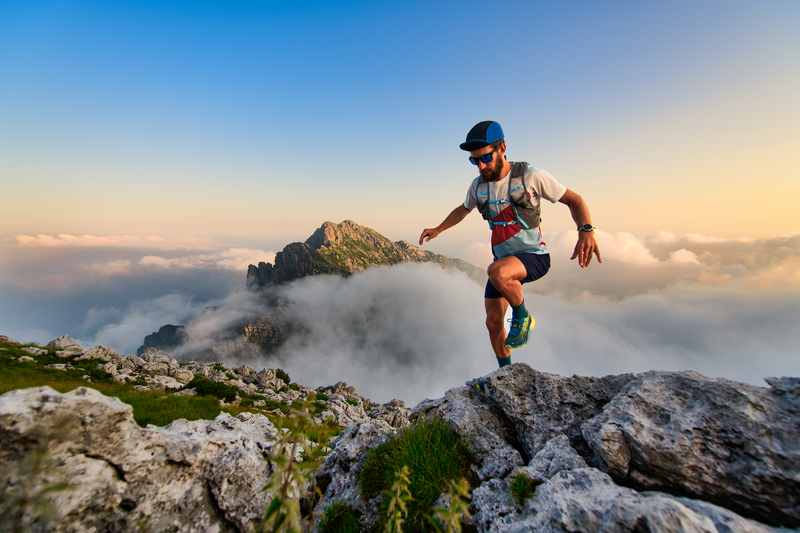
94% of researchers rate our articles as excellent or good
Learn more about the work of our research integrity team to safeguard the quality of each article we publish.
Find out more
MINI REVIEW article
Front. Energy Res. , 07 February 2025
Sec. Hydrogen Storage and Production
Volume 13 - 2025 | https://doi.org/10.3389/fenrg.2025.1515463
Hydrogen (H2) will play a vital role in the global shift towards sustainable energy systems. Due to the high cost and challenges associated with storing hydrogen in large quantities for industrial applications, Underground Hydrogen Storage (UHS) in geological formations has emerged as a promising solution. Clay minerals, abundant in subsurface environments, play a critical role in UHS by providing low permeability, cation exchange capacity, and stability, essential for preventing hydrogen leakage. However, microorganisms in the subsurface, particularly hydrogenotrophic species, interact with clay minerals in ways that can affect the integrity of these storage systems. Microbes form biofilms on clay surfaces, which can cause pore clogging and reduce the permeability of the reservoir, potentially stabilizing H2 storage and limiting injectivity. Microbial-induced chemical weathering, through the production of organic acids and redox reactions, can degrade clay minerals, releasing metal ions and destabilizing the storage site. These interactions raise concerns about the long-term storage capacity of UHS, as microbial processes could lead to H2 loss and caprock degradation, compromising the storage system’s effectiveness. This mini review aims to cover the current understanding of the interactions between clay minerals and microorganisms and how these dynamics can affect the safe and sustainable deployment of UHS.
In the evolving narrative of global energy transitions, hydrogen (H2) has emerged as a viable option in pursuing a sustainable and low-carbon future. Its growing use in several industries from transportation to large-scale energy storage systems intended to balance the supply and demand for renewable energy (such as wind and solar power), is largely due to its clean energy potential (Čábelková et al., 2020). Despite its advantages, H2 poses unique storage issues. Hydrogen, the smallest and lightest molecule, is intrinsically difficult to hold. It requires substantial volumes (1 kg of gas has a volume of 11 m3 at ambient temperature and pressure) to be kept efficiently, and due to its low density (0.082 kg/m3 at 25°C and 1 atm), typical storage options like high-pressure cylinders or cryogenic tanks can be costly and complex (Züttel, 2003; Tarkowski et al., 2021; Zivar et al., 2021; Capurso et al., 2022; Krevor et al., 2023). Due to this, Underground Hydrogen Storage (UHS) has been proposed as a robust storage infrastructure for the short- and long-term storage of H2 in subsurface geologic formations (porous media).
UHS encompasses several geological and engineered options, such as salt caverns, depleted oil and gas fields (DOGFs), and aquifers that all offer sufficient storage space for large amounts of H2 (Zivar et al., 2021). Due to the limited geographic availability and the costly creation and maintenance of salt caverns, DOGFs, and aquifers offer a more suitable option for large-scale long-term storage of H2 (Carden and Paterson, 1979; Tarkowski, 2019; Lankof and Tarkowski, 2020; Zivar et al., 2021). Although each storage reservoir offers different geological characteristics, it is a common necessity for an impermeable seal (caprock) and tightness of the pore network to prevent the leakage of stored H2 (Reitenbach et al., 2015; Wolff-Boenisch et al., 2023).
Minerals are considered the building blocks of Earth, as they are vital for forming the Earth’s rocks and driving geological processes. Clays are comprised of tetrahedral and octahedral sheets, most commonly in a 1:1 or 2:1 ratio. Kaolinite is an example of a 1:1 clay structure that consists of one tetrahedral sheet and one octahedral sheet. Smectite is an example of a 2:1 clay structure with two tetrahedral sheets and an octahedral sheet in between. Clay minerals, prevalent in potential geological storage sites (except salt caverns), possess both physical and chemical properties, such as small particle size (<2 μm), large surface area, unique crystal structures, cation exchange capabilities, plastic behavior when wet, catalytic abilities, swelling behavior, and low permeability that promote the impermeability tightness that is needed for the long-term storage of H2 (Aboudi Mana et al., 2017; Al-Yaseri et al., 2021; Wolff-Boenisch et al., 2023). In literature, it is reported that clay minerals, such as illite, chlorite, and kaolinite, can result in the trapping of H2, which is key for UHS in porous media (Truche et al., 2018; Zivar et al., 2021). Ghasemi et al., determined through Molecular Dynamic simulations, that diffusion of H2 significantly increases based on clay critical pore size, playing an important role in the caprock leakage potential (Ghasemi et al., 2022). In previous studies, the chemical influence of H2 on clay minerals proved to be almost negligible (Yekta et al., 2018; Bo et al., 2021). However, along with promoting the storage of H2 these specific characteristics of clay minerals, also foster the appropriate habitat needed for a variety of microbial communities to flourish in the subsurface. When injecting H2 into the subsurface, understanding the relationship between clay minerals and microorganisms natural to the system is crucial, as particular microorganism enzymes can catalyze abiotic reactions.
