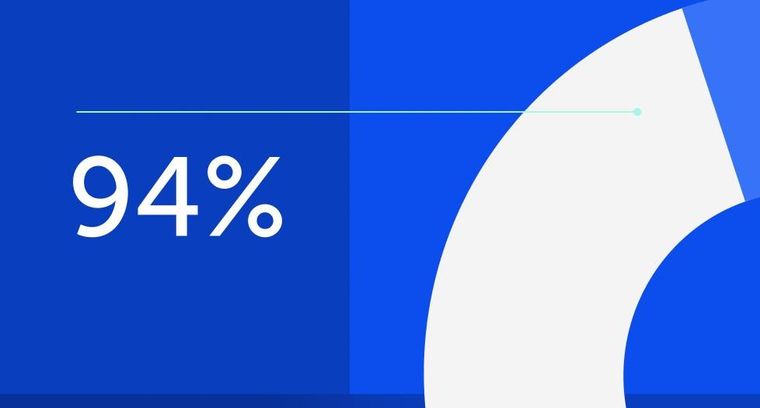
94% of researchers rate our articles as excellent or good
Learn more about the work of our research integrity team to safeguard the quality of each article we publish.
Find out more
ORIGINAL RESEARCH article
Front. Energy Res., 31 March 2025
Sec. Wind Energy
Volume 13 - 2025 | https://doi.org/10.3389/fenrg.2025.1569659
Introduction: In this paper, a dynamic structural response simulation program TwrDyn for wind turbine tower is developed based on geometrically exact beam theory model, and the program is verified by comparing with numerical simulation and experiment results.
Results and discussion: The results show that TwrDyn program can accurately predict the dynamic deformation and load response characteristics of large wind turbine tower under normal and extreme operation conditions, in which the prediction deviations of tower deformation and load response are less than 6.8% and 5.2%, respectively. The program developed in the present study can provide reliable prediction results for tower response characteristics.
To achieve the goal of cost-competitive offshore wind energy utilization, the large-scale development of offshore wind turbines has become an inevitable trend to reduce the levelized cost of electricity (LCOE) (Ma et al., 2024; Huang et al., 2024; Fang et al., 2024). Both the rotor diameter and tower height increase significantly with the capacity of the wind turbine. Under the effect of the aerodynamic loads and wave loads, the elastic deformation and vibration amplitude of the tower of offshore wind turbine are much greater than those of onshore wind turbines. Accurately evaluating the dynamic response characteristics of the tower is crucial for ensuring the structural stability and operational safety of offshore wind turbines, and it remains a key issue in the offshore wind energy research field (Zhou et al., 2024; Guo et al., 2024).
Currently, the tower accounts for approximately 20%–30% of the total cost of a wind turbine. Reasonable design of tower structures is one of the most effective ways to reduce costs, which largely depends on efficient and accurate calculations and simulations of external loads and structural responses. Compared to the wind turbine blades, the tower’s diameter and stiffness are larger, so the existing wind turbine structural simulation programs tend to focus more on the development of blade structural models. For example, the HAWC2 program at the Technical University of Denmark uses a Timoshenko beam model to calculate the deformation of wind turbine blades (Larsen and Hansen, 2007; Rinker et al., 2020), and the BeamDyn program developed by the National Renewable Energy Laboratory in the U.S. is based on geometrically exact beam theory for blade structural simulations (Wang et al., 2017). In terms of the wind turbine tower, most current integrated wind turbine load and structural analysis program uses the Euler-Bernoulli beam model. This beam model can account for nonlinear velocity-displacement effects under small deformations but is unable to accurately capture the geometrically nonlinear effects under large deformations. Flexible towers subjected to wind loads undergo significant deformation and nonlinear vibrations, leading to more complex aeroelastic issues in interaction with the inflow wind (Cheng et al., 2024a; Lin et al., 2024). Besides, the foundation of offshore wind turbines provides less constraint on the tower and is more sensitive to environmental loads. Under the combined effect of wind, waves, and currents, the tower structure undergoes considerable elastic deformation, which can lead to tower vibrations (Cheng et al., 2024b). For floating wind turbines, the aerodynamic yawing moment at the top of the tower and the wave-induced yawing moment on the floating platform are in opposite directions, causing significant torsional deformation of the tower (Cheng B. et al., 2024; Jiang et al., 2024).
