- Department Engineering, University of IMAM Ali, Tehran, Iran
Nanotechnology has arisen as a revolutionary technology, providing a diverse range of solutions to tackle energy-related difficulties. Nanotechnology allows for the creation of components and devices that are smaller than 100 nm, which in turn provides new opportunities for improving the efficiency of energy capture, storage, and transport. Through the process of nuclear fusion, the sun produces a vast quantity of energy on a daily basis, surpassing all the energy that humanity has ever harnessed throughout history. The worldwide technical capacity of solar energy significantly surpasses the current overall primary energy requirement. This review explores the role of nanomaterials in improving solar energy harvesting systems, including solar collectors, fuel cells, photocatalytic systems, and photovoltaic cells. Through a systematic review of peer-reviewed studies, key findings indicate that nanomaterials can enhance incident solar radiation absorption by up to nine times, leading to a 10% efficiency improvement in solar collectors compared to conventional designs. Additionally, advancements in third-generation solar cells demonstrate the potential of nanostructured materials in enhancing charge transport, light absorption, and cost-effectiveness. The study further highlights existing challenges, such as the long-term stability of nanomaterials, environmental concerns, and economic barriers to large-scale implementation. Addressing these limitations through sustainable nanomaterial design and scalable production techniques will be essential for realizing the full potential of nanotechnology in solar energy applications.
1 Introduction
The scientific community has been fascinated by nanotechnology ever since Nobel laureate Richard Feynman delivered his influential speech in 1959, titled “There’s plenty of room at the bottom,” in which he emphasized the immense possibilities of nanoscale study (Gribbin and Gribbin, 2002). Feynman envisioned a future in which the manipulation of individual atoms and molecules could be achieved by the use of increasingly smaller tools, ultimately allowing for perfect control at the atomic scale. In 1974, Norio Taniguchi provided a formal definition of nanotechnology as the deliberate manipulation of materials at the atomic or molecular level (Taniguchi, 1974). This definition has since been expanded to include structures that are up to 100 nm in size, which is referred to as the nanoscale. At the nanoscale, which spans from 1 to 100 nm (1 billionth to several tens of billionths of a meter), materials display distinct physical, chemical, and biological characteristics that differ considerably from those of bulk materials or individual atoms. The unique characteristics of these materials have resulted in the creation of tailor-made gadgets that possess capabilities that are absent in larger-scale materials or even in natural substances. In addition, nanotechnology has advanced to incorporate structures that exhibit quantum mechanical phenomena, such as quantum dots. This broadens its definition to highlight not only the reduction in size but also the precise control of atoms and molecules to design specific characteristics in nanomaterials and nanosystems. In the 1980s, Dr. K. Eric Drexler delved more into the concept of nanotechnology as a deterministic method for manipulating atoms and molecules. He established the notion of “Molecular Nanotechnology” (MNT) or “molecular manufacturing.” This perspective signifies a notable deviation from stochastic processes, with a specific emphasis on the intentional and meticulous fabrication of materials at the molecular scale (Aali et al., 2023; Kazemi et al., 2024; Tashakori-Asfestani et al., 2023; Kazemi et al., 2025). In 1974, Dr. Tuomo Suntola and his colleagues in Finland invented and patented the atomic layer deposition process. This approach enables the precise deposition of homogeneous thin films, one atomic layer at a time, which has significantly contributed to the progress of nanotechnology (Abdin et al., 2013).
Nanotechnology offers innovative instruments that can propel the advancement of emerging sectors, promoting economically viable and streamlined economies that make a substantial contribution to sustainable economic expansion. Nanotechnology has the potential to significantly decrease the environmental effects of energy generation, storage, and usage in the energy industry. While achieving a completely sustainable energy system is still a long-term objective, the scientific community is currently prioritizing the development of energy nanotechnologies. Energy experts predict that the worldwide energy demand will exceed 30 TW (TW) by 2050 (Serrano et al., 2009). Many experts believe that the only way to meet this issue is by utilizing solar energy. Solar power is considered a crucial form of renewable energy, provided that the production costs of solar cells are decreased to enable them to compete with other energy sources. Parida et al. (Parida et al., 2011) conducted a comprehensive analysis of solar cell technologies, highlighting the importance of developing innovative methods to improve the efficiency of solar energy collection in order to fulfill the increasing worldwide need for sustainable energy. Solar cell technology has advanced through three generations, each exhibiting unique properties (Werner, 2012; Ismail et al., 2024; Anika et al., 2024). The initial iteration depends on semiconductor wafers made of a single crystal structure, which provide excellent efficiency. Specifically, single junction crystalline solar cells can achieve efficiency levels of up to 27%. According to theoretical models rooted on Shockley-Queisser thermodynamics, the highest possible efficiency of individual junction cells is estimated to be around 33% (Shockley and Queisser, 2006). The second generation of solar cells use inorganic thin film structures, which are more economically viable to manufacture but exhibit lower efficiency. Amorphous thin film cells, in particular, often achieve less than 14% efficiency. In order to address the efficiency constraints of previous technologies, a novel method has been devised, known as third-generation solar cells (Green, 2000). These advanced cells are engineered to exceed the efficiency barrier of 33% and simultaneously decrease production costs. This makes them a potentially revolutionary technology for the future of solar energy. The interplay between the production costs of solar cells, the efficiency of the modules, and the cost per unit of power is crucial in this progression, as demonstrated in relevant research (Figure 1) (Frankl et al., 2010; Green, 2006; Souri and Mojra, 2021; Abbasi and Souri, 2021; Souri et al., 2021; Jana et al., 2024).
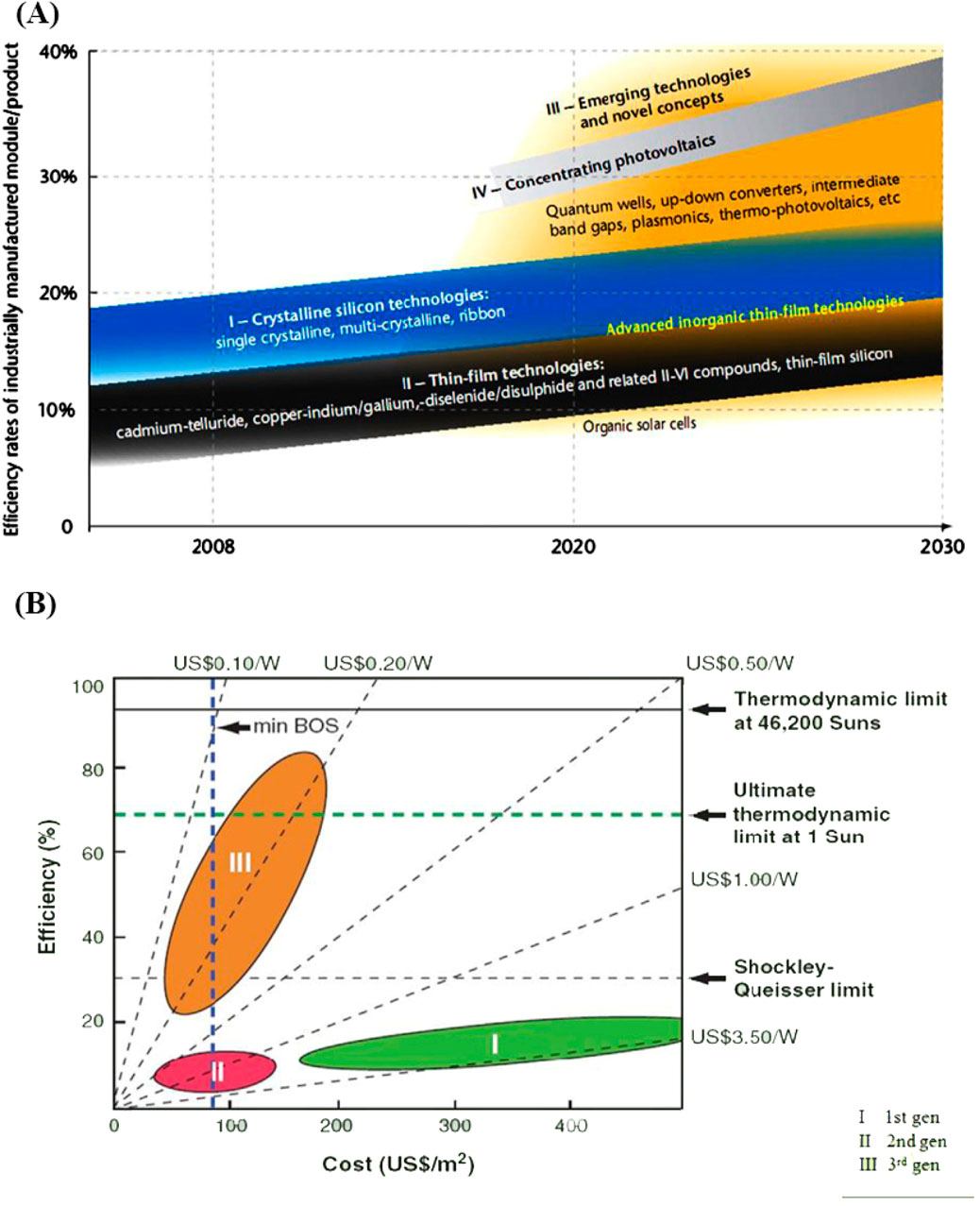
Figure 1. (A) Current advancements and future outlook of solar cell technology (Frankl et al., 2010), (B) The balance between efficiency and cost across the three generations of solar cell technology (Green, 2006).
This paper first examines the varied applications of nanotechnology in different solar energy systems. Moreover, it underscores the vital importance of nanotechnology in improving the efficacy of solar cell technologies. Comprehensive study indicates that nanotechnology has significantly advanced the utilization of solar radiation for sustainable energy generation through numerous methods. This paper aims to examine the role of nanotechnology in enhancing solar energy, specifically in addressing its current constraints. The review employs a defined process to guarantee a thorough and methodical investigation. Relevant papers were chosen from peer-reviewed journals, conference proceedings, and credible sources, concentrating on breakthroughs in nanotechnology applications for solar energy. The analysis of data was conducted using essential performance measures, including efficiency enhancements, material characteristics, and economic feasibility. This study seeks to synthesize data to offer a comprehensive knowledge of prevailing trends, difficulties, and future directions in the subject.
2 Utilizing nanotechnology for solar energy harvesting
Prior studies have shown that nanotechnology is a potent instrument for augmenting the efficiency, sustainability, and efficacy of solar energy systems. This is apparent in various crucial domains: (i) It allows for precise control of how light interacts with materials, making it easier to incorporate inexpensive semiconductors into devices such as solar panels; (ii) It helps in the development of more efficient photocatalysts, which are essential for converting sunlight into chemical fuels; (iii) It assists in the creation of new materials and membranes needed for various energy-related separations; (iv) It improves the conversion of chemical fuels into electrical energy and vice versa, resulting in higher energy and power densities in batteries; and (v) It increases efficiency in a wide range of applications, including displays, solid-state lighting, thermoelectric devices, and reducing friction.
Nanotechnology has been applied across different solar technologies, such as active solar systems and passive solar systems (Table 1). The following subsections highlight notable advancements in these areas in recent years.
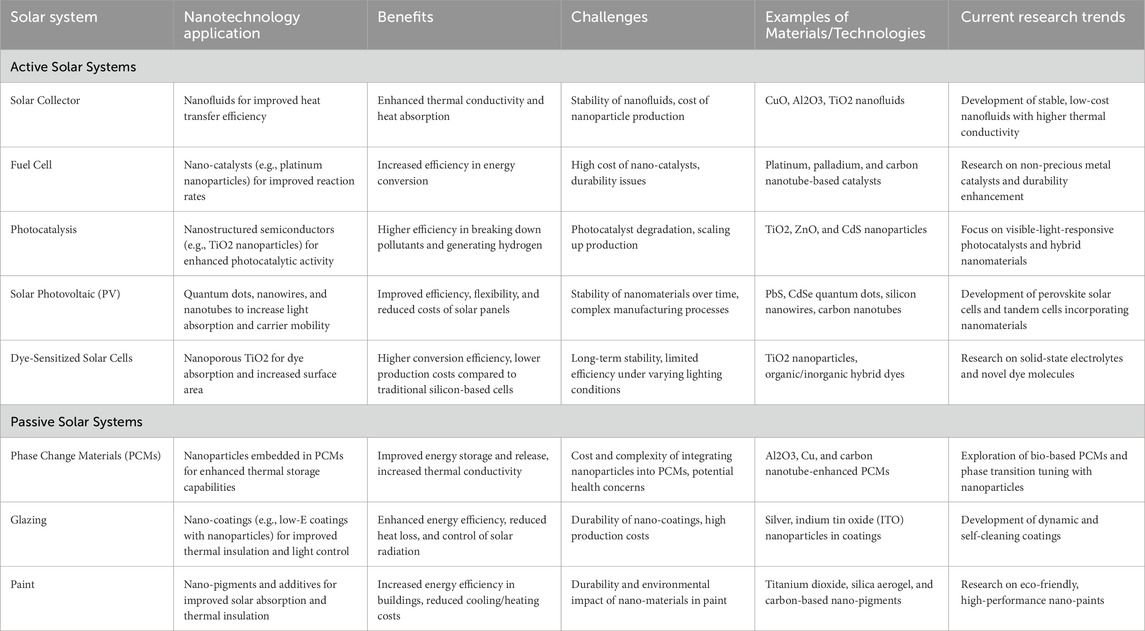
Table 1. A comprehensive table outlining the use of nanotechnology in various solar energy harvesting systems, both active and passive.
