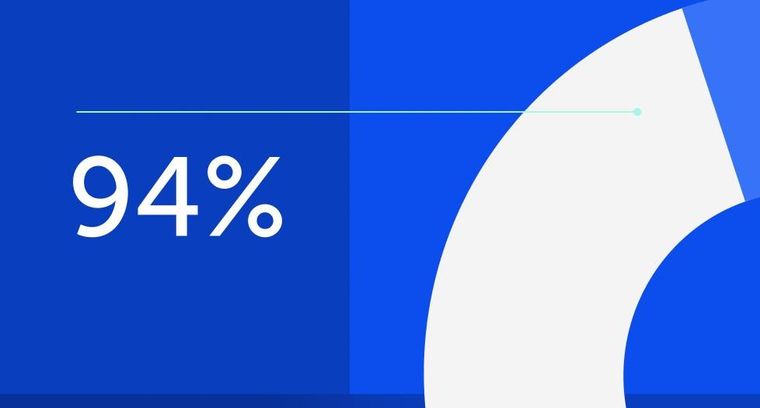
94% of researchers rate our articles as excellent or good
Learn more about the work of our research integrity team to safeguard the quality of each article we publish.
Find out more
REVIEW article
Front. Energy Res., 01 November 2024
Sec. Energy Storage
Volume 12 - 2024 | https://doi.org/10.3389/fenrg.2024.1448520
This article is part of the Research TopicGeneralized Energy Storage in Distributed Energy SystemsView all 5 articles
Energy is an essential factor in many activities. The need to generate adequate energy from various sources is becoming increasingly crucial to meeting the rising needs of the world’s population. Nevertheless, energy storage plays a vital role in meeting the energy demand, notably since affordable yet eco-friendly sources should meet it. Several recommendations were provided to overcome this limitation, with an increasing emphasis on energy sources. However, ecologically sustainable, and effective energy storage systems are the primary focus. Carbonaceous substances produced by pyrolyzing biomass, such as biochar, have recently gained attention as a sustainable material with the potential to be used in electrochemical energy storage technologies. It is an attractive option for electrode materials in supercapacitors, batteries, and hydrogen storage devices due to its abundant availability and distinct physicochemical characteristics, which include, excellent electric conductivity, tuneable surface functional groups, a densely porous structure, a high surface area, porosity, chemical stability, and pore volume. This review addresses the electrochemical performance, production, and characterization of materials based on biochar for energy storage developments. It investigates the choice of feedstock, various preparation routes, various controlling parameters for producing biochar, the biochar activation process, and post-treatment techniques that affect the electrochemical and structural characteristics of biochar for energy storage device fabrication in detail. Additionally, it reveals that recent developments in biochar modification methods like doping, activation, and hybridization have improved the material’s capacity for energy storage. Furthermore, an in-depth discussion on the environmental impacts of biochar-based energy storage devices is elaborated, along with the opportunities and challenges presented in this study.
Most nations greatly rely on fossil fuels due to the great global demand for electricity, which surpasses the current supply, so producing notable carbon emissions. Given its several environmental benefits and capacity, biochar-based energy storage has become a potential option among the several methods under discussion (Bi et al., 2019). This innovative approach to energy storage has gained significant attention and recognition. With these emerging technologies on energy storage application devices, it is possible to store energy sustainably. These technologies aim to address energy storage challenges, such as efficiency, cost-effectiveness, and environmental impact. Several promising advancements have been made using biochar, including lithium-air batteries, solid-state batteries, flow batteries, hydrogen storage, thermal energy storage, and supercapacitors (Usha Rani et al., 2020). Non-renewable energy sources have traditionally played a significant role in meeting global energy demands. They have been used extensively for power generation, transportation, commercial and domestic heating, and industrial processing. Serious concerns such as greenhouse gases, climate change, and severe air pollution call non-renewable energy sources’ viability into question. To address these issues, various countries are working together to increase the share of renewable energy sources in total energy production. Renewable energy sources, such as solar, hydro, wind, and geothermal energy, can be used instead of non-renewable energy sources since they are cleaner and more abundant (Rahman et al., 2022). Figure 1A depicts the increased power energy consumption in various countries over time, whereas Figure 1B depicts the energy sources and their growing consumption percentage. Renewable energy sources began to play a major role in addressing global energy demand. Despite their ubiquity, these sources’ inconsistent reliability, high maintenance costs, geographical and temporal changes, and unpredictable natural events make finding practical applications challenging. For example, solar energy generation requires regular exposure to direct sunshine, which presents issues owing to geographical and temporal fluctuations worldwide (Gupta et al., 2019). In Gulf Arab states like Oman, oil and gas sources provide for 99% of electrical energy output. In Oman, the reliance on gas and oil for energy generation is even higher, at 99.4%. By 2040, it is expected that Oman’s usage of gas for energy grid will increase by 28% (Al-Sarihi Aishaand Bello, 2019). Over the millennia, the use of non-renewable minerals for energy generation has caused significant environmental damage. Choosing renewable energy sources such as solar and hydropower-based techniques is quite helpful. Several countries throughout the world have started to adopt renewable energy sources. Regardless of the source of energy, a good energy storage device is critical for overcoming the restrictions of the power grid, energy waste in transmission lines, and associated costs.
