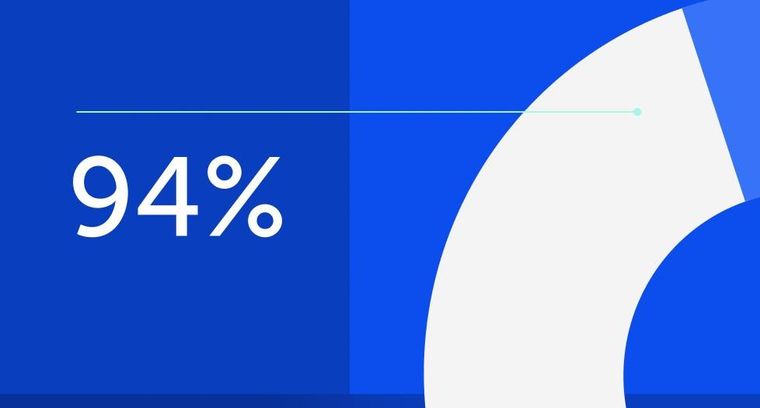
94% of researchers rate our articles as excellent or good
Learn more about the work of our research integrity team to safeguard the quality of each article we publish.
Find out more
METHODS article
Front. Energy Res., 25 March 2025
Sec. Solar Energy
Volume 13 - 2025 | https://doi.org/10.3389/fenrg.2025.1550153
This article is part of the Research TopicAdvanced Water Splitting Technologies Development: Best Practices and Protocols Volume IIView all 7 articles
Photoelectrochemical (PEC) water splitting is a promising technology for green hydrogen production by harnessing solar energy. Traditionally, this sustainable approach is studied under light intensity of 100 mW/cm2 mimicking the natural solar irradiation at the Earth’s surface. Sunlight can be easily concentrated using simple optical systems like Fresnel lens to enhance charge carrier generation and hydrogen production in PEC water splitting. Despite the great potentials, this strategy has not been extensively studied and faces challenges related to the stability of photoelectrodes. To prompt the investigations and applications, this work outlines the best practices and protocols for conducting PEC solar water splitting under concentrated sunlight illumination, incorporating our recent advancements and providing some experimental guidelines. The key factors such as light source calibration, photoelectrode preparation, PEC cell configuration, and long-term stability test are discussed to ensure reproducible and high performance. Additionally, the challenges of the expected photothermal effect and the heat energy utilization strategy are discussed.
Hydrogen (H2), produced from photocatalytic water splitting, is considered a clean energy and can offer a sustainable alternative to fossil fuels (Li Y. et al., 2024; Zhou et al., 2023; Li Z. et al., 2023). Since the 1970s, photoelectrochemical water splitting has garnered significant attention, owing to the ability to convert solar energy to hydrogen via utilizing photoanode||cathode, anode||photocathode, or photoanode||photocathode tandem configuration systems (Liu et al., 2023; Kim et al., 2016; Hisatomi et al., 2014a; Vanka et al., 2020; Wang S. et al., 2019). The photoelectrochemical process operates from the absorption of sunlight by semiconductor, generating electron-hole pairs to drive chemical reaction (Yao et al., 2018; Yang et al., 2013). The maximum photocurrent density achievable with these semiconductor photoelectrodes is limited by the generation of photogenerated electron-hole pairs, leading to the low hydrogen production rate and solar energy conversion efficiency (Luna et al., 2017; Bell et al., 2013).
Concentrated solar light irradiation offers a potential strategy to this limitation by increasing the number of photoexcited charge carriers, thereby improving photocurrent density and solar-to-chemicals conversion (e.g., H2 production and CO2 reduction). This approach has been demonstrated to be effective in various photovoltaic-electrocatalysis (PV-EC) devices (Yamaguchi et al., 2023; Calnan et al., 2022), providing the added benefits to reduce the costs of metal catalysts, semiconductor, and electricity. Nakamura et al. achieved a 24.4% solar-to-H2 (STH) conversion efficiency under concentrated sunlight irradiation (70 suns or 7 W/cm2) by integrating high-efficiency InGaP/GaAs/Ge three-junction PV cell and polymer-electrolyte EC cell (Nakamura et al., 2015). Bonke et al. reported a solar-to-chemical conversion efficiency of 22.4% under 100 suns illumination with the utilization of commercial InGaP/GaAs/Ge PV cell and earth-abundant Ni-based electrocatalysts, (Bonke et al., 2015), and the system could effectively operate in actual river water and various geographic locations. Besides, Jia and co-workers improved the STH efficiency in a similar PV-EC system to >30% with stability longer than 48 h under 42 suns light irradiation, through using two series-connected polymer electrolyte membrane electrolyzers (Figure 1A). (Jia et al., 2016) More importantly, Gentle et al. set up a kW-scale (concentrated solar light intensity) thermally integrated photo-electrochemical device for H2 production with rate of 0.8 g/min and STH efficiency of 20%, (Holmes-Gentle et al., 2023), which experimentally demonstrated an encouraging step towards the potential commercialization of PV-EC concept. However, the practical applications of the PV-EC solar water splitting technology face several shortcomings, such as system complexity, high investment cost, energy loss, and instability.
Figure 1. (a) PV-EC reaction system consisted of triple-junction InGaP/GaAs/Ge solar cell and two PEM electrolyzers. Reproduced with permission from Jia et al. (2016). (b) Photograph of the integrated PEC system for HER (top left) and OER (top right) and the schematic (bottom) illustration of the PEC testing setup. Reproduced with permission from Ziani et al. (2020).