2.1 Active solar systems
Active solar systems are designed to convert solar energy into more practical forms, such as heat or electricity. This energy can be utilized within a building for heating, cooling, or lowering energy consumption and costs. These systems depend on electrical or mechanical equipment to enable the conversion of energy. Active solar systems have a key benefit in their capacity to utilize controls, typically electrical, to maximize their performance. The next sections address several active solar systems, such as solar collectors, fuel cells, photocatalysis, solar photovoltaics, and dye-sensitized solar cells.
2.1.1 Solar collector
Solar collectors are a type of heat exchanger that harness solar energy through an absorber, typically composed of a solid material. As the absorber’s internal energy grows, its temperature elevates, and heat is then transmitted to a fluid through conduction. Significant study has been carried out in recent years to investigate the characteristics and uses of flat-plate solar collectors (Rojas et al., 2008; Villar et al., 2009; Tang et al., 2011). Researchers have suggested multiple approaches to improve the efficiency and output temperature. One highly effective method is to substitute the conventional absorbing substance, water, with nanofluids, which are fluids that include solid nanoparticles in suspension. The utilization of nanofluids in solar collectors and solar water heaters has become widespread due to their high efficiency, cost-effectiveness, and environmental benefits (Choi and Eastman, 2001). For instance, Khullar et al. (Khullar and Tyagi, 2010) conducted an analysis on a linear parabolic solar collector that utilized nanofluid as the medium for heat transmission. The researchers represented the collection as a stable system in two dimensions and using the finite difference approach to numerically solve the equations. Their investigation demonstrated that the nanofluid-based collector surpassed conventional collectors in performance under comparable conditions. In a similar manner, Taylor et al. (Taylor et al., 2011) conducted a study on a concentrating solar thermal system that utilized nanofluid. They discovered that replacing water with nanofluid has the potential to enhance efficiency by 10%. Their findings indicate that the utilization of graphite/Therminol VP-1 nanofluids with extremely low volume fractions (about 0.001% or lower) could provide notable benefits for power plants in the range of 10–100 MWe.
Tyagi et al. (2009) conducted a theoretical study on a direct absorption solar collector (DASC) utilizing nanofluids at low temperatures. They analyzed the impact of various factors on the collector’s efficiency, using a mixture of water and aluminum nanoparticles as the working fluid. The inlet temperature of the fluid, solar flux on the collector, and mass flow rate were set at 35°C, 1000 W/m2, and 1.2 kg/s, respectively. Their research revealed a notable enhancement in efficiency when nanoparticles were introduced, especially when present in small amounts. Nevertheless, as the volume percentage surpassed 2%, the efficiency reached a plateau, indicating that more nanoparticle additions would not yield any further advantages. He and his colleagues (He et al., 2011) investigated the light-to-heat conversion properties of water-based carbon nanotube (CNT) and TiO2 nanofluids under different weather settings, including bright and cloudy conditions. Their findings demonstrated that a nanoparticle weight concentration of 0.5% in CNT-H2O nanofluid had exceptional efficiency in converting light into heat. Meanwhile, Otanicar et al. (2010) did a comprehensive investigation on a microscale direct absorption solar collector (DASC) using both numerical simulations and practical tests. They assessed the collector’s effectiveness by testing several nanofluids, such as carbon nanotubes, graphite, and silver, and compared the findings to those obtained from a traditional collector. Their investigations demonstrated that the incorporation of minute quantities of nanoparticles, approximately 0.5%, resulted in notable enhancements in efficiency. Phelan et al. (Khullar et al., 2012) compared the theoretical and experimental results of a nanofluid-based concentrating parabolic solar collector (NCPSC) with those of traditional parabolic solar collectors. The comparison was done under similar conditions. The base fluid used was Therminol VP-1, which contained 0.05 volume percent of aluminum nanoparticles. According to their calculations, the NCPSC has the potential to deliver a 5%–10% enhancement in thermal efficiency when compared to conventional parabolic solar collectors.
Yousefi et al. (2012) conducted an experimental study to evaluate the impact of Al2O3/water nanofluid on the performance of a flat-plate solar collector. The experiments were carried out using two different weight fractions of the nanofluid, 0.2% and 0.4%, with nanoparticles measuring 15 nm in diameter. In addition, they evaluated the impact of Triton X-100, employed as a surfactant, on the efficacy of the system. Their research uncovered the subsequent results: (i) The use of a nanofluid with a weight fraction of 0.2% resulted in a 28.3% increase in efficiency compared to using water alone. (ii) Over a broad range of reduced temperature parameters, the collector utilizing the 0.2% nanofluid demonstrated greater efficiency compared to the one with the 0.4% nanofluid. (iii) Efficiency was improved by 15.63% with the addition of a surfactant.
Liu et al. (2013) developed an innovative evacuated tubular solar air collector designed to supply air at both high and moderate temperatures. This device was integrated with a simplified compound parabolic concentrator and featured a unique open thermosyphon utilizing a water-based CuO nanofluid as the working fluid. Experimental results showed that using nanofluid in the thermosyphon enhanced the air outlet temperature and overall efficiency of the solar air collector compared to using water alone.
2.1.2 Photocatalysis
Photocatalysis has become a subject of great interest among researchers in recent years, as it has the potential to effectively decrease environmental pollution and conserve energy. Figure 2A showcases a range of applications where TiO2 photocatalysis is utilized. Photocatalytic materials are presently employed to transform solar energy into chemical energy, enabling the oxidation or reduction of molecules to generate useful chemicals like hydrogen and hydrocarbons. Moreover, these materials exhibit efficacy in eliminating contaminants and microorganisms from various surfaces (Zhang et al., 2008). Titanium dioxide (TiO2) nanoparticles are extensively utilized in various applications because of their potent oxidizing properties, which facilitate the breakdown of organic contaminants. Additional benefits encompass superhydrophilicity, chemical inertness, exceptional longevity, absence of toxicity, affordability, and transparency to visible light. TiO2 exhibits photocatalytic capabilities due to the creation of charge carriers (electrons and holes) when it absorbs ultraviolet (UV) light, which is associated with the material’s band gap. Figure 2B depicts the series of events that take place on uncoated TiO2 particles after being stimulated by UV light.
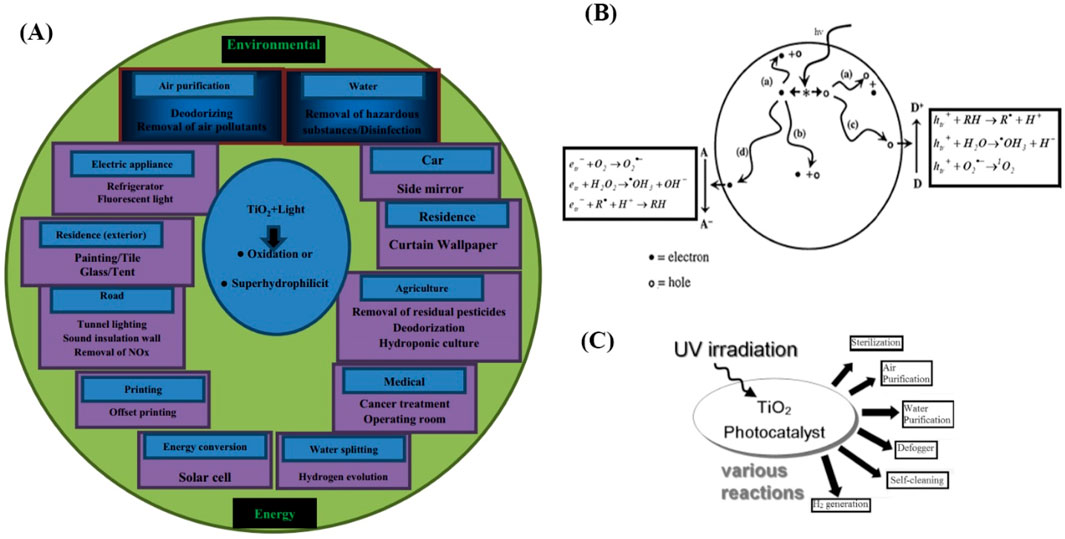
Figure 2. (A) Uses of TiO2 photocatalysis (Nakata and Fujishima, 2012), (B) Processes on bare TiO2 particles following UV excitation (Nakata and Fujishima, 2012), (C) Diagrammatic representation of photocatalysis applications (Ochiai and Fujishima, 2012).
Nanostructured TiO2 materials are widely employed in several applications, including photocatalysis, dye-sensitized solar cells (DSSCs), lithium-ion batteries, and electrochromic displays (Nakata and Fujishima, 2012; Bavykin et al., 2006). Titanium dioxide (TiO2) nanosheets are flat, two-dimensional structures that have high aspect ratios, low turbidity, and strong adherence to substrates. These nanosheets are especially efficient in applications like self-cleaning glass. This distinctive configuration presents potential advantages for filtration, segregation, and retention procedures (Nakata and Fujishima, 2012). The identification of these characteristics has greatly broadened the scope of uses for TiO2 coatings. Figure 2C presents a diagram that visually explains several uses of photocatalysis.
2.1.3 Solar photovoltaic
Solar photovoltaic/thermal (PV/T) technology is an effective method for simultaneously converting solar energy into both heat and electricity. This hybrid system captures part of the sunlight to generate electricity, while the remaining portion is converted into thermal energy (Tripanagnostopoulos, 2007; Chow et al., 2012). In a PV/T system, most of the solar energy is either absorbed by the solar cell or reflected. The incident radiation raises the temperature of the solar cell, resulting in consequences such as heightened reverse saturation current, diminished open-circuit voltage, and a decline in the energy gap (Cui and Zhu, 2012). Therefore, ongoing research is mostly concentrated on the cooling of solar cells and the retrieval of the produced heat. Figure 3 depicts a PV/T system that is decoupled. A review of the literature reveals extensive research on the use of nanofluids for cooling various thermal systems, including electronic devices (Taylor et al., 2012; Nguyen et al., 2007), vehicle radiators (Peyghambarzadeh et al., 2011), and microchannel heat sinks (Hung and Yan, 2012). The promising potential of nanofluids in cooling PV/T systems is attributed to their increased thermal conductivities. Table 2 compares various parameters between the PV/T system and a standard PV module. While the PV module boasts higher electrical efficiency, the overall efficiency of PV/T systems is significantly better, often exceeding 60% with a 2 mm thick liquid layer, due to the additional heat generated (Cui and Zhu, 2012). Xie (2013) studied the efficiency of a hybrid photovoltaic device incorporating a P3HT/CdS/CdSe/TiO2 nanorod array. Their findings showed that CdS/CdSe quantum dots are crucial in improving charge separation and injection. The researchers explained that by coating TiO2 nanorods with a layer of CdS/CdSe quantum dots and pairing them with P3HT to form p-n heterojunctions, they significantly enhanced the photovoltaic performance of the P3HT/CdSe/CdS/TiO2 nanorod solar cells. This improvement led to an energy conversion efficiency of 0.57%.