Figure 1. (A) Year-on-year change in electricity demand by region, 2019–2025 (World Energy Outlook, 2022, n.d.), (B) Percentage of energy consumption by source in the World (Energy Institute Statistical Review of World Energy, 2023) (C) Applications of biochar in various fields (Xiang et al., 2021). (D) The keyword “biochar” contains publications per year (Kumar et al., 2023).
Off-grid energy storage technologies, such as smart grids, are required to reconcile these gaps. On-grid energy generation and storage systems are also required (Chakraborty et al., 2022). Every year, the installation of energy storage devices becomes increasingly demanding. Meeting this rising need will require the development of specialized devices and systems dedicated to efficient energy storage (AL Shaqsi et al., 2020). Various sorts of energy storage systems, such as mechanical, chemical, thermal, electrical, superconducting magnetic, and so on, are already in use (Rawat et al., 2023). Figure 2 depicts the present trends and innovations of the energy storage devices technology. Among these devices, electrochemical energy storage devices (EESDs) have the most potential to contribute to sustainability. EESDs operate mainly through energy or power density. Most EESDs rely heavily on carbon materials. These substances are frequently coal or petroleum-based, necessitating a lot of energy and complex synthesis techniques. As a result, eco-friendly renewable carbon compounds with high performance are being investigated. In this context, biomass-based carbon, known as biochar, has numerous surface functional groups and varies in porosity, making it a promising long-term carbon source.
Biochar is currently being extensively explored by researchers all over the world due to its applications in a range of disciplines, including waste management, soil amendment, water purification, and so on, as shown schematically in Figure 1C. It is further emphasized by biochar-related articles published during the last few decades, as seen in Figure 1D. Biochar’s flexible surface chemistry and porosity make it an ideal energy storage and conversion medium (Liu et al., 2019). Biochar has a range of functional groups and heteroatoms that increase the electrode’s electrochemical activity and capacitance, making it appropriate for potential energy storage and conversion applications such as batteries, supercapacitors, fuel cells, and hydrogen production. It can be modified and adjusted to satisfy a wide range of energy applications and performance requirements (Liu et al., 2019).
The growing demand for renewable and sustainable carbon sources that can replace traditional graphite and activated carbon is encourages biochar’s innovation and expansion in the energy sector. Biochar offers numerous advantages as an electrode material for energy storage devices, including high porosity, huge surface area, a diverse variety of functional groups, and heteroatom doping. Biochar can also be easily tailored to meet the needs of various energy applications and performance specifications. Biochar can be transformed into a highly efficient electrochemical energy storage system by utilizing the relevant modification techniques (Zhang et al., 2022). Hence, in terms of cost-effectiveness and ecologically friendly substitutes, biochar will be a good competitor in the search of sustainable electrochemical energy storage. Particularly this kind of environmentally friendly electrochemical energy storage systems have a separate market and great demand. By 2024, biochar is predicted to be a developing and exciting area of research and development as well as a practical and long-term fix for adaption and mitigating of climate change. Some estimates place the worldwide biochar market at USD 2538 million by 2030, rising at 13.9% compound annual growth rate between 2024 and 2030 Biochar2024 | US Biochar Initiative (biochar-us.org).