The direct photoelectrochemistry with the use of monolithic photoelectrodes, especially photocathode, is a more promising approach for clean hydrogen production from water. Photoelectrochemical reactions can convert solar energy into chemical energy through catalytic processes, typically involving semiconductors and cocatalysts (Qureshi and Tahir, 2024). Compared to the PV-EC interconnected system, the PEC configuration system simplifies the overall design by integrating sunlight absorption and electrochemical reaction within a single device, reducing the need for additional wiring and power management components. Furthermore, the developed PEC electrodes enable direct charge transfer at the semiconductor-electrolyte interface, minimizing resistive losses and enhancing energy conversion efficiency (Wedege et al., 2018; Hisatomi et al., 2014b). This integration also allows for a more compact and potentially lower-cost system. Additionally, PEC systems can leverage tailored semiconductor architectures to optimize light harvesting, reaction kinetics, and catalytic performance, further improving solar-to-hydrogen conversion efficiency. In this context, significant progress has been made in photoelectrochemical solar water splitting through developing and optimizing GaN nanowires grown on p-n+ Si photocathodes (Wang et al., 2019b; Wang et al., 2019c; Fan et al., 2019; Chowdhury et al., 2018). To reduce the cost of configuration, increase H2 production, and prompt large-scale application of PEC water splitting, employing concentrated sunlight illumination would be an effective and promising strategy (Wang et al., 2022). However, at present, the photoelectrochemical energy conversion and system stability under concentrated solar light illumination is rarely explored. To date, Vilanova et al. studied the solar water splitting using 200 cm2 PEC cell under concentrated sunlight irradiation (1.28 W/cm2), where the experiments were conducted outdoor in flow-type operation mode, but the H2 yield rate only reached about 5.6 × 10−2 mg/h/cm2 (Vilanova et al., 2020). Ziani et al. developed a wireless integrated three-junction InGaP/GaAs/Ge-based photoelectrode, which was decorated with Pt nanoparticles and Ni foil on the front and back side, respectively (Figure 1B). (Ziani et al., 2020) Under 39 suns illumination, the stoichiometric amounts of H2 and O2 were produced and the STH efficiency was estimated to be ∼12%. Very recently, we have systematically investigated PEC solar water splitting in a custom-designed flow cell reactor using Pt/GaN/Si photocathode under concentrated solar light illumination (Dong et al., 2023; Dong et al., 2024). The results showed that the saturated photocurrent density, onset potential, and H2 yield rate could be dramatically increased with strengthening light irradiation intensity. Meanwhile, both the large photocurrent density and onset potential can maintain for over 1,500 h under 6.4 suns light illumination. However, while PEC water splitting has made notable progress in terms of long-term stability and scaling-up over the past decades (Bae et al., 2017; Vilanova et al., 2018; Hisatomi et al., 2024), PEC-based CO2 and N2 reduction remain at a lower technology readiness level. Moreover, investigations into PEC-driven solar water splitting, CO2 reduction, and N2 fixation under concentrated sunlight are still in their early stages, requiring further systematic studies to elucidate underlying mechanisms, address existing challenges, and accelerate practical applications.
However, the investigations on PEC solar water splitting, CO2 reduction, and N2 fixation under concentrated sunlight are in the infancy, which require further deep and systematical studies to understand the mechanisms, mitigate challenges, and prompt practical application.
It should be noted that, under concentrated solar light irradiation, the vigorous generation of hydrogen bubbles can induce surface tension to detach co-catalyst nanoparticles from semiconductor absorber (Du and Zheng, 2024; Kim et al., 2023). Additionally, the rapid consumption of protons near photocathode and hydroxyl anions near photoanode can significantly alter the local pH and reaction microenvironments (Monteiro et al., 2021; Zhu et al., 2024; Chen et al., 2024). These changes near photoelectrode/electrolyte interface can lead to unwanted photocorrosion, byproducts formation, and inhibitions on reaction kinetics. More importantly, the concentrated solar light illumination also can intensify temperatures near/on the photoelectrode surface, leading to severe adverse effects like photoelectrode structural evolution and degradation, side reactions, and membrane breakage, which will negatively influence photoelectrochemical performance. Therefore, understanding these material, chemical, and physical phenomena under concentrated solar light illumination during PEC operation process is essential and urgently needed.
In this work, we first analyze the advantages and limitations associated with various photoelectrode configurations, providing insights into how different designs impact performance and stability. Based on that, we propose that the effective flow cell designs are optimal for high gas evolution rates under concentrated solar light irradiation. Then, we detail the experimental procedures and methodologies necessary for implementing concentrated sunlight in photoelectrochemical system. Furthermore, the critical components, materials, measurement tools, and analytical equipment required for reliable and accurate results were discussed. For clarity, Pt/GaN/Si photocathode for photoelectrochemical hydrogen evolution under concentrated sunlight was presented as an example to show recent advancements and practical applications. Finally, the challenges and potentials associated with photothermal effects are explored, offering a detailed outlook on the future of concentrated sunlight in photoelectrochemical water splitting.
As illustrated in Figure 2, the photoelectrochemical cells for water splitting can be characterized by their division into two separate reaction compartments by an ion-exchange membrane. The implement of membrane is a simple and critical strategy to make the hydrogen evolution reaction (HER) and oxygen evolution reaction (OER) occur separately, preventing the recombination of H2 and O2 and ensuring the purity of hydrogen and safety of the reaction system (Minggu et al., 2010; Wang et al., 2016). In the following contents, we primarily focus on the configuration of photocathode for HER.
Figure 2. Classification of monolithic photoelectrochemical water splitting systems by photocathode configuration.
The photocathode can be immersed in electrolyte and is composed of a semiconductor light absorber overlaid with co-catalyst, and the light irradiation is from the front side (in other words, the light firstly reaches co-catalyst, as shown in Figure 2A). The role of photocathode is to absorb incident light for generating charge carriers to participate in catalytic reactions. The electrons produced at the conduction band of semiconductor migrate towards the co-catalyst layer and drive hydrogen evolution reaction, leading to the production of hydrogen. Simultaneously, the photogenerated holes in the valance band of semiconductor transport to anode situated in the anodic compartment, where they facilitate the oxygen evolution reaction (Yao et al., 2018; Peter et al., 2016). Therefore, the complete electrical circuit achieves overall solar water splitting in a continuous operational mode. To enable the effective function of photocathode, the substrate should be passivated using epoxy, except for the active surface area decorated by co-catalysts. The passivation is crucial as it prevents unwanted side reactions and protects the semiconductor from chemical corrosion by electrolyte. Electrical connection to the external circuitry is established at the back side of the substrate, often employing GaIn eutectic and Ag paste. However, a noticeable issue arises from the potential diffusion of these materials into the electrolyte over time, which may lead to contamination of the co-catalyst surface and membrane and impact the HER efficiency. Another critical issue pertains to the long-term chemical and physical stability of the passivation layer. Epoxy used to encapsulate photoelectrodes can degrade over extended periods, making electrolyte infiltration that may cause semiconductor corrosion or disruption of electrical connections. Additionally, the long-term exposure can result in carbonaceous impurities from epoxy accumulating on the photoelectrode surface, negatively affecting photoelectrochemical performances (Bae et al., 2019). Furthermore, the semi-transmitting nature of thin epoxy layers can lead to overestimated PEC performance due to unintended light absorption and scattering effects (Döscher et al., 2014). These influences highlight the potential limitations of using epoxy in PEC reaction system, necessitating further material selection and encapsulation strategies for long-term stability and accurate estimation on performance. Besides, because the incident light needs to pass through the co-catalyst layer, some of light may be absorbed or reflected, thereby reducing the number of photons that reach the semiconductor substrate. This effect may suppress the electron-hole pairs generation and thus leading to a decrease in photocurrent density and an inhibition in hydrogen production. Despite these shortcomings and challenges, the simple preparation process of this type of photoelectrode configuration makes it a widely adopted approach in laboratory studies. Researchers can easily test and optimize the performance of new semiconductor materials and co-catalysts for photoelectrochemical water splitting using this setup.