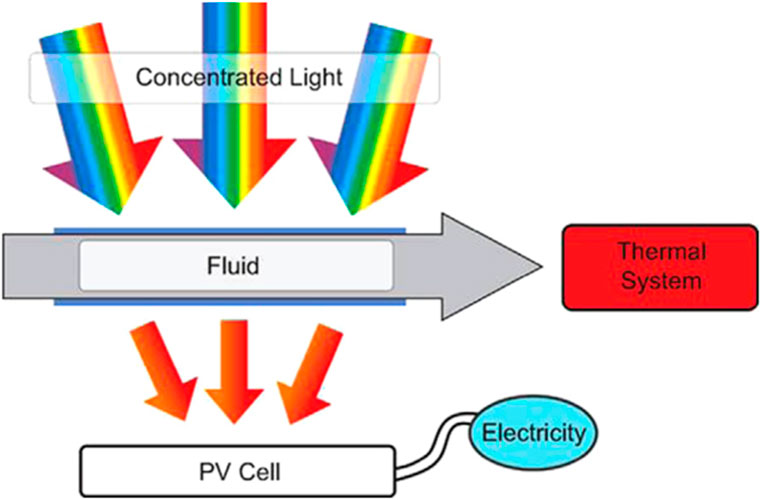
Figure 3. Diagram of the separated PV/T system (photovoltaic/thermal) (Xie, 2013).
2.1.4 Dye-sensitized solar cells
A dye-sensitized solar cell (DSSC) is a semiconductor photovoltaic device that directly converts solar energy into electrical current. The efficiency of a DSSC in converting visible light to electricity is influenced by various factors, including the sensitization of wide bandgap semiconductors, the photoelectrode, the redox electrolyte, and the counter electrode. Over the years, DSSC mechanisms have undergone various enhancements to improve cell efficiency. Chang et al. (2011) introduced photo-electrochemical parameters, as shown in Table 3, based on output data under simulated solar radiation. Their findings indicate a conversion efficiency of 4.83% and a TEM efficiency of 1.48% for the DSSC. Moreover, their experimental results showed that applying a CuO coating on the TEG surface can boost solar energy conversion efficiency and improve heat conduction. Recently, Wang et al. (2004) introduced an innovative TiO2 mesoporous network with a multilayer structure. In their research, they fabricated multilayer films composed of particles with diameters ranging from about 100 nm to 23 nm and layered them onto conducting glass to enhance light scattering. This design resulted in a 10.2% improvement in cell efficiency. Typically, TiO2 nanofluid is synthesized using an arc discharge nanofluid synthesis system. The nanofluid then undergoes electrophoresis deposition, where TiO2 nanoparticles are deposited onto indium tin oxide (ITO) conductive glass at room temperature, forming a thin film of TiO2 (Chang et al., 2010). In this process, the nanoparticles have diameters ranging from approximately 20–50 nm, and the nanofluid’s surface energy zeta potential is between −22 mV and −29 mV (Chen et al., 2010). Adachi et al. (Adachi et al., 2004) developed a network structure of single-crystal-like TiO2 nanowires using surfactant-assisted methods at a low temperature (353K). By adjusting the adsorption of surfactant molecules on the TiO2 surface, they were able to control the direction of crystal growth. Their experiment demonstrated that this surface achieved ruthenium dye adsorption that was four times higher than that of the commercial product P-25, leading to an overall cell efficiency of 9.3%.
2.2 Passive solar systems
Passive solar systems or building design involve the strategic arrangement of windows, walls, and floors to efficiently capture, retain, and distribute solar energy as heat in winter, while minimizing the absorption of solar heat in summer. This approach, known as passive solar design or climatic design, differs from active solar heating systems as it does not rely on mechanical or electrical components. The effectiveness of a passive solar structure relies on skillfully utilizing the specific characteristics of the local climate. Important aspects to take into account are the positioning and characteristics of windows, the utilization of insulating materials, thermal mass, and suitable coverings. Passive solar approaches can be readily incorporated into new construction, but older structures can also be adapted to include these elements. The following subsection provides a brief overview of the role of nanotechnology in passive solar systems.
2.2.1 Phase change materials (PCMs)
Phase Change Materials (PCMs) have a high heat capacity within a small temperature range, making them effective as heat reservoirs that maintain practically constant temperature. As temperatures increase, phase change materials (PCMs) undergo a shift from a solid state to a liquid state. This transition is accompanied by the absorption of heat, as it is an endothermic process. On the other hand, when temperatures decrease, the substance changes from a liquid state to a solid state, and this process releases heat through an exothermic reaction. Although the idea of utilizing phase change materials (PCMs) is easy to understand, precisely evaluating their impact on enhancing a building’s overall energy efficiency through latent heat storage remains an intricate task. Phase change materials (PCMs) are divided into three main categories: organic, inorganic, and eutectic. Organic PCMs are further classified into two primary groups: paraffins and non-paraffins. The non-paraffin group includes a range of organic molecules such as fatty acids, esters, alcohols, and glycols. Of these, fatty acids are of specific significance and are categorized into six subcategories: caprylic, capric, lauric, myristic, palmitic, and stearic acids. Inorganic phase change materials (PCMs) consist mostly of hydrated salts and metals. However, metals typically have melting values that are too high for use in passive construction applications. Eutectic phase change materials (PCMs) are composed of two or more components that have the lowest melting point when combined. When these components crystallize, they melt and freeze at the same temperature, resulting in a mixture of component crystals (Sharma et al., 2009). The eutectic phase change materials (PCMs) can be categorized into three types: organic-organic, organic-inorganic, and inorganic-inorganic. The successful incorporation of wallboards impregnated with phase change material (PCM) into the interior surfaces of building enclosures has attracted interest due to its capacity to preserve favorable indoor thermal conditions. Shilei et al. (Shilei et al., 2006) conducted a comparative research between a standard room and a room equipped with wallboards augmented with phase change material (PCM). The PCM utilized was a blend of capric acid and lauric acid in an 82:18 proportion, exhibiting freezing and melting values of 19.138°C and 20.394°C, correspondingly. The investigation revealed that the room equipped with wallboards infused with phase change material (PCM) had exceptional effectiveness in preserving warmth and ensuring thermal comfort in winter. This resulted in a substantial reduction in heat loss and a decrease in energy consumption from electrical heating systems. Ceron et al. (Cerón et al., 2011) have created and obtained a patent for tiles that utilize PCM (Phase Change Material) to regulate interior air temperatures in winter. These tiles has the ability to capture thermal energy from sunlight during the daytime and retain it for later usage as a source of heat during the nighttime. The investigation revealed that by modifying the fusion temperature, these tiles could function as a heat sink during hot summer days, so aiding in energy saving in buildings through passive cooling and heating. Zhu et al. (Zhu et al., 2011) developed a mathematical model for a shape-stabilized phase change material (SSPCM) wall system. They conducted a detailed analysis of its energy efficiency and determined the most effective control strategies for a real-time building air conditioning system that utilizes this SSPCM envelope. They investigated methods of reducing peak thermal load and controlling demand by utilizing the thermal features of the SSPCM. Simulations conducted in tropical climates like Hong Kong and Beijing showed that buildings with SSPCM envelopes exhibited enhanced thermal efficiency and indoor comfort, particularly under time-based and energy-plus-demand-based pricing models. Zhang et al. (Zhang et al., 2011) evaluated the thermal performance of brick walls embedded with phase change materials (PCMs) under varying external temperatures, using a thermal conduction model that incorporated phase change through the enthalpy-porosity method. Their findings indicated that PCM-filled bricks provide superior thermal storage capacity, enhanced thermal insulation, temperature management, and increased comfort for occupants, as compared to ordinary brick walls. Due to the increasing demand for housing in rising economies and the requirement for thermal improvements in existing structures in industrialized nations, there is a substantial opportunity for the advancement of innovative construction solutions that include Phase Change Materials (PCMs).
2.2.2 Glazing
Vanadium dioxide is highly promising as an intelligent glazing material due to its metal-to-semiconductor transition (MST) properties (Saeli et al., 2010). This transition involves a shift from a high-temperature rutile phase to a low-temperature monoclinic phase, leading to significant changes in its electrical conductivity and optical characteristics. In the monoclinic phase, vanadium dioxide acts as a semiconductor and is typically transparent to solar radiation. In contrast, in the rutile phase, it acts as a metal, reflecting solar radiation. Recent studies have aimed at lowering the transition temperature of vanadium dioxide by incorporating dopants, with tungsten proving to be the most effective, reducing the MST by 20°C–25°C per atomic percent (Manning et al., 2004). Saeli et al. (Saeli et al., 2010) investigated the incorporation of gold nanoparticles to improve the properties of vanadium dioxide films. Gold nanoparticles were chosen for their strong absorption, driven by surface plasmon resonance, which is affected by the dielectric properties of the surrounding environment. The study concluded that the properties of the resulting films could be precisely tailored by carefully selecting the shape and size of the nanoparticles. However, they also observed that while thermochromic films could be beneficial in warmer climates, they might not be ideal for cooler regions. Presting and König (Presting and König, 2003) extensively discussed the applications of nanotechnology in automotive glazing. They emphasized innovations such as antireflection coatings composed of multiple nanolayers on glass and sun-protective glazing that integrates infrared-reflecting nanolayers within glass panels. Additionally, they mentioned the use of thermoplastic nanocomposites, such as those marketed under the trade name Basell TPO-Nano, which incorporate nanoflakes to create stiff and lightweight exterior automotive parts.
2.2.3 Paint
Various commercial paint approaches are accessible for enhancing the thermal emission qualities of a surface with improved surface gratings. Each of these strategies possesses distinct strengths and limits, rendering them appropriate for a range of applications. The term “paint” generally refers to the substance in its liquid form, but once applied to a surface, it is called a “coating.” Paint typically consists of solid particles, known as “pigments,” dispersed in an organic medium called “resin.” These pigments often include complex metal oxides and semiconductors. Spectrally selective paints utilize various pigments, such as carbon, FeOx, melanin, Zn powder, silicon, PbS, and organic soot (e.g., Degussa FW2) (Gauden et al., 2008; Wijewardane and Goswami, 2012). Out of these options, paints containing TiO2 (rutile titania) are very efficient for radiative cooling purposes (Harrison, 1981). The efficacy of these pigments in obstructing or reflecting particular wavelengths is extensively demonstrated (Balfour, 1990). Rutile titania pigments, which are incorporated into a polymer matrix, are commonly employed in specialized paints that facilitate radiative cooling. On the other hand, FeMnOx has demonstrated superior performance as a pigment in sun thermal applications (Orel et al., 1993; Granqvist, 2012). These pigments have a great capacity to absorb solar energy within the spectrum, which makes them well-suited for heating applications.
3 Solar cell technologies
Solar cells are generally classified into three main generations based on their technology and stage of market development (Table 4). The first generation, which is widely used today, utilizes wafer-based crystalline silicon (c-Si) technology, available in two forms: single crystalline (sc-Si) and multi-crystalline (mc-Si). The second-generation solar cells, currently in the initial phases of market implementation, utilize thin-film technologies and can be classified into three primary types: (1) amorphous silicon (a-Si) and micromorph silicon (a-Si/μc-Si); (2) Cadmium Telluride (CdTe); and (3) Copper–Indium–Selenide (CIS) and Copper–Indium–Gallium–Diselenide (CIGS). The third generation of solar cells includes advanced technologies such as concentrating solar cells and organic solar cells, which are currently in the demonstration phase or have yet to reach broad commercialization. Furthermore, this generation includes nascent notions that are presently being developed. Figure 4 depicts the chronological improvement in the efficiency of solar cell conversion, emphasizing the notable breakthroughs made in each generation.