In this framework, the present review study addresses right from historical developments of biochar, its preparation pathways, and critical factors for adapting biochar into electrochemical energy storage devices (EESD) in detail. Moreover, the application of the biochar and its composites as an EESD, storage capacity, and performance evaluation tool noted in the literature also expands. At end, the present review addresses the life cycle evaluation, long-term sustainability, environmental implications, and future research opportunities.
Ancient Amazonian civilizations developed dark and rich soils known as terra preta by smoldering agricultural waste in pits or trenches, resulting in biochar. In 2005, Peter Read coined the term biochar to describe a substance that resembles charcoal but is intended to improve soil performance and reduce greenhouse gas emissions. Biochar, sometimes known as “black gold,” holds a unique place in the history of human-nature interaction. Its origins are millennia old, with evidence of its deliberate use in agriculture by archaic tribes dating back to 9,000 BC in the Amazon Basin. From improving African soils to lowering carbon emissions in prehistoric China, biochar demonstrates our forefathers’ keen grasp of environmental management. Unlike traditional graphite and activated carbon, it is derived from abundant and renewable biomass sources (Kong et al., 2014; Sudhan et al., 2016). Gasification, hydrothermal carbonization, torrefaction, flash carbonization, and fast and slow pyrolyzed biomass are among the several methods used in biochar production. Production techniques and factors like pressure, temperature, heating rate, particle size, residence time, catalysts, feedstock composition, carrier gas flow rate, reactor structure, and moisture content determine the physicochemical processes yield of biochar (Rathnayake et al., 2021) and varying combinations of feedstock (Jerry Antal, et al., 2003). The several methods of preparing biochar are described in this part. Figure 3 illustrates the key process parameters and the several pyrolysis techniques used in the manufacture of the biochar.
Carbonaceous materials such as biomass, coal, or organic waste can be gasified via a thermochemical process known as gasification (Molino et al., 2018). It involves partial oxidation of the feedstock at high temperatures of approximately 700°C–900°C in the presence of a precisely controlled amount of oxygen or steam to produce solid residue, also known as char and syngas. The gasifying medium can be oxygen, air, or supercritical water. During gasification, a series of processes occur that include drying, pyrolysis, combustion, and reduction to produce biochar. The drying process serves to generate steam from the biomass’s limited water supply. Pyrolysis is a process that converts big molecules into hydrocarbons, which is used to create charcoal, gas, and oil. To obtain biochar, the gasification process should be completed at 573 K. The combustion zone produces both CO and CO2. The final reduction zone promotes gas generation rather than biochar production. Torrefaction aids in the conversion of biomass into char for use as solid fuels. Torrification of biomass typically occurs in inert environments at temperatures ranging from 200°C to 300°C. Torrefaction is classified as dry or moist. Dry torrefaction occurs when a carrier gas such as nitrogen or carbon dioxide is present. Wet torrefaction involves heating solid biomass to 180ºC-260°C after treating it with dilute acids and hot compressed water. The acids and hot compressed water help derivatives of furfural compounds develop as aldehydes, resulting in humins. Typically, this technique allows fuels to have higher calorific values. However, a higher ash percentage in the produced biochar restricts the process’s utility.
Pyrolysis is a thermochemical, non-oxidative process that usually occurs at 400°C–600°C without oxygen (Bridgwater, 2007) and it has the versatility to convert any carbon-containing organic material into biochar (Zaman et al., 2017). During the pyrolysis process, the product is separated into three fractions: solid biochar, non-condensable syngas, and condensable biooil. Biomass pyrolysis includes multiple concurrent and sequential events, including depolymerization, dehydration, isomerization, decarboxylation, aromatization, and charring (Vamvuka, 2011). Typically, the pyrolysis of biomass occurs in three steps, including: (i) the evaporation of free moisture, (ii) the decomposition process, followed by (iii) secondary reactions including oil cracking and depolymerization (White et al., 2011; Kan et al., 2016). The initial decomposition that takes place at a temperature ranging from 200°C to 400°C to form a solid char, where a large amount of biomass gets degraded (Fisher et al., 2002). As the temperature continues to rise, secondary reactions continue to occur within the solid matrix of the biomass. This further results in the disintegration of materials formed due to the limited thermal stability of chemical bonds (Vamvuka, 2011). The produced liquids and gases are unstable which further causes cracking reactions. During the cracking reaction, the dissociated molecules recombine with volatile molecules to form a stable secondary product, known as char.