An alternative configuration is illustrated in Figure 2B, in which the incident light directly irradiates the semiconductor light absorber and the catalytic reactions occur on the opposite side of the substrate where co-catalyst is loaded (Vilanova et al., 2024; Fu et al., 2020). This type of photoelectrode configuration decouples the light absorption from the catalytic reaction, as the light irradiation does not need to pass through the co-catalyst layer. This configuration setup allows for the formation of co-catalysts with various thicknesses and nanostructures without being constrained by their optical properties such as transparency, reflectivity, and absorption. The advantage of this configuration is the ability to optimize both the semiconductor light absorber and the co-catalyst independently. Since the co-catalyst does not interfere with the light absorption, the efficient light harvesting can be achieved to increase the number of electron-hole pairs, thus improving the overall photocurrent density and hydrogen production rate. However, the semiconductor substrate must be thoroughly protected from corrosion in aqueous environment, not only on its sides but also on the surface where the light is incident. This requirement imposes the need for a transparent passivation material to avoid the inference on light transmission and absorption. In addition, the charge transport distance should also be considered in this type of PEC configuration system. The longer charge transport distances can lower charge collection efficiency, which becomes particularly critical for thick c-Si electrodes. Thinning c-Si to the micrometer scale (e.g., ∼5 µm) can help mitigate this issue by reducing transport losses, though it also introduces trade-offs in light absorption. This issue becomes even more significant when using light absorbers with lower charge carrier mobility. Thus, the balance between light absorption and charge transfer should be considered to improve the solar energy conversion efficiency under optimized conditions.
Moreover, as depicted in Figure 2C, the semiconductor substrate could be located outside of the aqueous solution, while the co-catalyst layer is in contact with the electrolyte (Peerakiatkhajohn et al., 2017). This configuration, though similar to the PV-EC system, differs in that it features a monolithic single-device structure rather than two separate devices connected via external electrical wiring. In this configuration, the light absorption and catalytic reactions are spatially separated and decouplable. Additionally, the semiconductor materials are not in contact with aqueous solution and there is no need for passivation of the substrate with epoxy. However, the possible leakage of liquid electrolyte from the reactor should be paid attention. To address this issue, rubber O-rings or corrosion-resistant gaskets can be employed. Although the initial design and fabrication of the reaction system can be somewhat challenging, the successful construction will allow for the convenient evaluation of the photoelectrode without concerns about the diffusion of unwanted materials from epoxy and contacts. The solar-to-fuel conversion efficiency of various photoelectrochemical systems has been well studied and reviewed (Peerakiatkhajohn et al., 2017; Yi et al., 2018; Jang and Lee, 2019; Yang and Moon, 2019), which can provide insightful guidance onto future research and the determination on system configuration.
The static H-type electrolysis cell is a traditional setup for photoelectrochemical water splitting study, comprising two separate compartments connected by an ion exchange membrane and external wires (Figure 3A). (Liu et al., 2019; Liu et al., 2020) This design effectively isolates the anodic and cathodic reactions, reduces cross-contamination, and allows precise control under various experimental conditions. However, under concentrated solar light illumination, the photocurrent density in the static electrolysis cell can easily surpass the mass transport limit, making it constrained by the transport of reactants and products rather than by light intensity, photogenerated charge carrier separation, and catalytic activity, thus leading to the decreased H2 evolution efficiency (Chen et al., 2021; Chen et al., 2023). Additionally, the accumulation of hydrogen gas bubbles on the photocathode can reduce the effective surface area, suggesting the efficient detachment of hydrogen bubbles is important, especially under concentrated solar light illumination. Furthermore, in the sealed reaction chamber, the rapid evolution of hydrogen, which occurs at twice the rate of oxygen evolution, could increase the gas pressure in cathodic compartments, which may induce a risk of physical damage to the ion exchange membrane.
Compared to the static cells that suffer from pressure accumulation and mass transfer limit, flow cell is a more advantageous option, particularly when gas production rates are extremely high under concentrated solar light. The first type of flow cell has a water inlet at the bottom of the chamber where the reaction solution is continuously supplied to the cell, and a gas outlet located at the top of the chamber that allows the generated hydrogen and oxygen to spontaneously exit from the chamber to maintain the pressure (Figure 3B). However, it is significant and difficult that the amount of water supplied should equal to the amount of consumed to maintain the optimal reaction conditions. Excessive water input can lead to overflow through the gas outlet, causing operational issues, while insufficient water input can lower the liquid level, potentially exposing the photoelectrode to ambient environment. In addition, the relatively slow rate of water input still limits the effectiveness of mass transport.
Another type of flow cell is illustrated in Figure 3C, which contains an inlet for fresh solution and an outlet for solution circulation, and the whole space of the chamber is filled with electrolyte (Minggu et al., 2010; Liu et al., 2022). This configuration enables continuous refreshment of electrolyte and the maintenance of optimal conditions. Through keeping the chamber filled with electrolyte, this setup solves problems related to gas accumulation, mass transport, and concentration gradients. The flexibility to adjust the flow rate of both incoming and outgoing electrolyte allows for precise control of reaction conditions during photoelectrochemical process. The high flow rate enhances mass transport and reaction efficiency and also could rapidly remove hydrogen bubbles adsorbed on photoelectrode surface. However, it also means that a substantial amount of unreacted electrolyte is expelled. Additional equipment should be used to recycle the electrolyte for further reaction.
The procedures for photoelectrochemical solar water splitting under concentrated sunlight irradiation can be divided into sequential steps that require specific actions and decisions (Figure 4), as described following:
Figure 4. Flowchart illustrating the steps for the concentrated solar light photoelectrochemical water splitting.
The fabrication of photoelectrode involves several key processes, including semiconductor material growth, co-catalyst synthesis, and photoelectrode preparation. The semiconductor materials are grown or purchased, followed by the deposition of co-catalysts onto the semiconductor substrates using physical vapor deposition, photodeposition, or electrodeposition. The obtained photoelectrode is then carefully assembled by making electrical contacts and applying protective epoxy. These steps should ensure the optimal light absorption, efficient charge carrier transfer and separation, and chemical stability.