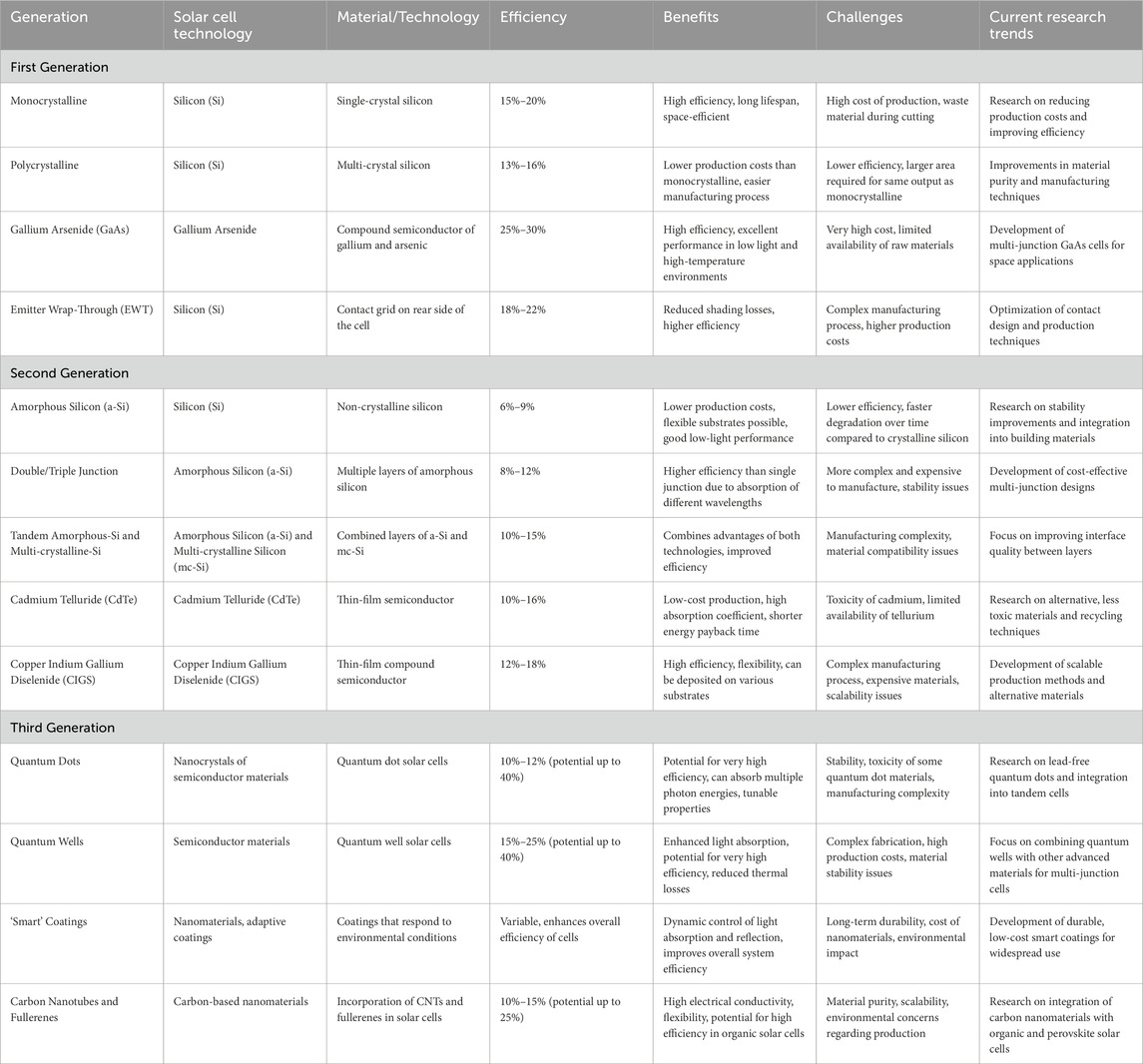
Table 4. A comprehensive table outlining different solar cell technologies across the first, second, and third generations.
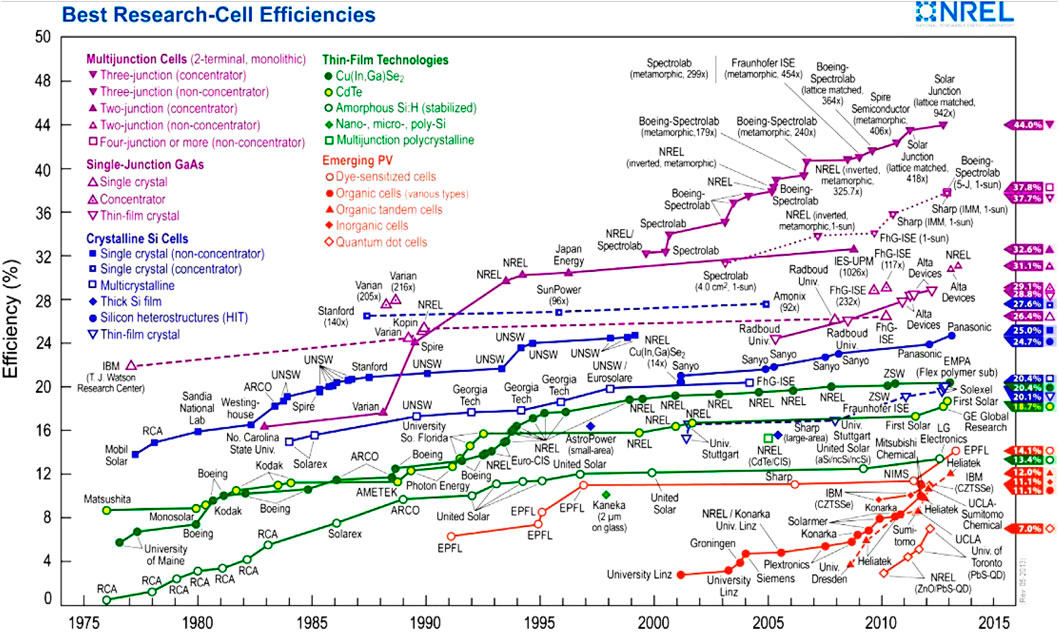
Figure 4. Efficiencies of different solar cell technologies under standard conditions. This figure compares first-, second-, and third-generation solar cells, highlighting efficiency trends and technological advancements. First-generation (monocrystalline, polycrystalline Si) offers high efficiency but at higher costs. Second-generation (thin-film CdTe, CIGS, a-Si) reduces costs but has lower efficiency. Third-generation (quantum dots, perovskites, multi-junction cells) surpasses traditional limits, offering higher potential efficiency. The figure underscores the trade-offs between efficiency, cost, and scalability in solar technology development.
3.1 First generation
The first generation of solar cell technologies is based on crystalline structures that use silicon (Si) to produce solar cells, which are then assembled into solar modules (Figure 5). Despite its longstanding presence, this technology remains relevant and is continuously being refined to improve its performance and efficiency. Several types of cells belong to the category of silicon crystalline structures, including as monocrystalline, polycrystalline, GaAs, and emitter wrap-through (EWT) cells. The subsequent sections will delve into these various technologies.
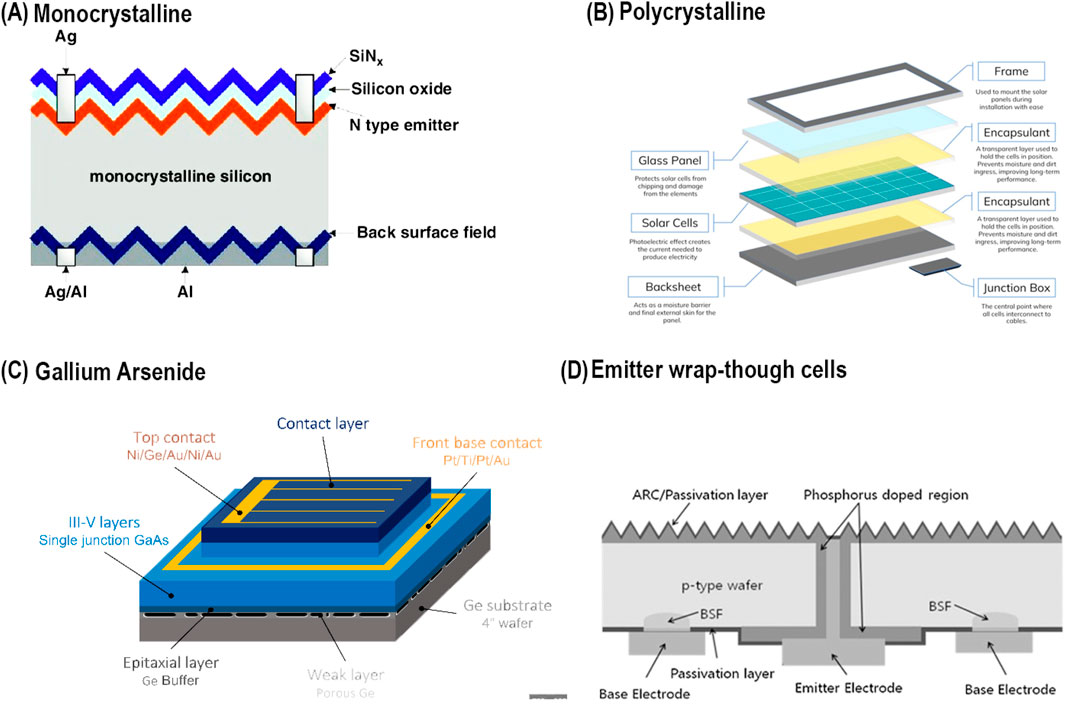
Figure 5. Structural designs of first-generation solar cells. (A) Monocrystalline silicon solar cell showing layers such as the SiNx anti-reflective coating, silicon oxide, N-type emitter, and back surface field for improved efficiency (Liu et al., 2014). (B) Polycrystalline silicon solar cell composed of multiple layers including a glass panel, encapsulant, solar cells, backsheet, and junction box, illustrating a typical module design. (C) Gallium Arsenide (GaAs) solar cell structure featuring III-V semiconductor layers, epitaxial Ge buffer, and multi-layered contacts for high efficiency in niche applications (Daniel et al., 2024). (D) Emitter wrap-through (EWT) solar cell with a p-type wafer and back-contact design, minimizing front-side metallization and enhancing light absorption and electrical performance (Cho et al., 2013).
Monocrystalline. The aforementioned solar cell variant is now the most prevalent in the industry, constituting over 80% of the total share. It is projected to maintain its dominance until a more proficient and economical alternative is introduced. The main dependence is on p-n junctions made of crystalline silicon (Si). The process of manufacturing monocrystalline silicon entails the cultivation of a solitary crystal ingot through the utilization of the Czochralski method (Abdin et al., 2013). However, the efficiency of these cells is inherently limited by the energy produced by photons, which decreases as wavelengths become longer. Additionally, longer-wavelength radiation results in thermal dissipation, which raises the cell’s temperature and subsequently lowers its overall efficiency. Monocrystalline silicon solar cells have reached a maximum efficiency of around 23% under standard test conditions (STC), with the highest recorded efficiency being 24.7%. However, the efficiency of solar cells is affected by several factors, including cell resistance, solar radiation reflection, and metal contacts on the cell surface. Once the Si ingot, which typically ranges from 10 to 15 cm in diameter, is produced, it is sliced into wafers approximately 0.3 mm thick. These wafers are then used to fabricate solar cells that generate approximately 35 mA of current per square centimeter and a voltage of 0.55 V under full illumination. Efficiencies of up to 30% under STC can be achieved for other semiconductor materials that are responsive to different wavelengths. Nevertheless, the efficiencies of modules are generally lower compared to those of individual cells. SunPower, however, has recently declared a remarkable 21% efficiency for its entire panels, which has been confirmed by the National Renewable Energy Laboratory (NREL) (Abdin et al., 2013).
Polycrystalline. Polycrystalline silicon (pc-Si) wafers may be manufactured across extensive surface areas, rendering them a financially efficient choice for the advancement of solar cell technology. Plasma processing is used to treat low-cost polycrystalline silicon (pc-Si) in order to generate a surface that allows more light to pass through, hence increasing light absorption. This method, known as reactive-ion etching, can result in a relative increase in absorption of approximately 40%. Although polycrystalline cells are less expensive to manufacture than monocrystalline cells, they often exhibit lesser efficiency. Nevertheless, monocrystalline cells exhibit a higher occurrence of metal contamination and crystal structural flaws in comparison to their counterparts. Polycrystalline silicon is manufactured by melting silicon and allowing it to cool in a controlled manner to align the crystal structure. The resulting material is formed into a rectangular ingot, which is then sliced into blocks and subsequently cut into thin wafers. An alternative approach involves producing very thin strips of polycrystalline silicon, a method developed by Evergreen Solar (Manna and Mahajan, 2007).
Gallium Arsenide (GaAs). A semiconductor material, exhibits a crystal structure that closely resembles that of silicon (Si). Conversely, crystalline silicon typically requires a thickness of at least 100 μm to effectively capture sunlight. In contrast, gallium arsenide, which has an ideal band gap of 1.43 eV, can achieve efficient light absorption with a thickness of only a few micrometers. Gallium arsenide (GaAs) solar cells exhibit a superior energy conversion efficiency, typically ranging from 25% to 30%, as compared to crystalline silicon (Si). Moreover, GaAs exhibits exceptional heat and radiation resistance, rendering it a superb option for concentrator systems and space-related uses. The main obstacle in the development of GaAs cells for terrestrial applications is the exorbitant expense associated with single-crystal GaAs substrates. In order to tackle this issue, two methods of reducing costs have been investigated: producing GaAs cells on less expensive substrates such as silicon or germanium (Ge), and cultivating GaAs cells on a detachable GaAs substrate. The detachable substrate can be recycled for the production of more cells or for the fabrication of GaAs thin films, employing manufacturing techniques like to those used for CIGS and CdTe thin films (Bright spot in a dark economy, 2009).