The heating rate is another critical parameter in the preparation of biochar. The composition of pyrolytic products is influenced by the heating rate (Al-Rumaihi et al., 2022). Typically, the pyrolysis is classified into slow, intermediate, fast, and flash pyrolysis. Among the two processes, the slow pyrolysis process helps in the formation of biochar (Wang et al., 2020). Slow pyrolysis usually occurs between 300ºC and 550°C, at a very slow heating rate (0.1–0.8 C/s) with extended residence time from 5 min up to 35 h. The highly organic carbonaceous biomass produces a high-yield biochar whereas the mineral-rich biomass produces low-yield biochar. The intermediate pyrolysis occurs between 450ºC and 550°C and little bit faster than slow pyrolysis. This process helps in converting the biomass which is bigger like pellets. The fast pyrolysis and flash pyrolysis process is a rapid process that occurs in temperatures ranging from 10°C–1,000°C, and >1000°C, at a residence time of 0.5–2 s, and <0.5 s, respectively. The biochar yield is generally less in fast and flash pyrolysis process (Zhou et al., 2021; Wang L. et al., 2023). A longer period of residence time results in higher production of biochar since it allows for a greater chance of polymerization (Chen et al., 2021a). Cycling stability and capacitance retention are essential parameters for supercapacitors in applications. The carbonization temperature has a significant influence on graphitization and hard carbon interlayer spacing. The reversible intercalation of sodium ions (Na+) requires a rising graphitization degree and decreasing interlayer distance with temperature. The ideal interlayer spacing for effective sodium ion storage is around 0.4 nm at temperatures ranging from 1,000°C to 1,600°C, with the greatest capacity seen at 1,400°C (Huang et al., 2023). The lignin-derived carbon monolith (LSCM) with active carbonyl groups and increased interlayer spacing. This oxalate pyrolysis process demethylates methoxyl groups and cleaves carboxyl groups to create C-O groups and CaC2O4 degrades at 480°C and 700°C, producing CO and CO2 in a staged-uniform manner that results in the largest interlayer spacing. This indicates a high lithium storage capacity, improved rate capability, and improved cycle performance. With an impressive energy/power density of 78 Wh kg−1/100 W kg−1, the developed lithium-ion capacitor device exhibits exceptional longevity, as seen by its capacitance retention of 84.6% even after 20,000 cycles (Wang C. et al., 2023). At 700°C, generated CaO combines with cyanamide units of graphitic carbon nitride (g-C3N4) to form an edge-nitrogen rich lignin-derived carbon nanosheet framework (EN-LCNF), which is then incorporated into the meso-/macropores of the carbon nanosheet framework via sp3-hybridized C-N linkages. The high edge-nitrogen level (7.0 at. %) results in a high-rate capability of 126.4 mAh g−1 at 50 mAg−1, a large capacity of 310.3 mAh g−1, and an extended cycle life. EN-LCNF. The built potassium-ion hybrid capacitors (PIHCs) with EN-LCNF anode and commercial activated carbon cathode exhibit outstanding capacitance retention of 98.7% at a power density of 100 W kg−1 and a high energy density of 110.8 Wh kg−1 (Wang et al., 2024).
A new technology attracting attention for its effective method of converting biomass into biochar with minimal energy is microwave pyrolysis. Uniform heating of biomass helps to make biochar by way of effective heat transfer rate during the microwave pyrolysis process. Unlike conventional pyrolyzes, microwave pyrolyzes the heat from biomass’s surface and helps biomass to be successfully transformed into biochar. Comparatively to the biochar formed by classical pyrolysis, the biochar generated by microwave pyrolysis has a higher surface area (∼800 m2/g) and numerous functional groups, according a study (Naji and Tye, 2022). Among various methods, pyrolysis-mediated biochar preparation is gaining interest due to its ability to minimize the carbon footprint (Li et al., 2021). Furthermore, the scalability and low economics of pyrolysis make it a preferred technique for the biochar production process (Rodriguez-Narvaez et al., 2019).