Therefore, the dynamics of the flexible tower of large offshore wind turbine is quite complex, presenting challenges for design and analysis. In-depth research on the fundamental dynamics of wind turbine tower under large deformations and the development of corresponding dynamic calculation programs are of great significance for the structural optimization of wind turbines, reducing offshore wind farm development costs, and ensuring the long-term safe and stable operation of the turbines. To address these issues, China Three Gorges Corporation (CTG) has developed the TwrDyn (Tower Dynamics) dynamic structural response simulation program based on geometrically exact beam theory based on the key technology research and application project for offshore wind turbines. The program’s accuracy has been verified through comparisons with numerical simulation results and experimental tests.
This study employs geometrically exact beam theory to develop a simulation program for the structural response of large wind turbine towers. Based on Hamilton’s principle, the governing equations of the geometrically exact beam are established. For the wind turbine tower, the relationship between stress and strain at each cross section along the tower can be expressed as Equation 1:
where,
Therefore, the strain energy of the deformation of the tower can be expressed as Equation 2:
where, l is the length of the beam.
Moreover, the momentum and angular momentum at the cross section of the tower can be expressed as Equation 3:
where,
Based on Hamilton’s principle, the governing equation for the deformation of the tower can be expressed as Equation 5:
where,
According to the Bauchau (2011), the governing equation for the tower deformation can be expressed as Equation 6:
where,
Through linearization and discretization of Equation 11, and represent the physical quantities of the beam element in terms of the nodal quantities, the expression becomes Equation 11:
where,
This study uses the developed TwrDyn program to conduct numerical simulation for the NREL-5 MW wind turbine tower, and compare the prediction results from TwrDyn with those obtained from ANSYS software to verify the reliability of the TwrDyn. The NREL-5 MW wind turbine is a digital wind turbine developed by the U.S. National Renewable Energy Laboratory (NREL) and is widely used in wind turbine research (Jonkman et al., 2009). The basic parameters of the NREL-5 MW wind turbine is shown in Table 1. The tower of the NREL-5 MW wind turbine is a steel structure with a height of 90 m, and its stiffness distribution along the height is shown in Figure 1. The rated operational wind speed for the NREL-5 MW wind turbine is 11.4 m/s. In this study, simulations are conducted at wind speeds of 8, 11.4, and 22 m/s to assess the reliability of the TwrDyn under condition below, at, and above the rated wind speed.
To verify the accuracy of the TwrDyn program in calculating the dynamic response of tower structures, the NREL-5 MW wind turbine is adopted as the object, and the results calculated by the TwrDyn are compared with those obtained using commercial software ANSYS. The required load time-domain data for the simulation was generated using OpenFAST, and the generated load sequences were then applied to both the TwrDyn program and ANSYS software.
By using the OpenFAST, the normal force and tilt moment at the tower top of the NREL-5 MW wind turbine under turbulent wind condition at 8, 11.4, and 22 m/s are calculated, and the results are shown in Figure 2. Since the initial rotational speed of the rotor is set to 0, the thrust and moment at the tower top exhibit a noticeable gradual increase under all three wind conditions. Under lower wind speeds, the rate of increase is slower, while under higher wind speeds, the thrust quickly reaches its peak. As the wind speed exceeds the rated value under condition of 22 m/s, the rotational speed of the turbine quickly reaches its rated value, and activate the pitch control system. Consequently, aerodynamic forces begin to decrease and a significant jump in the tower top thrust is observed around 30 s under 22 m/s wind condition, which is due to the pitch and speed control effect of the controller, leading to a sudden change in aerodynamic forces.
The dynamic response of the tower structure under the three load conditions was calculated using both ANSYS software and the TwrDyn program developed in this project. Figure 3 presents the comparison of the tower top displacement results. From the figure, it can be observed that under all three conditions, the results obtained from the TwrDyn program closely match those from ANSYS, with very similar trends and amplitude fluctuations in the time domain. The only exception is for the 8 m/s wind speed condition, where the results from the TwrDyn program show slight fluctuations, as the tower top load in this model changes direction in response to the structural bending deformation. However, the results for all three conditions are generally consistent and closely aligned, indicating that the TwrDyn program developed in the present study can accurately predict the tower structural deformation.