Emitter wrap-though cells. Emitter wrap-through (EWT) cells have achieved enhanced efficiency mostly through enhanced cell design rather than breakthroughs in the materials utilized. This method utilizes small laser-drilled apertures to establish a connection between the rear n-type contact and the emitter located on the opposite side. By removing the front connections, the full surface area of the cell becomes accessible for absorbing solar radiation, as it is no longer blocked by metal lines. Multiple studies have shown that moving the contacts to the rear of the cell offers manufacturing benefits. US-based companies such as Advent Solar and SunPower Corporation have implemented EWT technology, leading to a notable 15%–20% improvement in efficiency. Nevertheless, a notable disadvantage of this technology is its elevated series resistance, especially in expansive EWT cells, which has the potential to restrict the fill factor and overall efficiency (Kerschaver and Beaucarne, 2006).
3.2 Second generation
Thin-film technology offers a more economical option compared to crystalline silicon solar cells due to its reduced component requirements and simpler manufacturing process. Thin-film solar cells are exceptionally thin, usually measuring between 35 and 260 nm in thickness, thanks to the decreased amount of material used (Vrielink et al., 2012). There are five commercially significant varieties of thin-film cells, which will be covered in the following sections.
Amorphous silicon. Amorphous silicon (a-Si) was one of the earliest thin-film technologies developed (Carlson and Wronski, 1976). Unlike crystalline silicon, which has a structured lattice of silicon atoms, a-Si features a disordered arrangement of atoms (Mandal and Naskar, 2007). The lack of a regular atomic arrangement significantly affects the material’s electrical properties, resulting in a larger band gap of 1.7 eV in amorphous silicon, compared to the 1.1 eV band gap found in crystalline silicon. a-Si cells have a bigger band gap, which enables them to efficiently capture the visible part of the solar spectrum and reduces their sensitivity to the infrared region. This technology has multiple variations, which involve the utilization of diverse substrates such as glass or flexible stainless steel (SS). Additionally, there are different configurations available, such as tandem junction, double junction, and triple junction cells. Each of these variations offers different degrees of performance.
Amorphous-Si, double or triple junctions. Typically, amorphous silicon (a-Si) cells exhibit poorer efficiency in comparison to mono- and polycrystalline silicon cells. The current maximum efficiency attained in laboratory conditions for amorphous silicon (a-Si) cells is approximately 12%. Nevertheless, single-junction amorphous silicon modules have a tendency to deteriorate when exposed to sunlight, ultimately reaching a stable efficiency level of around 4%–8%. The degradation is principally induced by the Staebler-Wronski effect, which modifies the characteristics of hydrogenated amorphous silicon (Staebler and Wronski, 1977). In order to tackle the problems of efficiency and degradation, researchers have undertaken attempts to create multi-junction a-Si devices. These devices are specifically engineered to catch a wider spectrum of wavelengths emitted by solar radiation, encompassing both shorter and longer wavelengths, so enhancing their overall efficiency. The efficiencies of these systems, as rated under standard test conditions (STC), often fall within the range of 6%–7% (Jardine et al., 2001).
Tandem amorphous-Si and multi-crystalline-Si. Another approach to improving the efficiency of solar cells and modules is through the use of ‘stacked’ or multi-crystalline (mc) junctions, also known as micromorph thin-film technology. This method involves layering two or more solar cell junctions on top of each other. The top layer typically consists of a thin layer of amorphous silicon (a-Si), which excels at capturing the shorter wavelengths of the visible light spectrum. Microcrystalline silicon is more efficient for longer wavelengths and can also capture a portion of the infrared spectrum. The presence of several layers in this structure leads to increased efficiency in comparison to conventional amorphous silicon cells, with a typical range of 8%–9% depending on the specific cell shape and layer thickness. There has been substantial progress in the creation of thin-film silicon solar panels, particularly by businesses like as Oerlikon and Applied Materials (AMAT), who are at the forefront of creating the required equipment. AMAT has developed equipment with the ability to manufacture large thin-film a-Si and micromorph panels, reaching sizes of up to 5.5 m2 (El Chaar and El Zein, 2011).
Cadmium telluride (CdTe) and cadmium sulfide (CdS). CdTe, a polycrystalline semiconductor, is known for its strong light absorptivity. A thickness of around 1 mm is enough to absorb 90% of the sun spectrum. Another notable benefit of CdTe is its very straightforward and economical manufacturing method. Nevertheless, its conversion efficiency is similar to that of a-Si, which is relatively poor (Villar et al., 2009). Small-area CdTe cells can obtain efficiencies above 15% (Yousefi et al., 2012), although CdTe modules generally attain efficiencies surpassing 9% (Liu et al., 2013). Several primary obstacles in the development of CdTe solar cells involve the complexities associated with doping p-type CdTe, achieving low-resistance connections to p-type CdTe, and minimizing recombination losses at the junction interface (Matsumoto et al., 2004). Furthermore, the production process must be conducted with meticulous precautions due to the very hazardous nature of cadmium. One significant obstacle to the wider use of CdTe in solar technology is the lack of stability in the performance of cells and modules over time. Tang et al. (Li et al., 2003) have recently created solar cells utilizing nanowires with a cadmium sulfide core and a copper sulfide shell. They achieved this by using a cation exchange reaction that operates at low temperatures and is based on a solution. Nanowire solar cells, which are produced using a simple and cost-effective manufacturing process, achieved an energy conversion efficiency of 5.4%, comparable to that of conventional planar solar cells. The open-circuit voltage and fill factor, key factors in determining the solar cell’s maximum energy output, showed encouraging performance, highlighting the potential of this technology.
Copper indium diselenide (CIS) or copper indium gallium diselenide (CIGS). Anticipated progress in the near future is expected as a result of the material system’s ability to develop compounds with specified features, such as bandgap grading, with a high degree of flexibility. Gaining a more profound comprehension of the characteristics of surfaces and junctions could potentially result in the creation of diverse devices and create new opportunities for ternary thin-film solar cells (Goetzberger et al., 2003). Although CIS (Copper Indium Selenide) is a very efficient material for solar cells, its manufacturing process is intricate, which presents difficulties in production. In addition, it is necessary to address safety problems during the production process due to the specific composition, density, and adhesive qualities of the material (Guimard et al., 2002). CIGS (Copper Indium Gallium Selenide) is a multi-layered thin-film hybrid that includes gallium to enhance the bandgap. CIGS cells are characterized by a more intricate heterojunction paradigm, in contrast to the straightforward p-n junction silicon cells. The highest recorded efficiency for thin-film solar cells is around 20% using CIGS (Repins et al., 2008), but large-area modules normally achieve an efficiency of about 13% (Powalla and Dimmler, 2000). A significant obstacle faced by CIGS modules is the complexity of expanding the production process to achieve high productivity, high efficiency, and low expenses. Meyer and van Dyk conducted an experiment to assess the performance of CIS and other thin-film materials (Meyer and Van Dyk, 2003). The results showed that CIS only experienced a 10% degradation after being subjected to 130 kWh/m2 of outdoor settings. This level of degradation is lower than that observed in other thin-film materials. In addition, the absorption coefficient of CuInSe2 exceeds 105 cm-1, which enhances its efficiency in solar cells.
3.3 Third-generation
At present, third-generation solar cell technologies are in the pre-commercial stage. This category encompasses a range of innovations, from demonstration-stage systems like multi-junction concentrating solar cells to new concepts requiring extensive fundamental research and development, such as quantum-structured solar cells. Scientists are now investigating many advanced solar cell technologies, such as those that make use of quantum dots, quantum wires, quantum wells, and superlattice structures (Beard et al., 2013; Katiyar et al., 2012). These technologies have the potential to be incorporated into concentrating solar cell systems, enabling them to achieve exceptionally high efficiencies by surpassing the thermodynamic constraints of traditional crystalline cells, such as the Shockley-Queisser limit, which restricts power efficiency to 31%–41%. While these methods show potential for being highly efficient, they are currently in the initial phases of materials research. This document also includes a concise evaluation of various solar cell technologies currently under progress.
Quantum dots. Quantum dots (QDs) present a great opportunity to improve the efficiency of solar energy in a more economically efficient way. QDs have the capacity to transform solar technology and offer a feasible alternative energy source with substantial promise. By incorporating quantum dots into solar cells, their energy generation capacity is significantly enhanced, resulting in highly efficient energy production. In 2011, the U.S. National Renewable Energy Laboratory (NREL) created an innovative device that utilizes lead selenide (PbSe) quantum dots (QDs) to achieve multiple electron generation (MEG) or carrier multiplication. This breakthrough received significant attention due to its quantum efficiency, which surpassed 100%, surpassing that of conventional silicon solar cells. Studies have demonstrated that the majority of current quantum dot solar cells typically attain performance levels of approximately 10% (Laboratory NREL and N.R.E, 2011). However, by employing carrier multiplication techniques and leveraging the distinctive quantum features of quantum dots (QDs), it is possible to obtain substantially greater efficiencies. Quantum dots are currently being utilized in the development of solar cells that utilize “energy transfer”. QDs have the ability to absorb sunlight of multiple wavelengths due to their diverse sizes and volumes. These solar cells employ layers of quantum dots (QDs) with varying sizes to effectively capture a wider range of the solar spectrum, hence enhancing light absorption. The variety in quantum dot (QD) size enables the absorption of different wavelengths, resulting in increased energy generation within the cell. In addition, the stable state at the p-n junction inhibits the recombination of electrons and holes, hence enhancing the efficiency of the cell (Laboratory LANL, 2012). Quantum dots have the capability to produce advanced solar cells that possess the qualities of being cost-effective and exceptionally efficient. Their capacity to modify band gaps and capture different wavelengths makes them highly efficient. The band gap energy of quantum dots (QDs) exhibits an inverse relationship with their size, allowing them to effectively capture a broader range of sun wavelengths and so maximize energy harvesting. Research has demonstrated that quantum dots have the capability to increase the efficiency of solar cells by as much as 44%, underscoring their substantial promise in advancing solar technology (ScienceDaily, 2012).
Quantum wells. A quantum well (QW) is a confined region where energy levels are distinct and separate. Quantum wells (QWs) are created in semiconductors by sandwiching a material like gallium arsenide (GaAs) between two layers of a material with a wider band gap, such as aluminum arsenide (AlAs) (Scavennec et al., 2009). In solar cells, QWs confine charge carriers, like electrons and holes, to move in two dimensions rather than three, thereby influencing their behavior. The conduct of these electric charges is affected by the thickness of the semiconductor layer, typically varying from 1 to 10 nm. Courel et al. (Courel et al., 2012a) developed a novel approach by integrating GaAs/GaInNAs multiple quantum wells and superlattices with the intrinsic layer of conventional GaAs p–i–n solar cells. They first examined a GaAs/GaInNAs multiple quantum well solar cell (MQWSC) to explore how the conversion efficiency is affected by the dimensions (width and depth) of the quantum wells. Their study addresses strategies for improving the nitrogen content and quantum well width to enhance solar cell efficiency. They subsequently conducted a theoretical analysis of the GaAs/GaInNAs superlattice solar cell (SLSC) to assess its viability. This analysis involved calculating the effective density of states and the absorption coefficient for the superlattice structure to determine its current density-voltage (J-V) characteristics. The conversion efficiency of the SLSC was thereafter compared to the greatest efficiency achieved by the MQWSC (Courel et al., 2012b). The findings revealed a notable enhancement in the performance of the GaAs/GaInNAs SLSC with the implementation of solar concentration. In order to improve the efficiency of collecting light in InGaN-based MQWSCs, Lin et al. (Lin et al., 2011) employed ZnO nanorod arrays (NRAs) as an antireflection (AR) layer. The utilization of 1.1-μm-long nanorod arrays (NRAs) led to an approximately 36% enhancement in conversion efficiency, which can be attributed to the enhancement in optical transmission. This approach highlights the potential of utilizing cost-effective ZnO nanorod arrays (NRAs) for nanofabrication in anti-reflective (AR) coatings, consequently enhancing the efficiency of light collecting in indium gallium nitride-based multiple quantum well solar cells (MQWSCs).