Biochar alone would be ineffective as an energy storage device. With adequate biochar characterisation, such as surface, intrinsic, and external changes, it has the potential to be an outstanding electrical energy storage device. This is a current trend of creating hierarchical biochar electrode materials for supercapacitors (Li et al., 2021). In most circumstances, biochar will inherit the unique structure of the precursor, such as that of the fibers (Zhao et al., 2020), and carbon skeleton structure (Wang et al., 2017). These techniques include activation, functionalization, the production of composites, and others (Cheng et al., 2017). Lower pyrolysis temperatures result in the production of low-energy biochar. It produces a product that is heavier but has less carbon in it. Higher pyrolysis temperatures result in the production of high-energy biochar. A product with a higher carbon content is the outcome (Sajdak et al., 2022). Lower temperature-produced biochar often has lower pH, less porosity, less developed specific surface area, and a larger proportion of volatile substances and capacity for cation exchange (CEC). The synthesis of biochar with a highly developed specific surface area, high porosity, greater pH, and higher ash and carbon content is encouraged by high pyrolysis temperatures. Nevertheless, its volatile matter content and CEC values are lower (Tomczyk et al., 2020).
Activation mainly increases biochar’s surface area and porosity, enabling it to store and release energy more efficiently by the charge and discharge mechanism of static double-layer capacitance or electrochemical pseudo-capacitance (Senthil et al., 2019). Different biochar activation processes can be employed to enhance its characteristics (Sakhiya et al., 2020). This can lead to an increase in the energy density of the biochar. Several methods can be employed to achieve this, including activation through physical, chemical, or impregnation processes (Mehdi et al., 2022). Physical activation takes place in two stages. Initially, the biomass is carbonized at an appropriate temperature (400°C–450°C) in an inert environment with activators consisting of either air (600°C), steam, or carbon dioxide (up to 1200°C) (Senthil et al., 2019). The biochar created by the mentioned thermochemical methods frequently needs to be physically or chemically activated to increase its surface properties, as well as other characteristics like specific surface area or porosity that eventually improve its use in charge storage applications (Chu et al., 2022). With ammonia, steam, CO2, or a small amount of air while maintaining temperatures between 600°C and 900°C, biochar is physically activated (Rawat et al., 2022). The air-activation method resulted in carbon sheets having a significantly higher specific surface area of 2,396 m2 g−1 (Ding et al., 2015). In one study, grape seeds were treated to cyclic oxidation and desorption to create biochar. The oxidants used included air, ozone, and nitric acid. The produced carbon material’s pore size and pore volume distributions can be changed using the right operational parameters, such as the oxidation process and the number of oxidation/desorption cycles (Jiménez-Cordero et al., 2014). Even extremely high temperatures are required for physical activation, the diversity of activators used in the process makes it simple to run and environmentally friendly.
Emphasizing the flexibility and probable application of these materials in energy storage uses, Supplementary Table S1 shows a spectrum of feedstocks and process conditions. The variety of activators and electrolytes used, as well as the many applications. Engineers and researchers may find significant utility in this table on energy storage. Table shows cattle bones have the highest capacitance of any foodstuff at 1230 Fg−1. This feedstock consists of hydroxyapatites to promote self-activation which a process requiring 1 hour at 1100°C. Used in lithium-ion batteries, the final product is Greater capacitance a capacitor will be able to store more energy than a smaller capacitance capacitor as the charge a capacitor can store is exactly proportional to its capacitance. Thus, increasing the capacitance of a capacitor allows it store more energy, which can be favourable in usage including energy storage systems where high energy density is needed. Still, it is crucial to stress that the capacity of energy that might be stored also depends much on the voltage across the capacitor. The capacitance of a capacitor essentially determines its capacity for energy storage; so, it is essential to enhance this value to maximize the performance of energy storage devices.