Figure 3. Comparison of displacement of tower top under three wind conditions. (a) 8 m/s (b) 11.4 m/s (c) 22 m/s.
Figure 4 shows the distribution of the maximum tower deformation at different heights of the tower under the three wind conditions in the time-domain simulation, with the statistical range from 100 to 200 s. From the figure, it is clear that the results obtained from the TwrDyn program are in good agreement with those from ANSYS. As the tower height increases, the displacement at the tower top continuously increases, and the height distribution trend is similar to the first mode shape of the tower. The calculation results from the TwrDyn program in all three simulation wind conditions closely match the ANSYS results. The computational results of the TwrDyn program under three wind conditions are in good agreement with those obtained from ANSYS. Only the results for the 8 m/s wind speed condition are slightly higher, with the maximum displacement at the top of the tower reaching 0.28 m, compared to the ANSYS simulation result of 0.26 m, resulting in a difference of 6.8%. The discrepancies between the TwrDyn results and the ANSYS results for other conditions are all less than 1%.
Figure 4. Comparison of the distribution of the maximum time domain value of tower displacement with height under three wind conditions. (a) 8 m/s (b) 11.4 m/s. (c) 22 m/s.
Figure 5 presents the time-domain curves of the tower base moment calculated by both ANSYS and the TwrDyn program under the three wind conditions. Similar to the tower top displacement results, the results from the TwrDyn program are in good agreement with those from ANSYS in terms of the time-domain variation trend and the amplitude fluctuation range. The only exception is for the 8 m/s wind speed condition, where the results show little deviation in terms of fluctuation. However, the overall difference is minimal. This indicates that the TwrDyn program is highly reliable for load calculation of the tower loads.
Figure 5. Comparison of bending moment of the tower base under three wind conditions. (a) 8 m/s (b) 11.4 m/s. (c) 22 m/s.
Figure 6 shows the variation of the maximum tower moment with tower height under the three wind conditions. From the figure, it can be observed that the maximum tower moment exhibits an approximately linear relationship with the tower height. Both the results from the TwrDyn program and ANSYS demonstrate this trend. Similar to the displacement results, for the 8 m/s wind speed condition, the tower base moment calculated by ANSYS is slightly lower than the result from the TwrDyn program, with a difference of 5.2%. For the other two conditions, the results from both programs are almost identical, particularly in capturing the maximum tower moment, with differences below 2%, specifically 1.7% and 0.94%. This result indicates that the TwrDyn program can accurately predict the structural dynamic response of the tower under external loads.
Figure 6. Comparison of the distribution of the maximum time domain value of tower bending moments with height under three wind conditions. (a) 8 m/s (b) 11.4 m/s (c) 22 m/s.
To verify the accuracy of the TwrDyn program, an experiment is constructed, and the measured data are used for comparison with the TwrDyn program. For the NREL 5 MW wind turbine, a 1:50 scaling factor is applied, meaning that the key geometric parameters (diameter and height) of the experimental model are scaled by a factor of 1:50 relative to the full-scale model, as detailed in Table 2. It is important to note that the wall thickness of the prototype wind tower at its thinnest point is only 11 mm, and the tower diameter gradually decreases with height. If the geometrically similarity is strictly adhered to for scaling, it would result in a model with an unworkable wall thickness. Since the primary goal of this experiment is to validate the accuracy of the program, the differences between the experimental model and the reference prototype tower do not affect the calibration of the TwrDyn and ANSYS program as the model used in the TwrDyn program is identical to the scaled experimental tower. Figure 7 show the CAD model and the physical tower model used in the experiment.
Table 2. Comparison of main scale parameters between prototype and test scaling model of wind turbine tower.
The experimental setup is as shown in Figure 8. In this experiment, tower deformation is achieved using a cable-pulling mechanism. One end of the cable is connected to the nacelle, while the other end is attached to a balancing weight. The position of the balancing weight is adjusted using an excitation actuator and a lifting platform. The response of actuator is programmatically controlled to apply various types of excitations.
This experiment measures the vibration acceleration response, displacement response, and force response of the tower. Accordingly, the following instrumentation is used:
1) Acceleration sensors: Ten sensors are placed on the front side of the tower at different heights from top to bottom, positioned at 180, 170, 160, 140, 120, 100, 80, 60, 40, and 20 cm above the ground.
2) Displacement sensor: One sensor is installed on the tower top.