Carbon nanotubes and fullerenes. Over the past 2 decades, substantial research has been conducted on the physicochemical properties of carbon in various reduced dimensions. This research started with fullerenes (0D), then progressed to carbon nanotubes (1D), carbon nanohorns, and most recently, graphene (2D) (Guldi and Martin, 2002; Akasaka et al., 2024). These many forms of carbon have demonstrated significant promise for a diverse array of uses. Fullerenes, the first molecular-level sp2 carbon allotropes, marked a groundbreaking discovery that led to significant advancements in materials science. These structures have primarily been explored as n-type semiconductor materials in organic field-effect transistors (Matsuo et al., 2012; Chen et al., 2012) and organic photovoltaic (OPV) cells (Arias et al., 2010; Liang et al., 2011). However, they have also garnered considerable attention in inexpensive solar energy conversion devices. Spin-coating has become the preferred method for applying films of conjugated polymers, fullerenes, or electron donor-acceptor conjugates onto electrode surfaces in traditional bulk heterojunction solar cells. Carbon nanotubes (CNTs) can be described as graphene sheets rolled into seamless, single-walled cylinders. Vertically aligned carbon nanotubes (CNTs) are highly advantageous in hybrid solar cells due to their ability to enhance surface area and establish excellent contact with the electrode. Moreover, their alignment closely corresponds to the optimal shape required for solar cells (Bassani et al., 2010). The utilization of carbon nanotubes (CNTs) in dye-sensitized solar cells (DSSCs) has been extensively studied (Zhang and Cao, 2011). Zhang et al. (Zhang et al., 2010) have successfully fabricated DSSC devices by employing double-walled CNT counter electrodes manufactured through a printing technique. In addition, polymer organic photovoltaic (OPV) systems have been fabricated utilizing transparent electrodes composed of carbon nanotubes (CNTs). Graphene, a synthetic carbon allotrope, was initially obtained in 2004 by mechanically exfoliating graphite with Scotch tape (Cao et al., 2010). A single layer of graphene exhibits an optical transmittance of 97.7% (Nair et al., 2008). Transparent conducting films like indium tin oxide (ITO) and fluorine-doped tin oxide (FTO) are commonly used in inorganic, organic, and dye-sensitized solar cells. However, the increasing demand for alternatives arises from the scarcity of indium and the substantial production expenses linked to these substances. Graphene is a highly promising material for creating transparent conducting films due to its excellent electrical mobility, optical transparency, and flexibility (Yin et al., 2010). Additionally, it is a cost-effective option as it is made from carbon, which is an affordable raw material. Graphene sheets vary in transparency from 97% for a single layer to 84% for seven layers. Researchers have developed composite films of graphene/CdS and graphene/ZnO using ultrathin, high-mobility graphene films. These composites are designed for incorporation into glass/graphene/ZnO/CdS/CdTe/(graphite paste) solar cells. The achieved photovoltaic power conversion efficiency of these cells is 4.17%. Graphene-based ultrathin films have the potential to be a cost-effective material for the front electrode in solar devices. Nevertheless, a significant obstacle in utilizing graphene in OPV devices is its inadequate hydrophilicity, which detrimentally impacts the fabrication of solution-processed devices (Bi et al., 2011). In order to tackle this problem, researchers have utilized large-scale, uninterrupted, see-through, and extremely conductive few-layer graphene films created using chemical vapor deposition as an anode in organic photovoltaic devices. Additionally, a chemical treatment has been performed to the graphene layer to improve its efficiency. Dye-sensitized solar cells (DSSCs) have been thoroughly investigated as an economical substitute for conventional silicon-based solar cells. Recent studies have demonstrated encouraging outcomes by employing graphene monolayers as the active constituents. Yan et al. (Yan et al., 2013) introduced an innovative method by employing large, easily processable graphene quantum dots as light absorbers in photovoltaic devices. These solar cells were sensitized with black graphene quantum dots synthesized through solution chemistry techniques. Fullerene, a form of carbon, has demonstrated high effectiveness as an electron acceptor in bulk heterojunction organic photovoltaic (OPV) devices, achieving conversion efficiencies exceeding 8%. As previously noted, carbon-based materials hold significant promise for replacing rare metals in electrodes and interface layers in future solar technologies (Bakulin et al., 2012).
4 Challenges
Nanofluids present significant potential for a wide range of applications. However, researchers encounter various challenges in advancing this field, including: (i) Variability in experimental outcomes among different research teams, (ii) instability of nanoparticle suspensions over time, (iii) insufficient theoretical insight into essential energy transport mechanisms, (iv) challenges including increased pressure drop, higher viscosity, reduced specific heat, and elevated costs related to nanofluids, (v) the need for environmentally sustainable, large-scale production methods, (vi) the requirement for experimental investigations into the convective heat transfer properties of nanofluids, and (vii) the importance of accounting for factors such as temperature-dependent changes in thermophysical properties, particle migration, and Brownian motion during experimental studies (Wang and Mujumdar, 2008; Soltani et al., 2021; Abbasi and Souri, 2020). The difficulties associated with nanotechnology encompass the long-term stability of nanoparticle dispersions, elevated pressure drops, increased pumping power demands, thermal performance of nanofluids in turbulent and fully developed flow conditions, high viscosity, low specific heat, and thermal conductivity. Additionally, the economic viability of nanofluids and challenges in their manufacturing process are also significant concerns.
The manufacture of nanoparticles is a substantial obstacle in the progress of nanotechnologies. An important concern arises from the fact that the advantages of a large surface area can be reduced as a result of the clumping together of larger particles. In order to tackle this issue, additives are frequently incorporated into the base fluid that contains nanoparticles in order to enhance performance. Nevertheless, the utilization of additives has inherent disadvantages, including the modification of the surface characteristics of the particles and the potential introduction of undesirable amounts of contaminants. In order to obtain precise information on the characteristics of nanofluids, it is necessary to use higher quantities of samples during the testing process. The utilization of ultrasonic vibration is frequently employed to augment the dispersion of nanoparticles and disintegrate clusters. The duration and intensity of ultrasonication have a substantial impact on the dispersion properties. However, even after ultrasonication, clusters have a tendency to reassemble and increase in size over time. Therefore, varying results can be seen in apparently identical samples due to the time gap between ultrasonication and the assessment of nanofluid properties. Although nanotechnology has markedly enhanced solar energy conversion and storage, various obstacles impede its extensive implementation. A primary challenge is the long-term stability of nanomaterials, especially in solar and energy storage applications. Research indicates that perovskite solar cells, although exhibiting great efficiency, are prone to environmental degradation, moisture sensitivity, and thermal instability, which restrict their operational longevity. The environmental impact of nanomaterials is a significant worry, particularly with the toxicity of specific nanoparticles, such as lead-based perovskites, and the challenges associated with recycling nanostructured materials. Moreover, the expenses related to the synthesis of high-performance nanomaterials present economic obstacles to widespread implementation. Despite the exploration of breakthroughs in scalable synthesis techniques, difficulties include the necessity for costly precursors, intricate manufacturing procedures, and energy-intensive processes must be resolved to attain cost-effective solutions. Future research must prioritize the development of more stable, non-toxic, and cost-effective nanomaterials, while incorporating sustainability factors such as lifecycle evaluations and recycling techniques into their design (Urbina, 2020; Mahmud, 2023). The notable temperature dependence of nanofluids’ thermal conductivity is a key feature that could enhance their range of applications. However, before nanofluids can be successfully commercialized, additional challenges such as flow erosion and particle settling must be addressed. Thorough analysis and resolution are necessary to address these possible difficulties and ensure the practical use of nanofluids (Hong and Yang, 2005).
5 Future outlook and concluding remarks
Additional research is crucial to enhance our comprehension of the thermal transfer characteristics of nanofluids and their use in solar energy technologies. This review emphasizes that (i) solar energy is a plentiful but underexploited resource, with nanofluids presenting a potential method for enhancing its capture and conversion efficiency; (ii) the incorporation of nanofluids in direct absorption solar collectors can improve performance by as much as 10%; and (iii) although first-generation solar cells are fully commercialized, later generations necessitate additional optimization prior to widespread implementation. Future research should concentrate on (i) creating stable, cost-efficient nanofluids with minimal ecological repercussions, (ii) improving large-scale production techniques to guarantee economic viability, (iii) optimizing nanofluid compatibility with solar technologies to enhance energy conversion efficiency, and (iv) systematically examining convection heat transfer phenomena utilizing metallic nanoparticles of diverse shapes and concentrations to maximize thermal performance across various flow conditions. Overcoming these hurdles may expedite the practical use of nanofluids in solar energy systems, rendering them a feasible solution for sustainable energy production.
The incorporation of ‘smart’ coatings and nanostructured components offers a promising avenue for enhancing energy efficiency in solar and building applications. Electrochromic and thermochromic devices can effectively regulate light and heat transmission, hence diminishing dependence on artificial lighting and HVAC systems. Electrochromic windows are distinguished by their significant transparency modulation, low power consumption during switching, and proven reliability in commercial applications. Research indicates that electrochromic windows can decrease lighting energy usage by as much as 26% and lower peak cooling demands by 20% in hot regions such as California. Nonetheless, widespread adoption remains hindered by problems including sluggish switching speeds, performance variability in extensive installations, and insufficient data regarding their efficacy in cold climates. Future advancements in nanomaterials, such as increased stability under UV exposure and improved response times, may alleviate these challenges and broaden the practical application of smart coatings in solar energy systems.
Author contributions
AF: Conceptualization, Data curation, Investigation, Methodology, Software, Supervision, Writing – original draft, Writing – review and editing, Formal Analysis, Funding acquisition, Project administration, Resources, Validation, Visualization.
Funding
The author(s) declare that no financial support was received for the research and/or publication of this article.
Conflict of interest
The author declares that the research was conducted in the absence of any commercial or financial relationships that could be construed as a potential conflict of interest.
Generative AI statement
The author(s) declare that no Generative AI was used in the creation of this manuscript.
Publisher’s note
All claims expressed in this article are solely those of the authors and do not necessarily represent those of their affiliated organizations, or those of the publisher, the editors and the reviewers. Any product that may be evaluated in this article, or claim that may be made by its manufacturer, is not guaranteed or endorsed by the publisher.
References
Aali, H., Kazemi, S., Larijani, R. S., Zarghami, R., and Mostoufi, N. (2023). CFD-DEM modeling of breakage of non-spherical particles in fluidized beds. Chem. Eng. Res. Des. 189, 593–605. doi:10.1016/j.cherd.2022.11.047
Abbasi, S., and Souri, M. (2020). Reducing aerodynamic noise in a rod-airfoil using suction and blowing control method. Int. J. Appl. Mech. 12, 2050036. doi:10.1142/s1758825120500362
Abbasi, S., and Souri, M. (2021). On the passive control of aeroacoustics noise behind a square cylinder. J. Braz. Soc. Mech. Sci. Eng. 43, 58–16. doi:10.1007/s40430-021-02797-w
Abdin, Z., Alim, M. A., Saidur, R., Islam, M. R., Rashmi, W., Mekhilef, S., et al. (2013). Solar energy harvesting with the application of nanotechnology. Renew. Sustain. energy Rev. 26, 837–852. doi:10.1016/j.rser.2013.06.023
Adachi, M., Murata, Y., Takao, J., Jiu, J., Sakamoto, M., and Wang, F. (2004). Highly efficient dye-sensitized solar cells with a titania thin-film electrode composed of a network structure of single-crystal-like TiO2 nanowires made by the “oriented attachment” mechanism. J. Am. Chem. Soc. 126, 14943–14949. doi:10.1021/ja048068s
Anika, U. A., Kibria, M. G., Kanka, S. D., Mohtasim, M. S., Paul, U. K., and Das, B. K. (2024). Exergy, exergo-economic, environmental and sustainability analysis of pyramid solar still integrated hybrid nano-PCM, black sand, and sponge. Sol. Energy 274, 112559. doi:10.1016/j.solener.2024.112559
Arias, A. C., MacKenzie, J. D., McCulloch, I., Rivnay, J., and Salleo, A. (2010). Materials and applications for large area electronics: solution-based approaches. Chem. Rev. 110, 3–24. doi:10.1021/cr900150b
Bakulin, A. A., Rao, A., Pavelyev, V. G., van Loosdrecht, P. H., Pshenichnikov, M. S., Niedzialek, D., et al. (2012). The role of driving energy and delocalized states for charge separation in organic semiconductors. Science 335, 1340–1344. doi:10.1126/science.1217745
Balfour, J. (1990). Back to basics: opacity and titanium dioxide pigments. J. Oil Colour Chemists' Assoc. 73.