Within the earth’s subsurface, there is a large and varied microbial ecosystem composed of archaea and bacteria. These microorganisms require several fundamental materials for existence, including water, an energy source, and several important elements (C, N, Na, and other trace elements) to thrive (Dopffel et al., 2021). Energy can be obtained by microorganisms through the simultaneous reduction of an electron acceptor and oxidation of an electron donor. Due to its low reduction potential, H2 plays a key role in the subsurface as the primary electron donor needed to create cellular energy for microbial metabolism (Gregory et al., 2019; Dopffel et al., 2021). During the injection of H2 into a subsurface reservoir, microbial growth is stimulated, as a result of elevated H2 concentrations (Gao et al., 2024). Hydrogen participates in various microbially catalyzed redox reactions that are important to UHS, such as methanogenesis, acetogenesis, sulfate reduction, and iron reduction (Truche et al., 2013; Berta et al., 2018; Dopffel et al., 2021; Liu et al., 2023; Kumari and Ranjith, 2023). All these hydrogenotrophic reactions result in the consumption of H2 and subsequent energy loss, which is not ideal for UHS. Microbially induced gas mixture changes, souring and H2S formation, pore plugging/clogging, and dissolution of minerals can result in leakage of stored H2 negatively impacting UHS (Dopffel et al., 2021).
Previously, microbial H2 consumption has been reported in underground gas storage (UGS) field tests, such as the underground reservoir of town gas, located in Lobodice, Czechoslovakia (Amigáň et al., 1990). It was reported that about half of stored H2 was microbially converted to methane in interaction with CO and CO2 to CH4 (Amigáň et al., 1990; Buzek et al., 1994). Along with field tests, several laboratory experiments have been carried out to examine microbial activity’s effects on UHS. For example Liu et al. discovered a considerable loss of H2 after 2 days from microbial consumption and a change in surface wettability through biochemical pore network experiments using sulfate-reducing bacteria (Liu et al., 2023). However, there has been a lack of studies considering the interaction of clay minerals and microorganisms in UHS. This mini-review is aimed at covering the current views on the relationship between clay minerals and microorganisms by understanding how clay minerals affect microorganisms and how microorganisms affect clay minerals. Furthermore, this review aims to describe the microbial-clay-H2 risks helping better implement UHS in the future.
It is first necessary to understand the question: “How do clay minerals impact microorganisms?” Microorganisms are commonly found to habitat different mineral surfaces depending on the physical and chemical characteristics of the mineral (Uroz et al., 2009; Dong et al., 2022). Because of clay minerals’ small particle size, large surface area, and water retention properties, these properties allow for the formation of organo-mineral aggregates, that are ideal for microorganism development (Cuadros, 2017). Clay minerals ease the development of microorganisms by providing habitat, supplying nutrients, and adding protection from external elements. However, clay minerals can negatively impact microbial development by reducing respiration (Lavie and Stotzky, 1986; McMahon et al., 2016). The impacts of clay minerals on microorganisms can have implications for UHS, including H2 consumption, the transformation to unwanted byproducts (such as CH4 and H2S), and the weakening of reservoir and caprock leading to H2 leakage.
Microorganisms can inhabit almost all types of mineral surfaces depending on the physical and chemical characteristics of the mineral. Clay mineral surfaces provide a supportive habitat for microorganisms in the subsurface, due to their specific physical, chemical, and electrostatic properties. The fine-grained nature of clay (<2 μm) results in a large surface area to volume ratio, offering many microhabitats for microorganisms to adhere to and thrive (Fomina and Skorochod, 2020). Microhabitats are crucial for microorganisms, allowing them to adsorb water, nutrients, and organic molecules onto their surface through adsorption, due to their large surface area and adsorption capacity (Zhang et al., 2021). The swelling capacity of clays allows for water to collect in the pores and interlayer space of clay minerals, helping maintain moisture in dry environments, which is important for microbial survival (Cuadros, 2017). Metals nutrients leached from clay minerals, such as nitrogen, potassium, and sodium; and organic compounds can accumulate on clay surfaces, creating a nutrient-rich zone that acts as a food source for microorganisms, supplementing their metabolism and growth (Merchant and Helmann, 2012; Dong et al., 2022). Clay minerals present in UHS can provide the necessary habitat (surface area, water, and food supply) to support microorganism metabolism, increasing the risk of stored H2 consumption during microbial metabolism and future loss of energy. Microbes such as fermenters within UHS can produce H2 through the fermentation of organic molecules, potentially countering the consumption of H2 from methanogens, homoacteogens, and sulfate-reducers (Haddad et al., 2022a). It was found that H2 produced during fermentation was consumed by sulfate-reducers, suggesting that fermenter effects in UHS would be negligible (Haddad et al., 2022b). However, with an increased partial pressure of H2 (PH2) from injection into UHS, fermenter activity could be suppressed due to the created thermodynamic barrier, resulting in less favorable conditions for fermentative bacteria. The suppression of fermenters could lead to slower decomposition of complex organic matter into volatile fatty acids, alcohols, and gases, resulting in the accumulation of organic carbon, disrupting the natural turnover of carbon in the environment (Conrad, 1999). The resulting reduced availability of bioavailable substrates, such as acetate and H2 can disrupt anaerobic microbial processes (e.g., methanogenesis) further affecting the cycling of carbon and other elements (e.g., nitrogen), that can affect the microbial ecosystem (Conrad, 1999).
