 10.1111/j.1365-2435.2009.01576.x
NOAA (2021). NOAA National Centers for Environmental Information. Department of Commerce United States of America (2021). Available online at: https://www.ncei.noaa.gov/about (accessed July 15, 2021).
Poage, M. A., and Chamberlain, C. P. (2001). Empirical relationships between elevation and the stable isotope composition of precipitation and surface waters: considerations for studies of paleoelevation change. Am. J. Sci. 301, 1–15. doi: 10.2475/ajs.301.1.1
Price, J. S., Allen, S., Faucheux, C., Althnaian, T., and Mount, J. G. (2005). Deer antlers: a zoological curiosity or the key to understanding organ regeneration in mammals? J. Anat. 207, 603–618. doi: 10.1111/j.1469-7580.2005.00478.x
R Core Team (2021). R: A Language and Environment for Statistical Computing. Vienna: R Foundation for Statistical Computing.
Rivera-Araya, M., and Pilaar Birch, S. (2018). Stable isotope signatures in white-tailed deer as a seasonal paleoenvironmental proxy: a case study from Georgia, United States. Palaeogeogr. Palaeoclimatol. Palaeoecol. 505, 53–62. doi: 10.1016/j.palaeo.2018.05.025
Robinson, D. (2001). [Delta]. Sup. 15. N as an integrator of the nitrogen cycle. Trends Ecol. Evol. 16, 153–162. doi: 10.1016/S0169-5347(00)02098-X
Rössner, G. E., Costeur, L., and Scheyer, T. M. (2021). Antiquity and fundamental processes of the antler cycle in Cervidae (Mammalia). Sci. Nat. 108, 1–24. doi: 10.1007/s00114-020-01713-x
Schoeninger, M. J., and DeNiro, M. J. (1984). Nitrogen and carbon isotopic composition of bone collagen from marine and terrestrial animals. Geochim. Cosmochim. Acta 48, 625–639. doi: 10.1016/0016-7037(84)90091-7
Schoeninger, M. J., Most, C. A., Moore, J. J., and Somerville, A. D. (2016). Environmental variables across Pan troglodytes study sites correspond with the carbon, but not the nitrogen, stable isotope ratios of chimpanzee hair. Am. J. Primatol. 78, 1055–1069. doi: 10.1002/ajp.22496
Scribner, K. T., Smith, M. H., and Johns, P. E. (1989). Environmental and genetic components of antler growth in white-tailed deer. J. Mammal. 70, 284–291. doi: 10.2307/1381509
Sealy, J., Johnson, M., Richards, M., and Nehlich, O. (2014). Comparison of two methods of extracting bone collagen for stable carbon and nitrogen isotope analysis: comparing whole bone demineralization with gelatinization and ultrafiltration. J. Archaeol. Sci. 47, 64–69. doi: 10.1016/j.jas.2014.04.011
Smith, B. N., and Epstein, S. (1971). Two categories of 13 C/ 12 C ratios for higher plants. Plant Physiol. 47, 380–384. doi: 10.1104/pp.47.3.380
Somerville, A. D., Froehle, A. W., and Schoeninger, M. J. (2018). Environmental Influences on rabbit and hare bone isotope abundances: implications for paleoenvironmental research. Palaeogeogr. Palaeoclimatol. Palaeoecol. 497, 91–104. doi: 10.1016/j.palaeo.2018.02.008
Somerville, A. D., Nelson, B. A., Díaz, J. L. P., and Schoeninger, M. J. (2020). Rabbit bone stable isotope values distinguish desert ecoregions of North America: data from the archaeological sites of Pueblo Grande, La Ferreria, and La Quemada. J. Archaeol. Sci. 113, 105063. doi: 10.1016/j.jas.2019.105063
Stevens, R. E., Balasse, M., and O'Connell, T. C. (2011). Intra-tooth oxygen isotope variation in a known population of red deer: implications for past climate and seasonality reconstructions. Palaeogeogr. Palaeoclimatol. Palaeoecol. 301, 64–74. doi: 10.1016/j.palaeo.2010.12.021
Stevens, R. E., Lister, A. N., and Hedges, R. E. M. (2006). predicting diet, trophic level and palaeoecology from bone stable isotope analysis: a comparative study of five red deer populations. Oecologia 149, 12–21. doi: 10.1007/s00442-006-0416-1
Stevens, R. E., and O'Connell, T. C. (2016). Red deer bone and antler collagen are not isotopically equivalent in carbon and nitrogen. Rapid Commun. Mass Spectr. 30, 1969–1984. doi: 10.1002/rcm.7670
Szpak, P., Millaire, J.-F., White, C. D., and Longstaffe, F. J. (2012). Influence of seabird guano and camelid dung fertilization on the nitrogen isotopic composition of field-grown maize (zea mays). J. Archaeol. Sci. 39, 3721–3740. doi: 10.1016/j.jas.2012.06.035
Tieszen, L. L. (1991). Natural variations in the carbon isotope values of plants: implications for archaeology, ecology, and paleoecology. J. Archaeol. Sci. 18, 227–248. doi: 10.1016/0305-4403(91)90063-U
Vangilder, L. D., Torgerson, O., and Porath, W. R. (1982). Factors influencing diet selection by white-tailed deer. J. Wildl. Manage. 711–18. doi: 10.2307/3808563
Keywords: paleoclimate, paleoenvironment, stable isotope analysis, deer, Cervidae
Citation: Royer J and Somerville AD (2023) Assessing the use of stable isotope values from deer antlers as proxies for seasonal environmental variation. Front. Environ. Archaeol. 2:1221143. doi: 10.3389/fearc.2023.1221143
Received: 11 May 2023; Accepted: 31 October 2023;
Published: 28 November 2023.
Edited by:
Patrick Roberts, Max Planck Institute for the Science of Human History (MPI-SHH), GermanyReviewed by:
Sarah Pederzani, University of La Laguna, SpainAleksa K. Alaica, University of British Columbia, Canada
Copyright © 2023 Royer and Somerville. This is an open-access article distributed under the terms of the Creative Commons Attribution License (CC BY). The use, distribution or reproduction in other forums is permitted, provided the original author(s) and the copyright owner(s) are credited and that the original publication in this journal is cited, in accordance with accepted academic practice. No use, distribution or reproduction is permitted which does not comply with these terms.
*Correspondence: Andrew D. Somerville, YXNvbWVydmlAaWFzdGF0ZS5lZHU=