Bassani, D. M., Jonusauskaite, L., Lavie-Cambot, A., McClenaghan, N. D., Pozzo, J.-L., Ray, D., et al. (2010). Harnessing supramolecular interactions in organic solid-state devices: current status and future potential. Coord. Chem. Rev. 254, 2429–2445. doi:10.1016/j.ccr.2010.05.007
Bavykin, D. V., Friedrich, J. M., and Walsh, F. C. (2006). Protonated titanates and TiO2 nanostructured materials: synthesis, properties, and applications. Adv. Mater. 18, 2807–2824. doi:10.1002/adma.200502696
Beard, M. C., Luther, J. M., Semonin, O. E., and Nozik, A. J. (2013). Third generation photovoltaics based on multiple exciton generation in quantum confined semiconductors. Accounts Chem. Res. 46, 1252–1260. doi:10.1021/ar3001958
Bi, H., Huang, F., Liang, J., Xie, X., and Jiang, M. (2011). Transparent conductive graphene films synthesized by ambient pressure chemical vapor deposition used as the front electrode of CdTe solar cells. Adv. Mater. 28, 3202–3206. doi:10.1002/adma.201100645
Bright spot in a dark economy (2009). Available online at: http://www.pddnet.com/articles/2009/10/bright-spot-dark-economy.
Cao, A., Liu, Z., Chu, S., Wu, M., Ye, Z., Cai, Z., et al. (2010). A facile one-step method to produce graphene–CdS quantum dot nanocomposites as promising optoelectronic materials. Adv. Mater. 22, 103–106. doi:10.1002/adma.200901920
Carlson, D. E., and Wronski, C. R. (1976). Amorphous silicon solar cell. Appl. Phys. Lett. 28, 671–673. doi:10.1063/1.88617
Cerón, I., Neila, J., and Khayet, M. (2011). Experimental tile with phase change materials (PCM) for building use. Energy Build. 43, 1869–1874. doi:10.1016/j.enbuild.2011.03.031
Chang, H., Kao, M.-J., Cho, K.-C., Chen, S.-L., Chu, K.-H., and Chen, C.-C. (2011). Integration of CuO thin films and dye-sensitized solar cells for thermoelectric generators. Curr. Appl. Phys. 11, S19–S22. doi:10.1016/j.cap.2010.12.039
Chang, H., Wu, H., Chen, T., Huang, K., Jwo, C., and Lo, Y. (2010). Dye-sensitized solar cell using natural dyes extracted from spinach and ipomoea. J. Alloys Compd. 495, 606–610. doi:10.1016/j.jallcom.2009.10.057
Chen, S.-l., Su, H.-t., Chang, H., Jwo, C.-s., and Feng, H. J. (2010). TiO2 nanoparticles produced by electric-discharge-nanofluid-process as photoelectrode of DSSC. Chin. J. Chem. Phys. 23, 231–236. doi:10.1088/1674-0068/23/02/231-236
Chen, Y., Hu, Y., Fang, Y., Li, P., Feng, C., and Zhang, J. (2012). Lattice-directed growth of single-walled carbon nanotubes with controlled geometries on surface. Carbon 50, 3295–3297. doi:10.1016/j.carbon.2011.12.019
Cho, J., Lee, H., Hyun, D., Lee, Y., Jung, W., and Hong, J. (2013). Efficiency enhanced emitter wrap-through (EWT) screen-printed solar cells with non-uniform thickness of silicon nitride passivation layer in via-holes. Sol. energy 90, 188–194. doi:10.1016/j.solener.2013.01.008
Choi, S. U., and Eastman, J. A. (2001). Enhanced heat transfer using nanofluids. Argonne, IL United States: Argonne National Laboratory ANL.
Chow, T. T., Tiwari, G., and Menezo, C. (2012). Hybrid solar: a review on photovoltaic and thermal power integration. Int. J. Photoenergy 2012, 1–17. doi:10.1155/2012/307287
Courel, M., Rimada, J. C., and Hernández, L. (2012a). GaAs/GaInNAs quantum well and superlattice solar cell. Appl. Phys. Lett. 100. doi:10.1063/1.3687195
Courel, M., Rimada, J. C., and Hernández, L. (2012b). An approach to high efficiencies using GaAs/GaInNAs multiple quantum well and superlattice solar cell. J. Appl. Phys. 112. doi:10.1063/1.4749418
Cui, Y., and Zhu, Q. (2012). “Study of photovoltaic/thermal systems with MgO-water nanofluids flowing over silicon solar cells,” in 2012 asia-pacific power and energy engineering conference. IEEE, 1–4.
Daniel, V., Bidaud, T., Chretien, J., Paupy, N., Ayari, A., Diallo, T. M., et al. (2024). High-efficiency GaAs solar cells grown on porous germanium substrate with PEELER technology. Sol. RRL 8, 2300643. doi:10.1002/solr.202300643
El Chaar, L., and El Zein, N. (2011). Review of photovoltaic technologies. Renew. Sustain. energy Rev. 15, 2165–2175. doi:10.1016/j.rser.2011.01.004
Frankl, P., Nowak, S., Gutschner, M., Gnos, S., and Rinke, T. (2010). Technology roadmap: solar photovoltaic energy. Int. Energy Assoc.
Gauden, M., Pezzella, A., Panzella, L., Neves-Petersen, M., Skovsen, E., Petersen, S. B., et al. (2008). Role of solvent, pH, and molecular size in excited-state deactivation of key eumelanin building blocks: implications for melanin pigment photostability. J. Am. Chem. Soc. 130, 17038–17043. doi:10.1021/ja806345q
Goetzberger, A., Hebling, C., and Schock, H.-W. (2003). Photovoltaic materials, history, status and outlook. Mater. Sci. Eng. R Rep. 40, 1–46. doi:10.1016/s0927-796x(02)00092-x
Granqvist, C. G. (2012). Oxide electrochromics: an introduction to devices and materials. Sol. Energy Mater. Sol. Cells 99, 1–13. doi:10.1016/j.solmat.2011.08.021
Green, M. A. (2000). Prospects for photovoltaic efficiency enhancement using low-dimensional structures. Nanotechnology 11, 401–405. doi:10.1088/0957-4484/11/4/342
Guimard, D., Grand, P., Bodereau, N., Cowache, P., Guillemoles, J.-F., Lincot, D., et al. (2002). “Copper indium diselenide solar cells prepared by electrodeposition,” in Conference record of the twenty-ninth IEEE photovoltaic specialists conference. IEEE, 692–695.
Guldi, D. M., and Martin, N. (2002). Carbon nanotubes and related structures: synthesis, characterization, functionalization, and applications. John Wiley & Sons2010.
Harrison, A. (1981). Effect of atmospheric humidity on radiation cooling. Sol. Energy 26, 243–247. doi:10.1016/0038-092x(81)90209-7
He, Y., Wang, S., Ma, J., Tian, F., and Ren, Y. (2011). Experimental study on the light-heat conversion characteristics of nanofluids. Nanosci. Nanotechnol. Lett. 3, 494–496. doi:10.1166/nnl.2011.1194
Hong, T.-K., and Yang, H.-S. (2005). Nanoparticle-dispersion-dependent thermal conductivity in nanofluids. J. Korean Phys. Soc. 47, 321. doi:10.3938/jkps.47.321
Hung, T.-C., and Yan, W.-M. (2012). Enhancement of thermal performance in double-layered microchannel heat sink with nanofluids. Int. J. Heat Mass Transf. 55, 3225–3238. doi:10.1016/j.ijheatmasstransfer.2012.02.057
Ismail, M., Dincer, I., and Bicer, Y. (2024). Modeling and simulation of nano-enriched latent heat thermal storage system for concentrated solar energy. J. Energy Storage 78, 110071. doi:10.1016/j.est.2023.110071
Jana, R., Sayan, S., and Das, S. (2024). A review on nanotechnology for catalysis and solar energy conversion. Int. J. Renew. Energy Its Commer. 10, 32–48. doi:10.37628/IJREC
Jardine, C. N., Conibeer, G. J., and Lane, K. (2001). PV-COMPARE: direct comparison of eleven PV technologies at two locations in northern and southern Europe. In Seventeenth EU PVSEC.
Katiyar, R., Auciello, O., and Thomas, R. (2012). Preface to the International Symposium on Integrated Functionalities (ISIF 2010) special issue of journal of applied physics science and technology of integrated functionalities. J. Appl. Phys. 111. doi:10.1063/1.4712272
Kazemi, S., Aali, H., Saghafian Larijani, R., Zarghami, R., Liu, H., and Mostoufi, N. (2024). Effect of external electric field on fluidization of rodlike particles using CFD–DEM. Industrial and Eng. Chem. Res. 63, 20937–20949. doi:10.1021/acs.iecr.4c02474
Kazemi, S., Tashakori-Asfestani, F., Kheirabadi, S., Zarghami, R., and Mostoufi, N. (2025). CFD-DEM investigation of fine charged particle coating in fluidized beds. Chem. Eng. Res. Des. 216, 160–173. doi:10.1016/j.cherd.2025.02.030
Kerschaver, E. V., and Beaucarne, G. (2006). Back-contact solar cells: a review. Prog. photovoltaics Res. Appl. 14, 107–123. doi:10.1002/pip.657
Khullar, V., and Tyagi, H. (2010). “Application of nanofluids as the working fluid in concentrating parabolic solar collectors,” in Proceedings of the 37th national and 4th international conferences on fluid mechanics and fluid power.
Khullar, V., Tyagi, H., Phelan, P. E., Otanicar, T. P., Singh, H., and Taylor, R. A. (2012). Solar energy harvesting using nanofluids-based concentrating solar collector. J. Nanotechnol. Eng. Med. 3, 031003. doi:10.1115/1.4007387
Laboratory(LANL) (2012). L.A.N. Exploratory devices; Available online at: http://quantumdot.lanl.gov/devices.shtml.
Laboratory(NREL), N.R.E (2011). NREL Scientists report first solar cell producing more electrons in photocurrent than solar photons entering cell; Available online at: http://www.nrel.gov/news/press/2011/1667.html.
Li, W., Liang, C., Zhou, W., Qiu, J., Sun, G., Xin, Q., et al. (2003). Preparation and characterization of multiwalled carbon nanotube-supported platinum for cathode catalysts of direct methanol fuel cells. J. Phys. Chem. B 107, 6292–6299. doi:10.1021/jp022505c
Liang, Z., Cormier, R. A., Nardes, A. M., and Gregg, B. A. (2011). Developing perylene diimide based acceptor polymers for organic photovoltaics. Synth. Met. 161, 1014–1021. doi:10.1016/j.synthmet.2011.03.009
Lin, G., Lai, K., Lin, C., Lai, Y.-L., and He, J. (2011). Efficiency enhancement of InGaN-based multiple quantum well solar cells employing antireflective ZnO nanorod arrays. IEEE Electron Device Lett. 32, 1104–1106. doi:10.1109/led.2011.2158061
Liu, C. P., Chang, M. W., and Chuang, C. L. (2014). Effect of rapid thermal oxidation on structure and photoelectronic properties of silicon oxide in monocrystalline silicon solar cells. Curr. Appl. Phys. 14, 653–658. doi:10.1016/j.cap.2014.02.017
Liu, Z.-H., Hu, R.-L., Lu, L., Zhao, F., and Xiao, H.-s. (2013). Thermal performance of an open thermosyphon using nanofluid for evacuated tubular high temperature air solar collector. Energy Convers. Manag. 73, 135–143. doi:10.1016/j.enconman.2013.04.010
Mahmud, M. Z. A. (2023). A concise review of nanoparticles utilized energy storage and conservation. J. Nanomater. 2023, 1–14. doi:10.1155/2023/5432099
Mandal, R., and Naskar, R. (2007). An unique method of characterization and performance estimation of various solar photovoltaic cells/modules using microcontroller: a review.
Manna, T. K., and Mahajan, S. M. (2007). “Nanotechnology in the development of photovoltaic cells,” in 2007 international conference on clean electrical power. IEEE, 379–386.