The structural diversity of clay minerals (layer types) leads to different physical and chemical properties. Variations in these properties create heterogeneous habitats promoting microbial community diversity. Depending on the clay mineral structures and chemical composition, there can be variations in available nutrients and resistance to leaching (Dong, 2012). First layer type (1:1) clay minerals, such as kaolinite, display a lower cation exchange capacity (<10 meq/100 g) compared to second layer type (2:1) clay minerals, such as smectite (80–150 meq/100 g), making it more difficult to obtain and release necessary nutrients (Grim, 1968; Schulze, 2002; Huang et al., 2011; Fomina and Skorochod, 2020). The lower capacity for nutrient storage in kaolinite, limits the retention of nutrients, such as potassium, calcium, and magnesium, decreasing the microbial diversity potential (Schulze, 2002; Huang et al., 2011). Comparatively, montmorillonite has a high cation exchange capacity, high absorbance potential for polymers, and organic biomolecules (Burns, 1980; Napper, 1983; Lagaly, 1993; Lünsdorf et al., 2000; Detellier, 2018). The significant space between the layers of montmorillonite allows for the cations to more easily be exchanged, providing higher nutrient retention for microbes compared to kaolinite (Figure 1) (Lagaly, 1993; Detellier, 2018).
Figure 1. Comparison of kaolinite (1:1) and montmorillonite’s (2:1) interlayer space. With more interlayer space in montmorillonite, there is ample space for cation exchange allowing for increased nutrient and water retention. This creates an ideal environment for microbes to form biofilms (Wang et al., 2022).
The greater initial cation exchange capacity contributes to the negative charge on montmorillonite’s surface, attracting the positively charged components around the perimeter of polymer molecules, allowing for better absorption of water molecules between its layers (Figure 1) (Lagaly, 1993; Theng, 2012; Huang et al., 2022). The increased nutrient, water, and organic biomolecule retention creates a moist stable environment that is ideal for biofilm formation and microbial colonization (Figure 1). Depending on the clay mineral, different aggressiveness and techniques are needed to successfully leach the minerals for necessary nutrients (Cuadros, 2017). For instance Cuadros (2017), reported that the nutritional content of smectite is far higher than that of kaolinite, requiring microorganisms to exhibit more activity to dissolve the same amount of nutrients in a kaolinite-dominated environment compared to a smectite-dominated environment. In UHS, to limit microbial activity, kaolinite would be an ideal dominant clay mineral, due to low nutrient adsorption and exchange. Clay minerals, such as smectite would be less desirable, as these clays better retain the nutrients needed for microorganism metabolism, leading to increased H2 consumption.
Clay minerals play a crucial protective role for microbes in the subsurface by creating stable and secure microenvironments that shield them from various environmental stressors. The fine-grained structure and high surface area of clay minerals provide numerous microhabitats where microorganisms can physically reside, protected from ultraviolet radiation (UVR), physical erosion, and temperature fluctuations (Fomina and Skorochod, 2020; Dong et al., 2022). In subsurface environments, clay-rich zones have low permeability, limiting the depth to which UV radiation can penetrate, ensuring microorganisms in these microhabitats remain shielded from the surface UV effects (Scappini et al., 2004; Fomina and Skorochod, 2020). The chemical composition of clay minerals may also provide varying degrees of protection from UVR (Kugler and Dong, 2019). Kugler and Dong (2019), found that phyllosilicates rich in structural Fe, better shielded cyanobacteria from UVR. Microorganisms in clay-rich environments can secrete extracellular polymeric substances (EPS), which form biofilms of ‘clay-hutches’ that further anchor them to the large surface of clays (Lünsdorf et al., 2000). These biofilms enhance the protective effects of clay by creating a sticky, resilient matrix that can dissipate external mechanical disturbances and help the microbial community remain in place (Cuadros, 2017; Fomina and Skorochod, 2020). Clay minerals have low thermal conductivity, meaning they can act as insulating barriers that moderate temperature shifts in their surrounding environments (Dong et al., 2009; Fomina and Skorochod, 2020). The compact nature of clay-rich sediments also reduces heat transfer, which slows down the rate at which temperatures rise or fall in the pore spaces inhabited by microorganisms (Dong et al., 2009). Clay-rich UHS reservoirs would provide adequate protection to promote the survival of microorganisms, further enabling microbial processes that could result in the microbial depletion of stored hydrogen and the creation of harmful byproducts, leading to more H2 loss. Overall, clay-rich UHS could promote adequate habitats for microorganisms, providing necessary nutrients, water, and protection. These habitat properties of clay minerals could be detrimental to the long-term and short-term storage of H2.
It is also necessary to understand how the microorganisms impact clay minerals as that can also impact UHS Microorganisms can form biofilms on clay mineral grain surfaces, allowing for the surface, protection, moisture, and controlled environment needed for development (Cuadros, 2017). Clay minerals, in return, can be both transformed and formed by microorganisms through processes, such as weathering, dissolution, and biomineralization (Li et al., 2019). These processes can result in changes in the composition of clay, properties (such as plasticity), and the pore network, all impacting the viability of potential UHS. The impacts of microorganisms on clay minerals can result in biofilm pore clogging, corrosion of UHS leading to H2 leakage, and H2 consumption (Liu et al., 2023).
During the injection of H2 into the subsurface, elevated concentrations of H2 may encourage the growth of hydrogenotrophic microorganisms. This can have a negative impact on H2 storage, such as biofouling from biofilms (Dopffel et al., 2021; Liu et al., 2023; Thaysen et al., 2023). The extensive surface area of clay minerals, montmorillonite, kaolinite, and illite, provides ample space for microbial attachment, enhancing biofilm formation. The growing layers of microbial cells and EPS during biofilm formation can physically obstruct the pore space of clay minerals, leading to reduced pore diameter (Eddaoui et al., 2021; Liu et al., 2023). Over time, as the biofilm expands, the small pores of clay minerals are effectively sealed off, limiting the permeability of UHS (Eddaoui et al., 2021). This reduced permeability can restrict the movement of essential nutrients leading to a heterogenous nutrient distribution (Shaw et al., 1985; Pintelon et al., 2012). This would limit the growth and survival of nutrient demanding microbes in depleted regions. The localized resource limitation would create increased competition between microbial species, reducing the microbial diversity in UHS (Zhong and Wu, 2013). Similarly, water transport would also be restricted adding to desiccation stress experienced in these depleted areas (Zhong and Wu, 2013). Biofilm pore clogging could be beneficial for UHS, by limiting the transport of needed nutrients and water, reducing microbial growth and associated H2 consuming metabolic processes. Conversely, biofilm pore clogging can create beneficial microhabitats where the restricted flow dynamics result in an accumulation of organic matter and nutrients near the biofilms supporting microbial growth in UHS (Pintelon et al., 2012). This could lead to increased consumption of stored H2. Restricted diffusion of oxygen could promote the growth of anaerobes, in oxygen-depleted areas, facilitating processes such as methanogenesis and sulfate reduction (de Beer et al., 1994). In confined pore spaces, the swelling and expansion of biofilms can also induce physical weathering on mineral structures (Eddaoui et al., 2021). Over time, mechanical stress can become large enough to cause microfractures (localized weakening), which can be detrimental to the trapping of stored H2 in UHS. The microbial weathering of clay minerals is significant in subsurface environments, such as UHS, where clay stability is crucial for the storage integrity of easily diffused H2.