3) Force sensor: One sensor is placed at the connection of the cable and nacelle.
4) Strain gauges: Five sets of strain gauges are attached to the front and back sides of the tower at five different heights.
This configuration ensures comprehensive data acquisition for acceleration, displacement, and force responses to evaluate the tower’s dynamic characteristics under varying excitation conditions.
By applying a sinusoidal wave with a period of 10 s and an amplitude of 20 N to the balancing weight using the actuator, the tower top experienced an average tensile force of 48 N. The tensile force measured by the force sensor is shown in Figure 9. As observed in the figure, the force measured by the sensor is generally consistent with the target applied load. However, due to factors such as the precision of the experimental equipment and environmental noise, there are slight fluctuations in the tension measured by the sensor. Nonetheless, the overall trend is quite consistent, and it can effectively represent the characteristics of the sinusoidal external force.
The comparison of the measured tower top displacement and tower base bending moment under dynamic load conditions with the results calculated by the TwrDyn program is shown in Figure 10. The input load for the simulation is the theoretical sinusoidal thrust force, without considering the effect of tower top bending moments. As shown in the figure, the phase and oscillation period of the calculated results from this project align perfectly with the experimental test results. Due to input excitation and measurement signal noise, slight differences are observed between the experimental and numerical results. However, these differences are within an acceptable range. This outcome demonstrates that the TwrDyn program has highly reliable accuracy in predicting the structural dynamic response of the tower under dynamic loading conditions.
Figure 10. Comparison of the results under dynamic load conditions. (a) Tower top displacement. (b)Tower base bending moment.
Based on the geometrically exact beam theory, a dynamic structural response simulation program for wind turbine towers, TwrDyn, is developed and its simulation results were compared with those from ANSYS software and experimental data. Regarding blade deformation, the comparison results of tower deformation responses at different wind speeds between the TwrDyn program and ANSYS software are in close agreement. The variation trends and amplitude fluctuations in the time domain are very similar, with the maximum deviation in predicted node deformations under turbulent wind conditions between the TwrDyn and ANSYS results being 6.8%. In terms of tower loads, the time-domain responses of the tower base load at different wind speeds predicted by the TwrDyn program are in good agreement with the results from ANSYS software. The maximum deviation in predicted node bending moments under turbulent wind conditions is 5.2%. In conclusion, the program developed in this study can accurately predict the deformation and load characteristics of the tower under various conditions.
The original contributions presented in the study are included in the article/supplementary material, further inquiries can be directed to the corresponding author.
LM: Conceptualization, Data curation, Methodology, Project administration, Software, Supervision, Validation, Writing–original draft, Writing–review and editing. DY: Investigation, Methodology, Writing–review and editing. HG: Conceptualization, Software, Writing–review and editing. XS: Funding acquisition, Project administration, Resources, Writing–review and editing, Writing–original draft. LZ: Writing–original draft, Writing–review and editing.
The author(s) declare that financial support was received for the research and/or publication of this article. The present study is supported by China Three Gorges Corporation (202303058).
The authors thank the editors and reviewers for their comments on this paper.
Authors ML, YD, and GH were employed by China Three Gorges Corporation.
The remaining authors declare that the research was conducted in the absence of any commercial or financial relationships that could be construed as a potential conflict of interest.
The authors declare that this study received funding from China Three Gorges Corporation. The funder had the following involvement in the study: Project administration, Resources.
The author(s) declare that no Generative AI was used in the creation of this manuscript.
All claims expressed in this article are solely those of the authors and do not necessarily represent those of their affiliated organizations, or those of the publisher, the editors and the reviewers. Any product that may be evaluated in this article, or claim that may be made by its manufacturer, is not guaranteed or endorsed by the publisher.