Once the photoelectrode is prepared, it will be mounted onto the flow cell, which should ensure that all the components are gas-tight and liquid-tight to prevent leakage. The reaction cell is then subjected to a calibration process, where the light intensity is adjusted to meet the experimental requirements. This step involves aligning the photoelectrode within the flow cell to ensure the uniform exposure to concentrated solar light.
Linear sweep voltammetry (LSV) and/or cyclic voltammetry (CV) are performed to assess the photoelectrochemical performances. For the LSV test, the potential is varied linearly and the resulting photocurrent response is monitored, providing information on the optoelectronic and catalytic performance of photoelectrode. CV measurement sweeps in the range of two limited potentials and records the photocurrent response to understand the redox processes and stability of the photoelectrode. Additionally, the LSV and CV tests are also measured in dark to reveal any background current that occurs in electrolysis process, allowing for more accurate evaluation of the photoelectrocatalytic performance. Data from these measurements are saved for subsequent analyses.
Chronoamperometry (CA) and/or chronopotentiometry (CP) measurements are conducted under constant voltage or constant current conditions to evaluate the catalytic performance of photoelectrode and to explore the long-term stability. Gas chromatography (GC) is employed to analyze the composition and quantity of the gaseous products evolved during photoelectrochemical reaction. The data from CA, CP, and GC are systematically recorded and stored for the calculation of Faradaic efficiency and H2/O2 production rate.
The photoelectrochemical stability of the photoelectrode could be assessed based on the data collected from LSV, CV, CA, and CP. If the performance is stable, consecutive measurements of LSV, CV, CA, and CP are carried out to monitor ongoing performance. If instability is observed, potential issues such as light intensity variations, flow rate discrepancies, gas and liquid leakage, and the structural change of photoelectrode should be checked and corrected.
After completing the stability test, the photoelectrode could be subjected to detailed material characterizations to analyze any structural changes or degradation. Scanning electron microscopy (SEM) provides images of the surface morphology, transmission electron microscopy (TEM) offers insights into microstructure and compositions, and X-ray photoelectron spectroscopy (XPS) reveals information about surface composition and chemical states. These analyses are crucial for understanding the material’s behavior and identifying the degradation mechanisms.
Based on the results obtained from performance tests and material characterizations, detailed analysis of the reaction mechanisms and experimental issues is conducted. The analyses aim to identify the reasons behind any observed performance degradation or instability. Potential strategies for improvements are proposed to optimize the catalytic system, which may include modifications on the photoelectrode, adjustments in experimental conditions, and optimizations on the flow cell design.
Based on the above-mentioned analysis and proposed solutions, the process is iterated by fabricating a new photoelectrode incorporating the identified improvements or modifications.
The flow cell is a crucial component in photoelectrochemical experiments for continuous flow of electrolyte over photoelectrode. The flow cell typically includes inlet and outlet ports for electrolyte, ensuring the steady supply of reactants and the removal of products. The flow cell also integrates features for effective light irradiation and includes components to ensure the gas and liquid tightness.
The liquid circulation pump is used to maintain continuous flow of electrolyte throughout the flow cell. The electrolyte should be evenly distributed over the photoelectrode for prompting the removal of any reaction products. The flow rate can be adjusted to optimize the reaction conditions and improve mass transfer.
The light source, typically a lamp or laser, provides illumination for the photoelectrochemical reaction. In addition, optical lenses can be used to concentrate the light and increase irradiation intensity, and filters can be applied to adjust the wavelength to satisfy specific experiments.
Potentiostat is an instrument used to control the voltage applied to the photoelectrode and record the resulting photocurrent, allowing for the assessment of various photoelectrochemical properties, such as LSV, CV, CP, and CA. Moreover, the electrochemical impedance, providing insights into the resistance and dynamic behavior of charge carriers, can be analyzed using potentiostat.
The gas chromatograph (GC) is used for analyzing the composition and quantity of gases produced during photoelectrochemical reaction. The instrument separates and quantifies different gaseous components, such as hydrogen and oxygen, providing crucial data for determining the efficiency, productivity, and selectivity of the reaction system.
SEM could provide high-resolution images for analyzing the surface morphology of the photoelectrode. It uses a focused beam of electrons to scan the surface to reveal the structural features, particle size, and surface defects.
By transmitting electrons through samples, TEM provides detailed images of microstructural features and can reveal information about the crystallinity, phase distribution, and interface characteristics.
XPS analyzes the surface composition and chemical states of the photoelectrode through measuring the binding energies of photoelectrons emitted from the surface when irradiated with high-energy X-rays, which could provide detailed information on the elemental composition and chemical bonding.
To fabricate the Pt-decorated GaN/Si photocathode, n+-p Si wafer was prepared firstly through thermal diffusion process with phosphorus and boron as dopants, followed by growing GaN nanowires on the wafer using plasma-assisted molecular beam epitaxy. The GaN/Si samples were then subjected to Pt co-catalyst decoration by photodepostion method. In detail, the solution containing chloroplatinic acid, water, and methanol was illuminated to generate Pt nanoparticles on GaN nanowires. Afterwards, back contacts were prepared using GaIn eutectic alloy and Cu wire for preparing photoelectrode. Photoelectrochemical measurements under natural and concentrated solar light were conducted using simulated solar light to drive hydrogen evolution reaction and oxygen evolution reaction in H-type flow cell (Figure 5), with Pt wire as the counter electrode and Ag/AgCl as the reference electrode. The Nafion 117 membrane was used as proton exchange membrane and 0.5 M H2SO4 electrolyte was circulated at a flow rate of 10 mL/min. LSV curves were measured after each period of CA measurement. Redeposition of Pt co-catalyst was performed after each CA measurement to assess chemical stability and high performance. Characterizations including SEM, TEM, and XPS were performed to evaluate the material properties and microstructure.
Figure 5. Schematic illustration of H-type flow cell utilized for photoelectrochemical solar water splitting under concentrated solar light. Reproduced with permission from Dong et al. (2024).