Along with physical implications for UHS, biofilm formation on the surface of clay minerals can also chemical implications. As microbes grow on the surface of clays minerals, through EPS, the surface wettability can be altered (Soccol et al., 2005; Liu et al., 2024). By decreasing the contact angle between liquid and the clay surface, the surface can become more hydrophobic or hydrophilic depending on the surface properties, microbial strains, and initial conditions (Kowalewski et al., 2006; Liu et al., 2024). Depending on the microbial strain, a more hydrophilic or hydrophobic surface can discourage the attachment of microbes, which would be ideal for UHS (Lee et al., 1998; Hannig, 1999; Liu et al., 2024). Reservoir souring can also be a result of biofilm formation of clay mineral surfaces. Most commonly, SRB present in biofilms can amplify corrosion process through the concentration of corrosive compounds, such as H2S, leading to a drop of reservoir fluid pH and corrosion of UHS infrastructure and potential leakage of stored H2 (Eckert, 2015). However, biofilms can possibly perform a protective role against corrosion in UHS, as they can hinder the interaction of corrosive agents, such as oxygen, protecting infrastructure from corrosion in UHS (Eckert, 2015; Liu et al., 2024).
The lattice of naturally occurring clay minerals frequently contains Fe(II) and Fe(III) [predominately Fe(III)], with the majority of the Fe dispersed in the octahedral and tetrahedral layers (Zhou et al., 2016; Fan et al., 2023). However, Fe can also be found existing in an ion exchangeable form at basal planes and on the edge of clay surfaces bound by hydroxyl groups (Hofstetter et al., 2003; Neumann et al., 2009; Fan et al., 2023). It is well known that Fe(III) within clay minerals can be microbially reduced by iron-reducing bacteria (IRB), sulfate-reducing bacteria (SRB), and methanogens (Kostka et al., 1999; Liu et al., 2012; Zhang et al., 2013; Fan et al., 2023). In UHS systems, IRB can use H2 as an energy source and reduce Fe(III) to Fe(II) (Figure 2) (Liu et al., 2012; Zhang et al., 2013).
Figure 2. Overview of iron reduction process mediated by IRB on clay mineral surfaces in UHS. Iron reduction results in the weakening of the clay mineral structure leading to potential leakage of H2 (Fan et al., 2023).
When bacteria interacts with clay’s structural Fe(III), it experiences small, reversible changes in structure and chemistry. However, reductive dissolution has also been observed (Stucki, 2011), where the mineral breaks down when Fe(III) is reduced to Fe(II). The relative importance of these two mechanisms depends on factors like the type of clay mineral, the degree of reduction, and the surrounding water and microbial environment. Previous studies suggest that when less than a third of the total Fe(III) is reduced, the clay mineral remains stable (Stucki, 2011). But when this threshold is exceeded, the mineral becomes unstable and may dissolve, forming new minerals like amorphous silica, siderite, and illite (Stucki, 2011). The reduction process also affects the clay mineral’s properties, such as decreasing its surface area, water absorption, and hydraulic conductivity. At the same time, the reduction increases the mineral’s negative layer charge and exchange capacity for cations, although these cations become harder to exchange.
The resulting Fe(II) is predominately edge-complexed Fe(II) compared to structural Fe(II), as it is harder for microbes to “attack” the interior structure of clay minerals (Fan et al., 2023). The reduction of Fe(III) to Fe(II) could potentially collapse the structure of phyllosilicate clay minerals (Figure 2) (Kostka et al., 1999; Boylan et al., 2019; Rooney et al., 2024). The weakening of clay minerals from IRB could compromise the structural integrity of UHS, leading to stored H2 leakage.
Unlike abiotic dissolution, which is largely driven by pH, biotic dissolution also relies on various organic compounds to disrupt the mineral structure in the absence of significant microbial activity. Structural Fe(III) in clay minerals functions as an electron acceptor, while organic matter serves as an electron (Dong, 2012). Key organic compounds involved in this process include siderophores, which help break down iron oxides and silicate minerals, as well as organic acids, facilitate iron transport, and EPS, secreted by microorganisms that alter the surrounding environment (Ahmed and Holmström, 2014; Torres et al., 2014; Finlay et al., 2020; Ribeiro et al., 2020).
Under optimal conditions, only a portion of structural Fe(III) in clay can be reduced biologically, likely because biogenic Fe(II) is released during the process and then accumulates on the surface of clay and microorganisms. This accumulated Fe(II) can block electron transfer and hinder further reduction (Jaisi et al., 2007; Shi et al., 2016). However, this inhibitory effect can be mitigated with flowing water to remove Fe(II) from the clay surfaces. Additionally, incomplete reduction of Fe(III) may be due to the build-up of solid products (such as amorphous phases) on the clay and cell surfaces, or due to changes in the system’s energy requirements (Dong et al., 2009; Dong, 2012).