Although both supercapacitors and batteries are energy storage devices, they operate in fundamentally different ways and have different strengths. Supercapacitors store energy in an electrostatic field, whereas batteries store energy through a chemical reaction. Batteries have a higher energy density, which means they can hold more energy per unit mass. This makes batteries valuable for applications that require sustained power for an extended period. Because supercapacitors have a higher power density, they can deliver or absorb energy faster than batteries. As a result, they are well-suited for applications that require rapid charge/discharge cycles. Supercapacitors charge and discharge much faster than batteries. They do, however, tend to release their stored energy more quickly when not in use. When compared to batteries, supercapacitors typically have a longer lifespan and can withstand more charge/discharge cycles (Zhang et al., 2023). The standard batteries lifecycle varies from 300 to 30,000 cycles depends upon the type and usage conditions of the batteries. Whereas the supercapacitors can withstand up to one million cycles generally (Rashid Khan and Latif Ahmad, 2024; Xing et al., 2024). In the circumstance of chemical activation, the biochar is pre-combined with a chemical agent before undergoing thermal treatment, which is typically performed at temperatures ranging from 400° to 900°C (Cheng et al., 2017). Chemical activation can occur in either one step or two steps. The biomass precursors are combined with the proper activator during a single-step chemical activation process. Direct heating at temperatures ranging from 450°C to 900°C in an oxygen-free atmosphere is required to produce activated carbon (Senthil et al., 2019). Potassium chloride (KCl) has a substantial impact on the activation process of carbon derived from tobacco stems. It changes into potassium carbonate (K2CO3), which plays a critical role in building porous structures, increasing the oxygen content, facilitating crosslinking, and producing micropores. These aspects contribute to the exceptional performance of the final carbon material in supercapacitors. The authors reported the specific capacitance and energy density achieved as 320 Fg−1 and 10.68 Wh/kg with 98.88% capacitance stability after 10,000 cycles (Li et al., 2024). The 3D honeycomb structure of hemp-derived activated carbon resulted in a very wide surface area with decreased surface functional groups, resulting in a high specific capacitance of 240 Fg−1 at 1 Ag−1 and was discovered to be highly reversible with an excellent retention rate of 98%. The obtained pore sizes are highly efficient in double-layer production and correspond closely to the size of hydrated ions (Minakshi et al., 2024a). The study reported that the honey dew (Cucumis melo Inodorous group) fruit peel-derived activated carbon had been effectively synthesized at the optimal combination of carbonized honey dew fruit peel to H3PO4 ratio (1:4) and activation temperature of 500°C. The insertion of hetero atoms (Phosphorous) and oxygen surface functions into the cellulose enables for the tailoring of electrochemical performance in supercapacitors (Minakshi et al., 2024b).
The biomass precursor is first subjected to a two-step process that involves pre-treatment at 400°C–450°C. Following that, pre-carbonized material is combined with chemical activators, and the mixture is heated inertly at 650°C–900°C. The result of the chemical activation processes is the products being cleaned with pristine water. When contrasting the advantages of chemical and physical activation, chemical activation is superior due to its shorter higher yields, activation times, lower activation temperatures, (meso- and macro-) pore networks, and larger surface area (Senthil et al., 2019). However, it can be corrosive and harmful to nature because of its harsh chemical usage. Chemical activation, when compared to the physical activation method, has some disadvantages, such as high costs associated with chemicals and it poses challenges in recovering these chemicals (Cheng et al., 2017). Multiple activating agents or activators can be used for the process. Among several activation agents, KOH is favorable because of its advantages, including higher yields, a lesser activation temperature, and the production of a higher surface area and pore volume (Senthil et al., 2019). Chemical activation improves the surface properties of the sludge biochar used in electrode manufacture, but at the expense of decreased electrical conductivity, wettability, and carbon and nitrogen concentrations (Liu et al., 2019). Research reveals that hard carbon created from waste hemp through mild chemical activation with KOH at 450°C demonstrates outstanding electrochemical performance as an anode in hybrid supercapacitors. The biomass hemp powder activated with melted KOH solution serves as both a deoxidant and an activating agent, lowering the demand for high temperatures that permit molecular-level activation of hemp during the subsequent one-step calcination at 450°C (Minakshi et al., 2024a).