Cheng, B., Yao, Y., Qu, X., Zhou, Z., Wei, J., Liang, E., et al. (2024c). Multi-objective parameter optimization of large-scale offshore wind Turbine's tower based on data-driven model with deep learning and machine learning methods. Energy 305, 132257. doi:10.1016/j.energy.2024.132257
Cheng, Y., Cao, L., Liu, J., Wang, Y., and Zhou, X. (2024b). Intelligent analysis of dynamic characteristics of steel-concrete hybrid wind turbine tower based on adaptive vibration mode. Structures 68, 107235. doi:10.1016/j.istruc.2024.107235
Cheng, Y., Zhao, Y., Qi, H., and Zhou, X. (2024a). Intelligent optimal design of steel-concrete hybrid wind turbine tower based on evolutionary algorithm. J. Constr. Steel Res. 218, 108729. doi:10.1016/j.jcsr.2024.108729
Fang, H., Lin, S., Zhu, J., and Lu, W. (2024). Application of deep forest algorithm incorporating seasonality and temporal correlation for wind speed prediction in offshore wind farm. Front. Energy Res. 12. doi:10.3389/fenrg.2024.1488718
Guo, J., Liu, M., Fang, Z., Chen, W., Pan, X., and Xiao, L. (2024). Tower loads characteristics of a semi-submersible floating wind turbine: an experimental study. Ocean. Eng. 311, 118967. doi:10.1016/j.oceaneng.2024.118967
Huang, J., Xu, H., Chen, L., Lin, K., Guo, M., Yang, M., et al. (2024). Analysis of mooring performance and layout parameters of multi-segment mooring system for a 15 MW floating wind turbine. Front. Energy Res. 12. doi:10.3389/fenrg.2024.1502684
Jiang, T., Lv, P., and Li, D. (2024). A new shape reconstruction method for monitoring the large deformations of offshore wind turbine towers. Ocean. Eng. 312, 119253. doi:10.1016/j.oceaneng.2024.119253
Jonkman, J., Butterfield, S., Musial, W., and Scott, G. (2009). Definition of a 5-MW reference wind turbine for offshore system development. Golden, CO: United States: National Renewable Energy Lab.
Lin, S., Zhang, B., Zhang, S., Yang, X., and Peng, Y. (2024). Dynamic responses of thin-walled FRP-concrete-steel tubular wind turbine tower under horizontal impact loading: experimental study and FE modelling. Structures 69, 107383. doi:10.1016/j.istruc.2024.107383
Ma, L., Ding, J., Zhang, X., Wang, W., Zhao, X., Sun, C., et al. (2024). Analysis of dynamic response of offshore wind turbines subjected to ship impacts and the corresponding protection measures: a review. Front. Energy Res. 12. doi:10.3389/fenrg.2024.1497210
Rinker, J., Gaertner, E., Zahle, F., Skrzypiński, W., Abbas, N., Bredmose, H., et al. (2020). Comparison of loads from HAWC2 and OpenFAST for the IEA wind 15 MW reference wind turbine. J. Phys. Conf. Ser. 1618 (5), 052052. doi:10.1088/1742-6596/1618/5/052052
Wang, Q., Sprague, M. A., Jonkman, J., Johnson, N., and Jonkman, B. (2017). BeamDyn: a high-fidelity wind turbine blade solver in the FAST modular framework. Wind Energy 20 (8), 1439–1462. doi:10.1002/we.2101
Keywords: wind turbine tower, geometrically exact beam theory, structural response, numerical simulation, experiment
Citation: Ma L, Yang D, Guo H, Shen X and Zhou L (2025) Development and verification of dynamic structural model of wind turbine tower based on the geometrically exact beam theory. Front. Energy Res. 13:1569659. doi: 10.3389/fenrg.2025.1569659
Received: 01 February 2025; Accepted: 13 March 2025;
Published: 31 March 2025.
Edited by:
Wen Zhong Shen, Yangzhou University, ChinaReviewed by:
Bofeng Xu, Hohai University, ChinaCopyright © 2025 Ma, Yang, Guo, Shen, and Zhou. This is an open-access article distributed under the terms of the Creative Commons Attribution License (CC BY). The use, distribution or reproduction in other forums is permitted, provided the original author(s) and the copyright owner(s) are credited and that the original publication in this journal is cited, in accordance with accepted academic practice. No use, distribution or reproduction is permitted which does not comply with these terms.
*Correspondence: Xin Shen, c2hlbnhpbkBzanR1LmVkdS5jbg==
Disclaimer: All claims expressed in this article are solely those of the authors and do not necessarily represent those of their affiliated organizations, or those of the publisher, the editors and the reviewers. Any product that may be evaluated in this article or claim that may be made by its manufacturer is not guaranteed or endorsed by the publisher.
Research integrity at Frontiers
Learn more about the work of our research integrity team to safeguard the quality of each article we publish.