This mini review of the current understanding of clay mineral-microorganism interactions provides a basis for the discussions on the current conditions and future directions of this key area of UHS research. Understanding the impact of interactions with clay minerals and microorganisms in UHS is imperative for safe and sustainable future development. Currently, there is a lack of experimental studies highlighting clay mineral-microorganism interactions in the presence of H2. In the future, various experimental studies should be conducted with varying variables to enhance the current literature further. Conducting experiments with different pure clay minerals (kaolinite, illite, and montmorillonite) with mixed natural microbial communities (not cultured), all subject to a known amount of varying concentrations of H2 gas and blends at UHS reservoir temperature and pressure. Investigating different clay minerals with a natural microbial community could provide insight into what clay minerals are preferably attacked by microorganisms, how each clay mineral responds to microorganism attacks and the rate at which clay minerals can be manipulated by microorganisms. This would lead to a better understanding of what clay minerals are most suitable for the effective underground storage of H2 when wanting to reduce microbial H2 consumption, H2 migration from microbial-induced clay structure weakening, and preventing H2 gas injectivity reduction. Unlike previous studies in literature, using natural communities of microorganisms (not lab-grown) will better represent microorganism metabolisms in potential subsurface reservoirs. The potential future studies outlined above can help ensure that H2 can be safely and efficiently stored underground for the future implementation of H2 as a sustainable energy source.
1) In UHS, clay minerals would provide the appropriate living conditions for the promotion of microorganism biodiversity and growth, including needed surface area, small pore space, nutrients (metal cations and organic compounds), water, and protection leading to microbial consumption of stored H2.
2) Microbial-induced biofilms can clog the pore space of clay minerals limiting nutrient, water, and oxygen movement reducing microbial H2 consumption.
3) Microorganisms can weaken the silicate structure of clay minerals from reduction of Fe and swelling and contraction of biofilms, resulting in microbial H2 consumption, metal release, and H2 leakage.
4) Future studies are needed to fill the gap of the lack of experimental clay mineral-microorganism-H2 studies in the literature.
AC: Conceptualization, Writing–original draft, Writing–review and editing. SS: Conceptualization, Funding acquisition, Project administration, Resources, Supervision, Writing–review and editing.
The author(s) declare that financial support was received for the research, authorship, and/or publication of this article. This research was funded by the Department of Energy Office of Basic Energy Sciences, grant number DE-SC0023489 to SS.
The authors declare that the research was conducted in the absence of any commercial or financial relationships that could be construed as a potential conflict of interest.
The author(s) declare that no Generative AI was used in the creation of this manuscript.
All claims expressed in this article are solely those of the authors and do not necessarily represent those of their affiliated organizations, or those of the publisher, the editors and the reviewers. Any product that may be evaluated in this article, or claim that may be made by its manufacturer, is not guaranteed or endorsed by the publisher.
Aboudi Mana, S. C., Hanafiah, M. M., and Chowdhury, A. J. K. (2017). Environmental characteristics of clay and clay-based minerals. Geol. Ecol. Landscapes 1 (3), 155–161. doi:10.1080/24749508.2017.1361128
Ahmed, E., and Holmström, S. J. M. (2014). Siderophores in environmental research: roles and applications. Microb. Biotechnol. 7 (3), 196–208. doi:10.1111/1751-7915.12117
Al-Yaseri, A., Wolff-Boenisch, D., Fauziah, C. A., and Iglauer, S. (2021). Hydrogen wettability of clays: implications for underground hydrogen storage. Int. J. Hydrogen Energy 46 (69), 34356–34361. doi:10.1016/j.ijhydene.2021.07.226
Amigáň, P., Greksák, M., Kozánková, J., Buzek, F., Onderka, V., and Wolf, I. (1990). Methanogenic bacteria as a key factor involved in changes of town gas stored in an underground reservoir. FEMS Microbiol. Ecol. 6 (3), 221–224. doi:10.1111/j.1574-6968.1990.tb03944.x
Berta, M., Dethlefsen, F., Ebert, M., Schäfer, D., and Dahmke, A. (2018). Geochemical effects of millimolar hydrogen concentrations in groundwater: an experimental study in the context of subsurface hydrogen storage. Environ. Sci. and Technol. 52 (8), 4937–4949. doi:10.1021/acs.est.7b05467
Bo, Z., Zeng, L., Chen, Y., and Xie, Q. (2021). Geochemical reactions-induced hydrogen loss during underground hydrogen storage in sandstone reservoirs. Int. J. Hydrogen Energy 46 (38), 19998–20009. doi:10.1016/j.ijhydene.2021.03.116
Boylan, A. A., Perez-Mon, C., Guillard, L., Burzan, N., Loreggian, L., Maisch, M., et al. (2019). H2-fuelled microbial metabolism in Opalinus Clay. Appl. Clay Sci. 174, 69–76. doi:10.1016/j.clay.2019.03.020
Buzek, F., Onderka, V., Vancura, P., and Wolf, I. (1994). Carbon isotope study of methane production in a town gas storage reservoir. Fuel 73 (5), 747–752. doi:10.1016/0016-2361(94)90019-1
Čábelková, I., Strielkowski, W., Firsova, I., and Korovushkina, M. (2020). Public acceptance of renewable energy sources: a case study from the Czech republic. Energies 13 (7), 1742. doi:10.3390/en13071742