The as-prepared Pt/GaN/Si photocathode was then loaded into a flow-cell chamber, and LSV curve was measured. Subsequently, the photocurrent density was recorded at 0 V vs reversible hydrogen electrode (VRHE) through CA for long term stability measurements, followed by another LSV measurement. The processes were repeated, and when noticeable degradation in performance was observed during CA measurement, the experiment was stopped and the Pt/GaN/Si photoelectrode was removed from the chamber for additional Pt co-catalyst photodeposition. The LSV and CA measurements were then repeated for many times. As shown in Figure 6A, the comparison of LSV curves between pristine (0 h) and 24 h reacted Pt/GaN/Si showed a clear degradation in performance. The performance degradation even after the redeposition of Pt is often attributed to the inability of contaminated photoelectrode surfaces to firmly anchor the Pt nanoparticles during resumed stability testing (Bae et al., 2019). In our study, notably, we found that the high performance was recovered after Pt redeposition. More interestingly, after the fifth redeposition of Pt co-catalysts, the stability significantly improved (Figure 6B). Furthermore, there was no noticeable degradation in photocurrent density after the seventh Pt redeposition and the excellent performance stability was achieved in consequence, with onset potential >0.5 VRHE, photocurrent density at 0 VRHE > 60 mA/cm2, and Faradaic efficiency of hydrogen >97%. Moreover, the photoelectrochemical stability was maintained over 1,500 h under concentrated solar light (640 mW/cm2). The improved stability on PEC water splitting via Pt redeposition was due to the epitaxial growth of Pt NPs on GaN NWs.
Figure 6. (a) LSV and (b) CA results of photoelectrochemical water splitting under concentrated solar light (640 mW/cm2). TEM and EDS elemental map of (c, d) pristine Pt/GaN/Si photocathode, (e) after 24 h reaction, and (f) after 288 h reaction with 5 times of Pt redeposition. Reproduced with permission from Dong et al. (2024).
The morphology of Pt/GaN/Si photoelectrode was characterized by using high-angle annular dark-field scanning transmission electron microscopy (HAADF-STEM). Figures 6C, D showed that, before chemical reaction, Pt nanoparticles deposited on the top and sidewalls of GaN nanowires with homogeneous distribution. After 24 h of reaction under concentrated sunlight, some Pt nanoparticles were detached and smaller Pt nanoparticles sparsely distributed on the GaN nanowire (Figure 6E). Notably, after five times of Pt redeposition and 288-h test, a substantial number of Pt nanoparticles with larger size remained on GaN nanowires (Figure 6F), indicating the in-situ growth during redeposition process. In addition, the performance degradation was significantly faster under concentrated solar light compared to that under natural sunlight. Analysis implies that concentrated sunlight caused rapid detachment of Pt nanoparticles likely due to the vigorous hydrogen gas evolution. However, the redeposition of Pt can improve HER activity and stability. These results underscore that the stable bonding of Pt co-catalysts on GaN nanowires is beneficial to efficient and stable PEC water splitting under concentrated solar light irradiation.
Under very strong solar light irradiation (4,000 mW/cm2), the photocurrent density at 0 VRHE increased to over 240 mA/cm2 (Figure 7). However, the rubber O-rings in the flow cell were damaged by photothermal heating effect after 52 h. To enable stable operation for concentrated solar light water splitting, the reactor design and construction will need careful adjustments. Despite these stability issues, the photothermal effect caused by concentrated sunlight irradiation can significantly enhance reaction kinetics on the photoelectrode surface. Although the photothermal catalysis has been extensively investigated in various fields including photocatalytic water splitting, CO2 reduction, and N2 fixation, as well as in gas-phase reactions, (Zhou et al., 2023; Zhao et al., 2022; He et al., 2021; Li J. et al., 2023; Zhang et al., 2021; Li J. et al., 2024; Ghoussoub et al., 2019), the corresponding impact on photoelectrochemical water splitting is rarely explored. Understanding how the localized high temperatures affect the photoelectrode surface and the catalytic reactions will be critical, because developing promising strategies to combine the photo- and thermal-energies probably could improve hydrogen production efficiency compared to the conventional photoelectrochemical water splitting under mild temperature.
Figure 7. Photocurrent density decrease due to reactor chamber malfunction caused by damage to rubber O-rings from photothermal heating under highly concentrated light (∼4,000 mW/cm2). Reproduced with permission from Dong et al., (2024).
This study suggests general protocols and guidelines on performing the photoelectrochemical solar water splitting under concentrated sunlight, including the aspects on photoelectrode preparation and characterization, reaction chamber design, light source calibration, photoelectrochemical measurement, and long-term stability test. Systematic framework and standard procedures for evaluating the catalytic performance and chemical/structural evolution were proposed, and the recent advancements based on Pt/GaN/Si photoelectrode were presented as an example to show how the protocols could be correctly conducted to measure the activity and stability and to understand the related challenges and mechanisms. The descriptions and discussions could pave an avenue for studying the photoelectrochemical solar energy conversion using concentrated sunlight to improve the solar chemicals production and to prompt the practical applications, through simultaneously achieving the ultra-high stability and excellent efficiency.
At present, the photoelectrochemical studies under concentrated sunlight have not garnered much attention and this technology still faces challenges like localized heating effect, bench-scale investigation, and high cost. On the other hand, the concentrated sunlight irradiation could rapidly increase the temperature around the photoelectrode, therefore, it is of great promise to improve the PEC reaction efficiency through properly utilizing the photothermal effect. Nevertheless, note that the potential high temperature may damage the reaction chamber and the photoelectrode and even may cause safety issue. To address these issues, the use of a water bath system to control the temperature and the use of heat resisting materials to construct the flow cell would be feasible approaches. On the other hand, the efficiency and stability of tandem photoelectrochemical cell under concentrated sunlight should be investigated. which could operate under bias-free conditions. This reaction system contains photoanode and photocathode, and the light irradiation first illuminates the semiconductor with narrower band gap. Notably, the substrate for semiconductor light absorber growth should be transparent and the co-catalyst layer should not be too thick to influence the light irradiation, and the reaction chamber should be designed to satisfy the experimental requirements. Further, there is a need to conduct the related measurements outdoor using the concentrated natural sunlight, although it may be more difficult than the indoor bench-scale test. This will build a platform to industrialize the photoelectrochemical solar energy conversion for the production of various valuable chemicals from water splitting, CO2 reduction, N2 fixation, and biomass upgrading.
The original contributions presented in the study are included in the article/supplementary material, further inquiries can be directed to the corresponding author.
KL: Writing–original draft. WD: Writing–original draft. ZM: Writing–review and editing.