Manning, T. D., Parkin, I. P., Pemble, M. E., Sheel, D., and Vernardou, D. (2004). Intelligent window coatings: atmospheric pressure chemical vapor deposition of tungsten-doped vanadium dioxide. Chem. Mater. 16, 744–749. doi:10.1021/cm034905y
Matsumoto, T., Komatsu, T., Nakano, H., Arai, K., Nagashima, Y., Yoo, E., et al. (2004). Efficient usage of highly dispersed Pt on carbon nanotubes for electrode catalysts of polymer electrolyte fuel cells. Catal. Today 90, 277–281. doi:10.1016/j.cattod.2004.04.038
Matsuo, Y., Okada, H., Maruyama, M., Sato, H., Tobita, H., Ono, Y., et al. (2012). Covalently chemical modification of lithium ion-encapsulated fullerene: synthesis and characterization of [Li+@ PCBM] PF6. Org. Lett. 14, 3784–3787. doi:10.1021/ol301671n
Meyer, E., and Van Dyk, E. (2003). Characterization of degradation in thin-film photovoltaic module performance parameters. Renew. Energy 28, 1455–1469. doi:10.1016/s0960-1481(02)00062-9
Nair, R. R., Blake, P., Grigorenko, A. N., Novoselov, K. S., Booth, T. J., Stauber, T., et al. (2008). Fine structure constant defines visual transparency of graphene. science 320, 1308. doi:10.1126/science.1156965
Nakata, K., and Fujishima, A. (2012). TiO2 photocatalysis: design and applications. J. Photochem. Photobiol. C Photochem. Rev. 13, 169–189. doi:10.1016/j.jphotochemrev.2012.06.001
Nguyen, C. T., Roy, G., Gauthier, C., and Galanis, N. (2007). Heat transfer enhancement using Al2O3–water nanofluid for an electronic liquid cooling system. Appl. Therm. Eng. 27, 1501–1506. doi:10.1016/j.applthermaleng.2006.09.028
Ochiai, T., and Fujishima, A. (2012). Photoelectrochemical properties of TiO2 photocatalyst and its applications for environmental purification. J. Photochem. Photobiol. C Photochem. Rev. 13, 247–262. doi:10.1016/j.jphotochemrev.2012.07.001
Orel, B., Gunde, M. K., and Krainer, A. (1993). Radiative cooling efficiency of white pigmented paints. Sol. energy 50, 477–482. doi:10.1016/0038-092x(93)90108-z
Otanicar, T. P., Phelan, P. E., Prasher, R. S., Rosengarten, G., and Taylor, R. A. (2010). Nanofluid-based direct absorption solar collector. J. Renew. Sustain. energy 2. doi:10.1063/1.3429737
Parida, B., Iniyan, S., and Goic, R. (2011). A review of solar photovoltaic technologies. Renew. Sustain. energy Rev. 15, 1625–1636. doi:10.1016/j.rser.2010.11.032
Peyghambarzadeh, S., Hashemabadi, S., Jamnani, M. S., and Hoseini, S. (2011). Improving the cooling performance of automobile radiator with Al2O3/water nanofluid. Appl. Therm. Eng. 31, 1833–1838. doi:10.1016/j.applthermaleng.2011.02.029
Powalla, M., and Dimmler, B. (2000). Scaling up issues of CIGS solar cells. Thin Solid Films 361, 540–546. doi:10.1016/s0040-6090(99)00849-4
Presting, H., and König, U. (2003). Future nanotechnology developments for automotive applications. Mater. Sci. Eng. C 23, 737–741. doi:10.1016/j.msec.2003.09.120
Repins, I., Contreras, M., Romero, M., Yan, Y., Metzger, W., Li, J., et al. (2008). “Characterization of 19.9%-efficient CIGS absorbers,” in 2008 33rd IEEE photovoltaic specialists conference. IEEE, 1–6.
Rojas, D., Beermann, J., Klein, S., and Reindl, D. (2008). Thermal performance testing of flat-plate collectors. Sol. Energy 82, 746–757. doi:10.1016/j.solener.2008.02.001
Saeli, M., Piccirillo, C., Parkin, I. P., Ridley, I., and Binions, R. (2010). Nano-composite thermochromic thin films and their application in energy-efficient glazing. Sol. Energy Mater. Sol. Cells 94, 141–151. doi:10.1016/j.solmat.2009.08.010
Scavennec, A., Sokolich, M., and Baeyens, Y. (2009). Semiconductor technologies for higher frequencies. IEEE Microw. Mag. 10, 77–87. doi:10.1109/mmm.2008.931712
ScienceDaily (2012). Mobile electrons multiplied in quantum dot films. Available online at: http://www.sciencedaily.com/releases/2011/10/111014080041.htm⟩.
Serrano, E., Rus, G., and Garcia-Martinez, J. (2009). Nanotechnology for sustainable energy. Renew. Sustain. Energy Rev. 13, 2373–2384. doi:10.1016/j.rser.2009.06.003
Sharma, A., Tyagi, V. V., Chen, C. R., and Buddhi, D. (2009). Review on thermal energy storage with phase change materials and applications. Renew. Sustain. energy Rev. 13, 318–345. doi:10.1016/j.rser.2007.10.005
Shilei, L., Neng, Z., and Guohui, F. (2006). Impact of phase change wall room on indoor thermal environment in winter. Energy Build. 38, 18–24. doi:10.1016/j.enbuild.2005.02.007
Shockley, W., and Queisser, H. (2006). Detailed balance limit of efficiency of p–n junction solar cells. Renew. energy, Routledge2018. doi:10.1063/1.1736034
Soltani, M., Kashkooli, F. M., Souri, M., Rafiei, B., Jabarifar, M., Gharali, K., et al. (2021). Environmental, economic, and social impacts of geothermal energy systems. Renew. Sustain. Energy Rev. 140, 110750. doi:10.1016/j.rser.2021.110750
Souri, M., and Mojra, A. (2021). A nexus between active and passive control methods for reduction of aerodynamic noise of circular cylinder. Int. J. Mech. Sci. 200, 106446. doi:10.1016/j.ijmecsci.2021.106446
Souri, M., Moradi Kashkooli, F., Soltani, M., and Raahemifar, K. (2021). Effect of upstream side flow of wind turbine on aerodynamic noise: simulation using open-loop vibration in the rod in rod-airfoil configuration. Energies 14, 1170. doi:10.3390/en14041170
Staebler, D. L., and Wronski, C. (1977). Reversible conductivity changes in discharge-produced amorphous Si. Appl. Phys. Lett. 31, 292–294. doi:10.1063/1.89674
Tang, R., Yang, Y., and Gao, W. (2011). Comparative studies on thermal performance of water-in-glass evacuated tube solar water heaters with different collector tilt-angles. Sol. Energy 85, 1381–1389. doi:10.1016/j.solener.2011.03.019
Taniguchi, N. (1974). On the basic concept of'nano-technology. Proc. Intl. Conf. Prod. Eng. Tokyo, Part II, 1974, Jpn. Soc. Precis. Eng.
Tashakori-Asfestani, F., Kazemi, S., Zarghami, R., and Mostoufi, N. (2023). Effect of inter-particle forces on solids mixing in fluidized beds. Powder Technol. 415, 118098. doi:10.1016/j.powtec.2022.118098
Taylor, R. A., Otanicar, T., and Rosengarten, G. (2012). Nanofluid-based optical filter optimization for PV/T systems. Light Sci. and Appl. 1, e34. doi:10.1038/lsa.2012.34
Taylor, R. A., Phelan, P. E., Otanicar, T. P., Walker, C. A., Nguyen, M., Trimble, S., et al. (2011). Applicability of nanofluids in high flux solar collectors. J. Renew. Sustain. Energy 3. doi:10.1063/1.3571565
Tripanagnostopoulos, Y. (2007). Aspects and improvements of hybrid photovoltaic/thermal solar energy systems. Sol. energy 81, 1117–1131. doi:10.1016/j.solener.2007.04.002
Tyagi, H., Phelan, P., and Prasher, R. (2009). Predicted efficiency of a low-temperature nanofluid-based direct absorption solar collector. J. Sol. Energy Eng. 131. doi:10.1115/1.3197562
Urbina, A. (2020). The balance between efficiency, stability and environmental impacts in perovskite solar cells: a review. J. Phys. Energy 2, 022001. doi:10.1088/2515-7655/ab5eee
Villar, N. M., Lopez, J. C., Muñoz, F. D., García, E. R., and Andrés, A. C. (2009). Numerical 3-D heat flux simulations on flat plate solar collectors. Sol. energy 83, 1086–1092. doi:10.1016/j.solener.2009.01.014
Vrielink, J., Tiggelaar, R. M., Gardeniers, J. G., and Lefferts, L. (2012). Applicability of X-ray fluorescence spectroscopy as method to determine thickness and composition of stacks of metal thin films: a comparison with imaging and profilometry. Thin Solid Films 520, 1740–1744. doi:10.1016/j.tsf.2011.08.049
Wang, X.-Q., and Mujumdar, A. S. (2008). A review on nanofluids-part I: theoretical and numerical investigations. Braz. J. Chem. Eng. 25, 613–630. doi:10.1590/s0104-66322008000400001
Wang, Z.-S., Kawauchi, H., Kashima, T., and Arakawa, H. (2004). Significant influence of TiO2 photoelectrode morphology on the energy conversion efficiency of N719 dye-sensitized solar cell. Coord. Chem. Rev. 248, 1381–1389. doi:10.1016/j.ccr.2004.03.006
Werner, J. H. (2012). Second and third generation photovoltaics--dreams and reality. Adv. Solid State Phys., 51–66. doi:10.1007/978-3-540-39970-4_5
Wijewardane, S., and Goswami, D. (2012). A review on surface control of thermal radiation by paints and coatings for new energy applications. Renew. Sustain. Energy Rev. 16, 1863–1873. doi:10.1016/j.rser.2012.01.046
Xie, Y.-L. (2013). Enhanced photovoltaic performance of hybrid solar cell using highly oriented CdS/CdSe-modified TiO2 nanorods. Electrochimica Acta 105, 137–141. doi:10.1016/j.electacta.2013.04.157
Yan, X., Li, B., and Li, L.-s. (2013). Colloidal graphene quantum dots with well-defined structures. Accounts Chem. Res. 46, 2254–2262. doi:10.1021/ar300137p
Yin, Z., Sun, S., Salim, T., Wu, S., Huang, X., He, Q., et al. (2010). Organic photovoltaic devices using highly flexible reduced graphene oxide films as transparent electrodes. ACS nano 4, 5263–5268. doi:10.1021/nn1015874
Yousefi, T., Shojaeizadeh, E., Veysi, F., and Zinadini, S. (2012). An experimental investigation on the effect of pH variation of MWCNT–H2O nanofluid on the efficiency of a flat-plate solar collector. Sol. Energy 86, 771–779. doi:10.1016/j.solener.2011.12.003
Zhang, C., Chen, Y., Wu, L., and Shi, M. (2011). Thermal response of brick wall filled with phase change materials (PCM) under fluctuating outdoor temperatures. Energy Build. 43, 3514–3520. doi:10.1016/j.enbuild.2011.09.028
Zhang, D., Li, X., Chen, S., Tao, F., Sun, Z., Yin, X., et al. (2010). Fabrication of double-walled carbon nanotube counter electrodes for dye-sensitized solar sells. J. Solid State Electrochem. 14, 1541–1546. doi:10.1007/s10008-009-0982-3
Zhang, L., Ding, Y., Povey, M., and York, D. (2008). ZnO nanofluids–A potential antibacterial agent. Prog. Nat. Sci. 18, 939–944. doi:10.1016/j.pnsc.2008.01.026
Zhang, Q., and Cao, G. (2011). Nanostructured photoelectrodes for dye-sensitized solar cells. Nano today 6, 91–109. doi:10.1016/j.nantod.2010.12.007
Keywords: solar energy, nanotechnology, energy efficiency, photovoltaic cells, renewable energy
Citation: Farajollahi A (2025) Nanotechnology in solar energy: From active systems to Advanced Solar cells. Front. Energy Res. 13:1560718. doi: 10.3389/fenrg.2025.1560718
Received: 14 January 2025; Accepted: 28 March 2025;
Published: 08 April 2025.
Edited by:
Abhishek Saxena, Dev Bhoomi Uttarakhand University, IndiaReviewed by:
Slavica Prvulovic, University of Novi Sad, SerbiaSibin Kunhi Purayil, Virginia Tech, United States
Kaushalya Thopate, Savitribai Phule Pune University, India
Copyright © 2025 Farajollahi. This is an open-access article distributed under the terms of the Creative Commons Attribution License (CC BY). The use, distribution or reproduction in other forums is permitted, provided the original author(s) and the copyright owner(s) are credited and that the original publication in this journal is cited, in accordance with accepted academic practice. No use, distribution or reproduction is permitted which does not comply with these terms.
*Correspondence: Amirhamzeh Farajollahi, YS5mYXJham9sbGFoaUBzaGFyaWYuZWR1