Capurso, T., Stefanizzi, M., Torresi, M., and Camporeale, S. (2022). Perspective of the role of hydrogen in the 21st century energy transition. Energy Convers. Manag. 251, 114898. doi:10.1016/j.enconman.2021.114898
Carden, P. O., and Paterson, L. (1979). Physical, chemical and energy aspects of underground hydrogen storage. Int. J. Hydrogen Energy 4 (6), 559–569. doi:10.1016/0360-3199(79)90083-1
Conrad, R. (1999). Contribution of hydrogen to methane production and control of hydrogen concentrations in methanogenic soils and sediments. FEMS Microbiol. Ecol. 28 (3), 193–202. doi:10.1111/j.1574-6941.1999.tb00575.x
Cuadros, J. (2017). Clay minerals interaction with microorganisms: a review. Clay Miner. 52 (2), 235–261. doi:10.1180/claymin.2017.052.2.05
de Beer, D., Stoodley, P., Roe, F., and Lewandowski, Z. (1994). Effects of biofilm structures on oxygen distribution and mass transport. Biotechnol. Bioeng. 43 (11), 1131–1138. doi:10.1002/bit.260431118
Dong, H. (2012). Clay–Microbe interactions and implications for environmental mitigation. Elements 8 (2), 113–118. doi:10.2113/gselements.8.2.113
Dong, H., Huang, L., Zhao, L., Zeng, Q., Liu, X., Sheng, Y., et al. (2022). A critical review of mineral–microbe interaction and co-evolution: mechanisms and applications. Natl. Sci. Rev. 9 (10), nwac128. doi:10.1093/nsr/nwac128
Dong, H., Jaisi, D. P., Kim, J., and Zhang, G. (2009). Microbe-clay mineral interactions. Am. Mineralogist 94 (11–12), 1505–1519. doi:10.2138/am.2009.3246
Dopffel, N., Jansen, S., and Gerritse, J. (2021). Microbial side effects of underground hydrogen storage – knowledge gaps, risks and opportunities for successful implementation. Int. J. Hydrogen Energy 46 (12), 8594–8606.doi:10.1016/j.ijhydene.2020.12.058
Eckert, R. B. (2015). Emphasis on biofilms can improve mitigation of microbiologically influenced corrosion in oil and gas industry. Corros. Eng. Sci. Technol. 50 (3), 163–168. doi:10.1179/1743278214Y.0000000248
Eddaoui, N., Panfilov, M., Ganzer, L., and Hagemann, B. (2021). Impact of pore clogging by bacteria on underground hydrogen storage. Transp. Porous Media 139 (1), 89–108. doi:10.1007/s11242-021-01647-6
Fan, Q., Wang, L., Fu, Y., Li, Q., Liu, Y., Wang, Z., et al. (2023). Iron redox cycling in layered clay minerals and its impact on contaminant dynamics: a review. Sci. Total Environ. 855, 159003. doi:10.1016/j.scitotenv.2022.159003
Finlay, R. D., Mahmood, S., Rosenstock, N., Bolou-Bi, E. B., Köhler, S. J., Fahad, Z., et al. (2020). Reviews and syntheses: biological weathering and its consequences at different spatial levels – from nanoscale to global scale. Biogeosciences 17 (6), 1507–1533. doi:10.5194/bg-17-1507-2020
Fomina, M., and Skorochod, I. (2020). Microbial interaction with clay minerals and its environmental and biotechnological implications. Minerals 10 (10), 861. doi:10.3390/min10100861
Gao, Q., Liu, J., and Elsworth, D. (2024). Phenomenal study of microbial impact on hydrogen storage in aquifers: a coupled multiphysics modelling. Int. J. Hydrogen Energy 79, 883–900. doi:10.1016/j.ijhydene.2024.07.004
Ghasemi, M., Omrani, S., Mahmoodpour, S., and Zhou, T. (2022). Molecular dynamics simulation of hydrogen diffusion in water-saturated clay minerals; implications for Underground Hydrogen Storage (UHS). Int. J. Hydrogen Energy 47 (59), 24871–24885. doi:10.1016/j.ijhydene.2022.05.246
Gregory, S. P., Barnett, M. J., Field, L. P., and Milodowski, A. E. (2019). Subsurface microbial hydrogen cycling: natural occurrence and implications for industry. Microorganisms 7 (2), 53. doi:10.3390/microorganisms7020053
Haddad, P. G., Mura, J., Castéran, F., Guignard, M., Ranchou-Peyruse, M., Sénéchal, P., et al. (2022a). Biological, geological and chemical effects of oxygen injection in underground gas storage aquifers in the setting of biomethane deployment. Sci. Total Environ. 806, 150690. doi:10.1016/j.scitotenv.2021.150690
Haddad, P. G., Ranchou-Peyruse, M., Guignard, M., Mura, J., Casteran, F., Ronjon-Magand, L., et al. (2022b). Geological storage of hydrogen in deep aquifers – an experimental multidisciplinary study. Energy and Environ. Sci. 15 (8), 3400–3415. doi:10.1039/D2EE00765G
Hannig, M. (1999). Transmission electron microscopy of early plaque formation on dental materials in vivo. Eur. J. Oral Sci. 107 (1), 55–64. doi:10.1046/j.0909-8836.1999.eos107109.x
Hofstetter, T. B., Schwarzenbach, R. P., and Haderlein, S. B. (2003). Reactivity of Fe(II) species associated with clay minerals. Environ. Sci. and Technol. 37 (3), 519–528. doi:10.1021/es025955r
Huang, P. M., Li, Y., and Sumner, M. E. (2011). Handbook of soil Sciences: properties and processes. Second Edition. Boca Raton, FL: CRC Press.
Huang, W., Lai, H., Du, J., Zhou, C., Liu, Z., and Ni, Q. (2022). Effect of polymer water retaining agent on physical properties of silty clay. Chem. Biol. Technol. Agric. 9 (1), 47. doi:10.1186/s40538-022-00309-z
Jaisi, D. P., Dong, H., and Liu, C. (2007). Influence of biogenic Fe(II) on the extent of microbial reduction of Fe(III) in clay minerals nontronite, illite, and chlorite. Geochimica Cosmochimica Acta 71 (5), 1145–1158. doi:10.1016/j.gca.2006.11.027
Kostka, J. E., Wu, J., Nealson, K. H., and Stucki, J. W. (1999). The impact of structural Fe(III) reduction by bacteria on the surface chemistry of smectite clay minerals. Geochimica Cosmochimica Acta 63 (22), 3705–3713. doi:10.1016/S0016-7037(99)00199-4
Kowalewski, E., Rueslåtten, I., Steen, K., Bødtker, G., and Torsæter, O. (2006). Microbial improved oil recovery—bacterial induced wettability and interfacial tension effects on oil production. J. Petroleum Sci. Eng. 52 (1), 275–286. doi:10.1016/j.petrol.2006.03.011