The author(s) declare that financial support was received for the research and/or publication of this article. The authors gratefully acknowledge research support from the HydroGEN Advanced Water Splitting Materials Consortium, established as part of the Energy Materials Network under the U.S. Department of Energy, Office of Energy Efficiency and Renewable Energy, Fuel Cell Technologies Office, under Award Number DE-EE0010730, from US Army Research Office under Award Number W911NF2110337, and from National Science Foundation under Award Number 2330525.
Some IP related to this work was licensed to NX Fuels, Inc., which was co-founded by Z. Mi. The University of Michigan and Mi have a financial interest in the company. The remaining authors declare that the research was conducted in the absence of any commercial or financial relationships that could be construed as a potential conflict of interest.
The author(s) declare that no Generative AI was used in the creation of this manuscript.
All claims expressed in this article are solely those of the authors and do not necessarily represent those of their affiliated organizations, or those of the publisher, the editors and the reviewers. Any product that may be evaluated in this article, or claim that may be made by its manufacturer, is not guaranteed or endorsed by the publisher.
Bae, D., Seger, B., Hansen, O., Vesborg, P. C., and Chorkendorff, I. (2019). Durability testing of photoelectrochemical hydrogen production under day/night light cycled conditions. ChemElectroChem 6 (1), 106–109. doi:10.1002/celc.201800918
Bae, D., Seger, B., Vesborg, P. C., Hansen, O., and Chorkendorff, I. (2017). Strategies for stable water splitting via protected photoelectrodes. Angew. Chem. Int. Ed. 46 (7), 1933–1954. doi:10.1039/C6CS00918B
Bell, S., Will, G., and Bell, J. (2013). Light intensity effects on photocatalytic water splitting with a titania catalyst. Int. J. Hydrogen Energy 38 (17), 6938–6947. doi:10.1016/j.ijhydene.2013.02.147
Bonke, S. A., Wiechen, M., MacFarlane, D. R., and Spiccia, L. (2015). Renewable fuels from concentrated solar power: towards practical artificial photosynthesis. Energy Environ. Sci. 8 (9), 2791–2796. doi:10.1039/C5EE02214B
Calnan, S., Bagacki, R., Bao, F., Dorbandt, I., Kemppainen, E., Schary, C., et al. (2022). Development of various photovoltaic-driven water electrolysis technologies for green solar hydrogen generation. Sol. RRL 6 (5), 2100479. doi:10.1002/solr.202100479
Chen, B., Li, B., Tian, Z., Liu, W., Liu, W., Sun, W., et al. (2021). Enhancement of mass transfer for facilitating industrial-level CO2 electroreduction on atomic Ni-N4 Sites. Adv. Energy Mat. 11 (40), 2102152. doi:10.1002/aenm.202102152
Chen, C., Jin, H., Wang, P., Sun, X., Jaroniec, M., Zheng, Y., et al. (2024). Local reaction environment in electrocatalysis. Chem. Soc. Rev. 53 (4), 2022–2055. doi:10.1039/D3CS00669G
Chen, D., Bai, H., Zhu, J., Wu, C., Zhao, H., Wu, D., et al. (2023). Multiscale hierarchical structured NiCoP enabling ampere-level water splitting for multi-scenarios green energy-to-hydrogen systems. Adv. Energy Mat. 13 (22), 2300499. doi:10.1002/aenm.202300499
Chowdhury, F. A., Trudeau, M. L., Guo, H., and Mi, Z. (2018). A photochemical diode artificial photosynthesis system for unassisted high efficiency overall pure water splitting. Nat. Commun. 9 (1), 1707. doi:10.1038/s41467-018-04067-1
Dong, W. J., Xiao, Y., Yang, K. R., Ye, Z., Zhou, P., Navid, I. A., et al. (2023). Pt nanoclusters on GaN nanowires for solar-asssisted seawater hydrogen evolution. Nat. Commun. 14 (1), 179. doi:10.1038/s41467-023-35782-z
Dong, W. J., Ye, Z., Tang, S., Navid, I. A., Xiao, Y., Zhang, B., et al. (2024). Concentrated solar light photoelectrochemical water splitting for stable and high-yield hydrogen production. Adv. Sci. 11, 2309548. doi:10.1002/advs.202309548
Döscher, H., Geisz, J. F., Deutsch, T. G., and Turner, J. (2014). Sunlight absorption in water-efficiency and design implications for photoelectrochemical devices. Energy Environ. Sci. 7 (9), 2951–2956. doi:10.1039/C4EE01753F
Du, L., and Zheng, W. (2024). Catalyst deactivation during water electrolysis: understanding and mitigation. Apl. Energy 2 (2). doi:10.1063/5.0191316
Fan, R., Mi, Z., and Shen, M. (2019). Silicon based photoelectrodes for photoelectrochemical water splitting. Opt. Express 27 (4), A51–A80. doi:10.1364/OE.27.000A51
Fu, H.-C., Varadhan, P., Tsai, M.-L., Li, W., Ding, Q., Lin, C.-H., et al. (2020). Improved performance and stability of photoelectrochemical water-splitting Si system using a bifacial design to decouple light harvesting and electrocatalysis. Nano Energy 70, 104478. doi:10.1016/j.nanoen.2020.104478
Ghoussoub, M., Xia, M., Duchesne, P. N., Segal, D., and Ozin, G. (2019). Principles of photothermal gas-phase heterogeneous CO2 catalysis. Energy Environ. Sci. 12 (4), 1122–1142. doi:10.1039/C8EE02790K
He, B., Jia, S., Zhao, M., Wang, Y., Chen, T., Zhao, S., et al. (2021). General and robust photothermal-heating-enabled high-efficiency photoelectrochemical water splitting. Adv. Mat. 33 (16), 2004406. doi:10.1002/adma.202004406
Hisatomi, T., Kubota, J., and Domen, K. (2014a). Recent advances in semiconductors for photocatalytic and photoelectrochemical water splitting. Chem. Soc. Rev. 43 (22), 7520–7535. doi:10.1039/C3CS60378D
Hisatomi, T., Kubota, J., and Domen, K. (2014b). Recent advances in semiconductors for photocatalytic and photoelectrochemical water splitting. Angew. Chem. Int. Ed. 43 (22), 7520–7535. doi:10.1039/C3CS60378D
Hisatomi, T., Wang, Q., Zhang, F., Ardo, S., Reisner, E., Nishiyama, H., et al. (2024). Photocatalytic water splitting for large-scale solar-to-chemical energy conversion and storage. Front. Sci. 2, 1411644. doi:10.3389/fsci.2024.1411644
Holmes-Gentle, I., Tembhurne, S., Suter, C., and Haussener, S. (2023). Kilowatt-scale solar hydrogen production system using a concentrated integrated photoelectrochemical device. Nat. Energy 8 (6), 586–596. doi:10.1038/s41560-023-01247-2
Jang, Y. J., and Lee, J. S. (2019). Photoelectrochemical water splitting with p-type metal oxide semiconductor photocathodes. ChemSusChem 12 (9), 1835–1845. doi:10.1002/cssc.201802596
Jia, J., Seitz, L. C., Benck, J. D., Huo, Y., Chen, Y., Ng, J. W. D., et al. (2016). Solar water splitting by photovoltaic-electrolysis with a solar-to-hydrogen efficiency over 30%. Nat. Commun. 7 (1), 13237. doi:10.1038/ncomms13237
Kim, J., Jung, S.-M., Lee, N., Kim, K.-S., Kim, Y.-T., and Kim, J. K. (2023). Efficient alkaline hydrogen evolution reaction using superaerophobic Ni nanoarrays with accelerated H2 bubble release. Adv. Mat. 35 (52), 2305844. doi:10.1002/adma.202305844
Kim, J. H., Kaneko, H., Minegishi, T., Kubota, J., Domen, K., and Lee, J. S. (2016). Overall photoelectrochemical water splitting using tandem cell under simulated sunlight. ChemSusChem 9 (1), 61–66. doi:10.1002/cssc.201501401
Li, J., Ding, L., Su, Z., Li, K., Fang, F., Sun, R., et al. (2023b). Non-lignin constructing the gas–solid interface for enhancing the photothermal catalytic water vapor splitting. Adv. Mat. 35 (45), 2305535. doi:10.1002/adma.202305535
Li, J., Sheng, B., Chen, Y., Yang, J., Wang, P., Li, Y., et al. (2024b). Utilizing full-spectrum sunlight for ammonia decomposition to hydrogen over GaN nanowires-supported Ru nanoparticles on silicon. Nat. Commun. 15 (1), 7393. doi:10.1038/s41467-024-51810-y
Li, Y., Zhou, H., Cai, S., Prabhakaran, D., Niu, W., Large, A., et al. (2024a). Electrolyte-assisted polarization leading to enhanced charge separation and solar-to-hydrogen conversion efficiency of seawater splitting. Nat. Catal. 7 (1), 77–88. doi:10.1038/s41929-023-01069-1
Li, Z., Fang, S., Sun, H., Chung, R.-J., Fang, X., and He, J.-H. (2023a). Solar hydrogen. Adv. Energy Mat. 13 (8), 2203019. doi:10.1002/aenm.202203019
Liu, B., Wang, S., Zhang, G., Gong, Z., Wu, B., Wang, T., et al. (2023). Tandem cells for unbiased photoelectrochemical water splitting. Chem. Soc. Rev. 52, 4644–4671. doi:10.1039/D3CS00145H
Liu, B., Wang, T., Wang, S., Zhang, G., Zhong, D., Yuan, T., et al. (2022). Back-illuminated photoelectrochemical flow cell for efficient CO2 reduction. Nat. Commun. 13 (1), 7111. doi:10.1038/s41467-022-34926-x
Liu, D., Liu, J.-C., Cai, W., Ma, J., Yang, H. B., Xiao, H., et al. (2019). Selective photoelectrochemical oxidation of glycerol to high value-added dihydroxyacetone. Nat. Commun. 10 (1), 1779. doi:10.1038/s41467-019-09788-5
Liu, D., Wang, J., Bian, S., Liu, Q., Gao, Y., Wang, X., et al. (2020). Photoelectrochemical synthesis of ammonia with black phosphorus. Adv. Funct. Mat. 30 (24), 2002731. doi:10.1002/adfm.202002731
Luna, A. L., Dragoe, D., Wang, K., Beaunier, P., Kowalska, E., Ohtani, B., et al. (2017). Photocatalytic hydrogen evolution using Ni-Pd/TiO2: correlation of light absorption, charge-carrier dynamics, and quantum efficiency. J. Phys. Chem. C 121 (26), 14302–14311. doi:10.1021/acs.jpcc.7b01167
Minggu, L. J., Wan Daud, W. R., and Kassim, M. B. (2010). An overview of photocells and photoreactors for photoelectrochemical water splitting. Int. J. Hydrogen Energy 35 (11), 5233–5244. doi:10.1016/j.ijhydene.2010.02.133
Monteiro, M. C. O., Mirabal, A., Jacobse, L., Doblhoff-Dier, K., Barton, S. C., and Koper, M. T. M. (2021). Time-resolved local pH measurements during CO2 reduction using scanning electrochemical microscopy: buffering and tip effects. JACS Au 1 (11), 1915–1924. doi:10.1021/jacsau.1c00289
Nakamura, A., Ota, Y., Koike, K., Hidaka, Y., Nishioka, K., Sugiyama, M., et al. (2015). A 24.4% solar to hydrogen energy conversion efficiency by combining concentrator photovoltaic modules and electrochemical cells. Appl. Phys. Express 8 (10), 107101. doi:10.7567/APEX.8.107101
Peerakiatkhajohn, P., Yun, J.-H., Wang, S., and Wang, L. (2017). Review of recent progress in unassisted photoelectrochemical water splitting: from material modification to configuration design. J. Photonics Energy 7 (1), 012006. doi:10.1117/1.JPE.7.012006
Peter, L. M. (2016). “Photoelectrochemistry: from basic principles to photocatalysis,” in Photocatalysis: fundamentals and perspectives. Editors J. Schneider, J. Schneider, D. Bahnemann, J. Ye, G. Li Puma, and D. D. Dionysiou (The Royal Society of Chemistry), 1 –28.