Krevor, S., de Coninck, H., Gasda, S. E., Ghaleigh, N. S., de Gooyert, V., Hajibeygi, H., et al. (2023). Subsurface carbon dioxide and hydrogen storage for a sustainable energy future. Nat. Rev. Earth and Environ. 4 (2), 102–118. doi:10.1038/s43017-022-00376-8
Kugler, A., and Dong, H. (2019). Phyllosilicates as protective habitats of filamentous cyanobacteria Leptolyngbya against ultraviolet radiation. PLOS ONE 14 (7), e0219616. doi:10.1371/journal.pone.0219616
Kumari, W. G. P., and Ranjith, P. G. (2023). An overview of underground hydrogen storage with prospects and challenges for the Australian context. Geoenergy Sci. Eng. 231, 212354. doi:10.1016/j.geoen.2023.212354
Lagaly, G.(1993). “Reaktionen der Tonminerale,” in Tonminerale und Tone: Struktur, Eigenschaften, Anwendungen und Einsatz in Industrie und Umwelt. Editors K. Jasmund,, and G. Lagaly (Heidelberg: Steinkopff), 89–167. doi:10.1007/978-3-642-72488-6_3
Lankof, L., and Tarkowski, R. (2020). Assessment of the potential for underground hydrogen storage in bedded salt formation. Int. J. Hydrogen Energy 45 (38), 19479–19492. doi:10.1016/j.ijhydene.2020.05.024
Lavie, S., and Stotzky, G. (1986). Adhesion of the clay minerals montmorillonite, kaolinite, and attapulgite reduces respiration of Histoplasma capsulatum. Appl. Environ. Microbiol. 51 (1), 65–73. doi:10.1128/aem.51.1.65-73.1986
Lee, J. H., Khang, G., and Lee, H. B. (1998). Interaction of different types of cells on polymer surfaces with wettability gradient. J. Colloid Interface Sci. 205 (2), 323–330. doi:10.1006/jcis.1998.5688
Li, G. L., Zhou, C. H., Fiore, S., and Yu, W. H. (2019). Interactions between microorganisms and clay minerals: new insights and broader applications. Appl. Clay Sci. 177, 91–113. doi:10.1016/j.clay.2019.04.025
Liu, K., Chen, Q., Yin, Z., Hu, H., and Ding, Z. (2012). Kinetics of leaching of a Chinese laterite containing maghemite and magnetite in sulfuric acid solutions. Hydrometallurgy 125–126, 125–136. doi:10.1016/j.hydromet.2012.06.001
Liu, N., Dopffel, N., and Stepec, B. A. A. (2024). “Impact of microbial biofilms on subsurface energy systems: from oil and gas to renewable energy,” in Petroleum microbiology (Boca Raton, FL: CRC Press).
Liu, N., Kovscek, A. R., Fernø, M. A., and Dopffel, N. (2023). Pore-scale study of microbial hydrogen consumption and wettability alteration during underground hydrogen storage. Front. Energy Res. 11. doi:10.3389/fenrg.2023.1124621
Lünsdorf, N., Abraham, E., and Timmis, N. (2000). “Clay hutches”: a novel interaction between bacteria and clay minerals. Environ. Microbiol. 2 (2), 161–168. doi:10.1046/j.1462-2920.2000.00086.x
McMahon, S., Anderson, R. P., Saupe, E. E., and Briggs, D. E. G. (2016). Experimental evidence that clay inhibits bacterial decomposers: implications for preservation of organic fossils. Geology 44 (10), 867–870. doi:10.1130/G38454.1
Merchant, S. S., and Helmann, J. D. (2012). Elemental Economy: microbial strategies for optimizing growth in the face of nutrient limitation. Adv. Microb. physiology 60, 91–210. doi:10.1016/B978-0-12-398264-3.00002-4
Napper, D. H. (1983). Polymeric stabilization of colloidal dispersions, 3. London: Academic Press (Colloid science.
Neumann, A., Hofstetter, T. B., Skarpeli-Liati, M., and Schwarzenbach, R. P. (2009). Reduction of polychlorinated ethanes and carbon tetrachloride by structural Fe(II) in smectites. Environ. Sci. Technol. 43 (11), 4082–4089. doi:10.1021/es9001967
Pintelon, T. R. R., Picioreanu, C., van Loosdrecht, M. C., and Johns, M. L. (2012). The effect of biofilm permeability on bio-clogging of porous media. Biotechnol. Bioeng. 109 (4), 1031–1042. doi:10.1002/bit.24381
Reitenbach, V., Ganzer, L., Albrecht, D., and Hagemann, B. (2015). Influence of added hydrogen on underground gas storage: a review of key issues. Environ. Earth Sci. 73 (11), 6927–6937. doi:10.1007/s12665-015-4176-2
Ribeiro, I. D. A., Volpiano, C. G., Vargas, L. K., Granada, C. E., Lisboa, B. B., and Passaglia, L. M. P. (2020). Use of mineral weathering bacteria to enhance nutrient availability in crops: a review. Front. Plant Sci. 11, 590774. doi:10.3389/fpls.2020.590774
Rooney, C., Tappero, R., Nicholas, S., and Li, Q. (2024). Wellbore cement alteration and roles of CO2 and shale during underground hydrogen storage. Appl. Geochem. 170, 106088. doi:10.1016/j.apgeochem.2024.106088
Scappini, F., Casadei, F., Zamboni, R., Franchi, M., Gallori, E., and Monti, S. (2004). Protective effect of clay minerals on adsorbed nucleic acid against UV radiation: possible role in the origin of life. Int. J. Astrobiol. 3 (1), 17–19. doi:10.1017/S147355040400179X
Schulze, D. G. (2002). “An introduction to soil mineralogy,” in Soil mineralogy with environmental applications (John Wiley and Sons, Ltd), 1–35. doi:10.2136/sssabookser7.c1
Shaw, J. C., Bramhill, B., Wardlaw, N. C., and Costerton, J. W. (1985). Bacterial fouling in a model core system. Appl. Environ. Microbiol. 49 (3), 693–701. doi:10.1128/aem.49.3.693-701.1985
Shi, B., Liu, K., Wu, L., Li, W., Smeaton, C. M., Beard, B. L., et al. (2016). Iron isotope fractionations reveal a finite bioavailable Fe pool for structural Fe(III) reduction in nontronite. Environ. Sci. and Technol. 50 (16), 8661–8669. doi:10.1021/acs.est.6b02019
Soccol, C., (2005). Brazilian biofuel program: an overview. J. Sci. and Industrial Res. Prepr. Available at: https://www.semanticscholar.org/paper/Brazilian-biofuel-program%3A-an-overview.-Soccol-Vandenberghe/acafc53c40bd429325033a88c85a9b278f7debc5 (Accessed: December 5, 2024).