Qureshi, F., and Tahir, M. (2024). Photoelectrochemical water splitting with engineering aspects for hydrogen production: recent advances, strategies and challenges. Int. J. Hydrogen Energy 69, 760–776. doi:10.1016/j.ijhydene.2024.05.039
Vanka, S., Zhou, B., Awni, R. A., Song, Z., Chowdhury, F. A., Liu, X., et al. (2020). InGaN/Si double-junction photocathode for unassisted solar water splitting. ACS Energy Lett. 5 (12), 3741–3751. doi:10.1021/acsenergylett.0c01583
Vilanova, A., Dias, P., Azevedo, J., Wullenkord, M., Spenke, C., Lopes, T., et al. (2020). Solar water splitting under natural concentrated sunlight using a 200 cm2 photoelectrochemical-photovoltaic device. J. Power Sources 454, 227890. doi:10.1016/j.jpowsour.2020.227890
Vilanova, A., Dias, P., Lopes, T., and Mendes, A. (2024). The route for commercial photoelectrochemical water splitting: a review of large-area devices and key upscaling challenges. Angew. Chem. Int. Ed. 53 (5), 2388–2434. doi:10.1039/D1CS01069G
Vilanova, A., Lopes, T., and Mendes, A. (2018). Large-area photoelectrochemical water splitting using a multi-photoelectrode approach. J. Power Sources 398, 224–232. doi:10.1016/j.jpowsour.2018.07.054
Wang, Q., Pornrungroj, C., Linley, S., and Reisner, E. (2022). Strategies to improve light utilization in solar fuel synthesis. Nat. Energy 7 (1), 13–24. doi:10.1038/s41560-021-00919-1
Wang, S., Liu, G., and Wang, L. (2019a). Crystal facet engineering of photoelectrodes for photoelectrochemical water splitting. Chem. Rev. 119 (8), 5192–5247. doi:10.1021/acs.chemrev.8b00584
Wang, W., Wang, H., Zhu, Q., Qin, W., Han, G., Shen, J. R., et al. (2016). Spatially separated photosystem II and a silicon photoelectrochemical cell for overall water splitting: a natural-artificial photosynthetic hybrid. Angew. Chem. Int. Ed. 128 (32), 9375–9379. doi:10.1002/ange.201604091
Wang, Y., Schwartz, J., Gim, J., Hovden, R., and Mi, Z. (2019c). Stable unassisted solar water splitting on semiconductor photocathodes protected by multifunctional GaN nanostructures. ACS Energy Lett. 4 (7), 1541–1548. doi:10.1021/acsenergylett.9b00549
Wang, Y., Wu, Y., Schwartz, J., Sung, S. H., Hovden, R., and Mi, Z. (2019b). A single-junction cathodic approach for stable unassisted solar water splitting. Joule 3 (10), 2444–2456. doi:10.1016/j.joule.2019.07.022
Wedege, K., Bae, D., Smith, W. A., Mendes, A., and Bentien, A. (2018). Solar redox flow batteries with organic redox couples in aqueous electrolytes: a minireview. J. Phys. Chem. C 122 (45), 25729–25740. doi:10.1021/acs.jpcc.8b04914
Yamaguchi, S., Watanabe, K., Minegishi, T., and Sugiyama, M. (2023). Solar to hydrogen efficiency of 28.2% under natural sunlight achieved by a combination of five-junction concentrator photovoltaic modules and electrolysis cells. Sustain. Energy. Fuels 7 (6), 1377–1381. doi:10.1039/D2SE01754G
Yang, J., Wang, D., Han, H., and Li, C. (2013). Roles of cocatalysts in photocatalysis and photoelectrocatalysis. Acc. Chem. Res. 46 (8), 1900–1909. doi:10.1021/ar300227e
Yang, W., and Moon, J. (2019). Recent advances in earth-abundant photocathodes for photoelectrochemical water splitting. ChemSusChem 12 (9), 1889–1899. doi:10.1002/cssc.201801554
Yao, T., An, X., Han, H., Chen, J. Q., and Li, C. (2018). Photoelectrocatalytic materials for solar water splitting. Adv. Energy Mat. 8 (21), 1800210. doi:10.1002/aenm.201800210
Yi, S.-S., Zhang, X.-B., Wulan, B.-R., Yan, J.-M., and Jiang, Q. (2018). Non-noble metals applied to solar water splitting. Energy Environ. Sci. 11 (11), 3128–3156. doi:10.1039/C8EE02096E
Zhang, F., Li, Y.-H., Qi, M.-Y., Yamada, Y. M., Anpo, M., Tang, Z.-R., et al. (2021). Photothermal catalytic CO2 reduction over nanomaterials. Chem. Catal. 1 (2), 272–297. doi:10.1016/j.checat.2021.01.003
Zhao, J., Guo, X., Shi, R., Waterhouse, G. I., Zhang, X., Dai, Q., et al. (2022). NiFe nanoalloys derived from layered double hydroxides for photothermal synergistic reforming of CH4 with CO2. Adv. Funct. Mat. 32 (31), 2204056. doi:10.1002/adfm.202204056
Zhou, P., Navid, I. A., Ma, Y., Xiao, Y., Wang, P., Ye, Z., et al. (2023). Solar-to-hydrogen efficiency of more than 9% in photocatalytic water splitting. Nature 613 (7942), 66–70. doi:10.1038/s41586-022-05399-1
Zhu, X., Huang, J., and Eikerling, M. (2024). Hierarchical modeling of the local reaction environment in electrocatalysis. Acc. Chem. Res. 57, 2080–2092. doi:10.1021/acs.accounts.4c00234
Ziani, A., Al-Shankiti, I., Khan, M. A., and Idriss, H. (2020). Integrated photo-electrocatalytic (PEC) systems for water splitting to hydrogen and oxygen under concentrated sunlight: effect of internal parameters on performance. Energy and Fuels 34 (10), 13179–13185. doi:10.1021/acs.energyfuels.0c02481
Keywords: photoelectrochemistry, water splitting, hydrogen energy, concentrated sunlight, protocols
Citation: Li K, Dong WJ and Mi Z (2025) Photoelectrochemical water splitting under concentrated sunlight: best practices and protocols. Front. Energy Res. 13:1550153. doi: 10.3389/fenrg.2025.1550153
Received: 31 December 2024; Accepted: 10 March 2025;
Published: 25 March 2025.
Edited by:
Sudhakar Kumarasamy, Universiti Malaysia Pahang, MalaysiaReviewed by:
Dowon Bae, Loughborough University, United KingdomCopyright © 2025 Li, Dong and Mi. This is an open-access article distributed under the terms of the Creative Commons Attribution License (CC BY). The use, distribution or reproduction in other forums is permitted, provided the original author(s) and the copyright owner(s) are credited and that the original publication in this journal is cited, in accordance with accepted academic practice. No use, distribution or reproduction is permitted which does not comply with these terms.
*Correspondence: Zetian Mi, enRtaUB1bWljaC5lZHU=
†These authors have contributed equally to this work
Disclaimer: All claims expressed in this article are solely those of the authors and do not necessarily represent those of their affiliated organizations, or those of the publisher, the editors and the reviewers. Any product that may be evaluated in this article or claim that may be made by its manufacturer is not guaranteed or endorsed by the publisher.
Research integrity at Frontiers
Learn more about the work of our research integrity team to safeguard the quality of each article we publish.