Stucki, J. W. (2011). A review of the effects of iron redox cycles on smectite properties. Comptes Rendus. Géoscience 343 (2–3), 199–209. doi:10.1016/j.crte.2010.10.008
Tarkowski, R. (2019). Underground hydrogen storage: characteristics and prospects. Renew. Sustain. Energy Rev. 105, 86–94. doi:10.1016/j.rser.2019.01.051
Tarkowski, R., Uliasz-Misiak, B., and Tarkowski, P. (2021). Storage of hydrogen, natural gas, and carbon dioxide – geological and legal conditions. Int. J. Hydrogen Energy 46 (38), 20010–20022. doi:10.1016/j.ijhydene.2021.03.131
Thaysen, E. M., McMahon, S., Strobel, G. J., Butler, I. B., Ngwenya, B., Heinemann, N., et al. (2023). Corrigendum to “Estimating microbial growth and hydrogen consumption in hydrogen storage in porous media”. Renew. Sustain. Energy Rev. 173, 113039. doi:10.1016/j.rser.2022.113039
Theng, B. K. G.(2012). “Chapter 2 - polymer behaviour at clay and solid surfaces,” in Developments in clay science. Editor B. K. G. Theng (Elsevier Formation and Properties of Clay-Polymer Complexes), 47–75. doi:10.1016/B978-0-444-53354-8.00002-5
Torres, M. A., West, A. J., and Nealson, K. (2014). Microbial acceleration of olivine dissolution via siderophore production. Procedia Earth Planet. Sci. 10, 118–122. doi:10.1016/j.proeps.2014.08.041
Truche, L., Jodin-Caumon, M. C., Lerouge, C., Berger, G., Mosser-Ruck, R., Giffaut, E., et al. (2013). Sulphide mineral reactions in clay-rich rock induced by high hydrogen pressure. Application to disturbed or natural settings up to 250°C and 30 bar. Chem. Geol. 351, 217–228. doi:10.1016/j.chemgeo.2013.05.025
Truche, L., Joubert, G., Dargent, M., Martz, P., Cathelineau, M., Rigaudier, T., et al. (2018). Clay minerals trap hydrogen in the Earth’s crust: evidence from the Cigar Lake uranium deposit, Athabasca. Earth Planet. Sci. Lett. 493, 186–197. doi:10.1016/j.epsl.2018.04.038
Uroz, S., Calvaruso, C., Turpault, M. P., and Frey-Klett, P. (2009). Mineral weathering by bacteria: ecology, actors and mechanisms. Trends Microbiol. 17 (8), 378–387. doi:10.1016/j.tim.2009.05.004
Wang, C., Myshkin, V. F., Khan, V. A., and Panamareva, A. N. (2022). A review of the migration of radioactive elements in clay minerals in the context of nuclear waste storage. J. Radioanalytical Nucl. Chem. 331 (9), 3401–3426. doi:10.1007/s10967-022-08394-y
Wolff-Boenisch, D., Abid, H. R., Tucek, J. E., Keshavarz, A., and Iglauer, S. (2023). Importance of clay-H2 interactions for large-scale underground hydrogen storage. Int. J. Hydrogen Energy 48 (37), 13934–13942. doi:10.1016/j.ijhydene.2022.12.324
Yekta, A. E., Pichavant, M., and Audigane, P. (2018). Evaluation of geochemical reactivity of hydrogen in sandstone: application to geological storage. Appl. Geochem. 95, 182–194. doi:10.1016/j.apgeochem.2018.05.021
Zhang, J., Dong, H., Liu, D., and Agrawal, A. (2013). Microbial reduction of Fe(III) in smectite minerals by thermophilic methanogen Methanothermobacter thermautotrophicus. Geochimica Cosmochimica Acta 106, 203–215. doi:10.1016/j.gca.2012.12.031
Zhang, L., Gadd, G. M., and Li, Z. (2021). “Chapter Four - microbial biomodification of clay minerals,” in Advances in applied microbiology. Editors G. M. Gadd,, and S. Sariaslani (Academic Press), 111–139. doi:10.1016/bs.aambs.2020.07.002
Zhong, X., and Wu, Y. (2013). Bioclogging in porous media under continuous-flow condition. Environ. Earth Sci. 68 (8), 2417–2425. doi:10.1007/s12665-012-1926-2
Zhou, C. H., Zhao, L. Z., Wang, A. Q., Chen, T. H., and He, H. P. (2016). Current fundamental and applied research into clay minerals in China. Appl. Clay Sci. 119, 3–7. doi:10.1016/j.clay.2015.07.043
Zivar, D., Kumar, S., and Foroozesh, J. (2021). Underground hydrogen storage: a comprehensive review. Int. J. Hydrogen Energy 46 (45), 23436–23462. doi:10.1016/j.ijhydene.2020.08.138
Keywords: underground hydrogen storage, biogeochemistry, clay minerals, microorganisms, bioclogging
Citation: Clark A and Sharma S (2025) Relationship between clay minerals and microorganisms in underground hydrogen storage reservoirs: a mini review. Front. Energy Res. 13:1515463. doi: 10.3389/fenrg.2025.1515463
Received: 22 October 2024; Accepted: 21 January 2025;
Published: 07 February 2025.
Edited by:
Tim Mays, University of Bath, United KingdomReviewed by:
Philip Bennett, The University of Texas at Austin, United StatesCopyright © 2025 Clark and Sharma. This is an open-access article distributed under the terms of the Creative Commons Attribution License (CC BY). The use, distribution or reproduction in other forums is permitted, provided the original author(s) and the copyright owner(s) are credited and that the original publication in this journal is cited, in accordance with accepted academic practice. No use, distribution or reproduction is permitted which does not comply with these terms.
*Correspondence: Shikha Sharma, c2hpa2hhLnNoYXJtYUBtYWlsLnd2dS5lZHU=
Disclaimer: All claims expressed in this article are solely those of the authors and do not necessarily represent those of their affiliated organizations, or those of the publisher, the editors and the reviewers. Any product that may be evaluated in this article or claim that may be made by its manufacturer is not guaranteed or endorsed by the publisher.
Research integrity at Frontiers
Learn more about the work of our research integrity team to safeguard the quality of each article we publish.