Physicochemical activation is a process that combines physical and chemical activation to produce biochar with a large surface area and pore volume. It is a two-step process that includes, the impregnation of the biochar with a chemical activating agent and thermal treatment of the impregnated biochar. The most common chemical activating agents used in physicochemical activation are acids (such as H2SO4 and HCl), bases (such as NaOH and KOH), and salts (such as ZnCl2 and FeCl3). The biochar undergoes a reaction with the chemical activating agent, which results in the formation of pores and an increase in surface area. After that, the biochar that has been impregnated is heated to a very high temperature (typically between 400°C and 600°C) in a vacuum. Because of the heat, the chemical activation agent degrades, leaving pores and increasing the biochar’s surface area. Physicochemical activation outperforms physical or chemical activation in terms of creating biochar with a large surface area and pore volume. This is since the chemical activating agent produces smaller and more uniform pores than physical activation. The smaller and more regular pores increase the sorption capacity of the biochar, making it more appropriate for applications such as water filtration and gas adsorption (Do et al., 2023). Figure 4 depicts the various biochar activation methods.
Figure 4. Advantages and disadvantages of different activation methods (Xu et al., 2021; Panwar and Pawar, 2022).
Surface modification via functionalization is another useful method that involves introducing specific functional groups or coatings onto the biochar surface (Usha Rani et al., 2020). To meet specific application requirements, biochar is functionalized to enhance surface area, altering the surface characteristics with new functional groups, and thereby producing biochar matrix composites (Li et al., 2020). This modification enhances its electrochemical properties, improving energy storage and conversion capabilities (Li et al., 2021). Biochar has inherent surface functional groups, particularly oxygenated groups, in contrast to other carbon-based substances such as activated carbon, graphene, and carbon nanotubes (Cheng et al., 2017). Numerous research has demonstrated that adding heteroatoms to carbon-based materials’ surfaces can increase the capacitance of supercapacitors. The presence of atoms such as nitrogen, phosphorus, and oxygen on the surface of an electrode promotes the combination of the electrode with the ions in an electrolyte solution. This presence also has the potential to raise the wettability of the electrode material concerning the electrolyte solution. Post-synthetic surface functionalization and doping are being performed on biochar in the research literature to customize biochar for a variety of applications. The synthetic porous carbon quasi-nanosheet has a notable mesopore volume that improves charge storage and ion transport, along with a high specific surface area of 1069 m2/g and a total pore volume of 1.375 cm³g−1 (Fu et al., 2020).
Materials that function as pseudo-capacitors include transition metal oxides and hydroxides like MnO2, NiO, and Co(OH)2, amongst others. Biochar can be utilized as a substrate to anchor nanoscale metal oxides and hydroxides, and it possesses electric double-layer capacitance and pseudo-capacitance properties (Li et al., 2021). For example, Wan et al. (2016) employed biochar generated from wood as a substrate to grow nanosheets of manganese dioxide (MnO2) by performing an in-situ redox reaction between the biochar and potassium manganese dioxide (KmnO4). The formed MnO2 was evenly dispersed on the biochar’s surface, creating a core-shell structure. The MnO2/biochar composite unveiled a higher specific capacitance (101 Fg−1) compared to biochar alone (19 Fg−1) and exhibited excellent cyclic stability, retaining 85% of its capacitance even after 10,000 cycles. Common transition metal oxides utilized in hybrid capacitors include V2O3, RuO, NiO, FeO, Fe2O3, Co3O4, CoO, MnO, and VO. Among the transition metal oxides utilized in batteries and supercapacitors, MnO2 possesses several advantages, including its abundance on Earth, cost-effectiveness, environmental acceptability, and a high theoretical capacitance of 1370 Fg−1 (Wickramaarachchi and Minakshi, 2022). Techniques such as surface oxidation, amination, and sulfonation have been used to adjust the surface functionality. These modifications have shown promise in enhancing biochar’s adsorption capacity, catalytic performance, and nitrogen doping for various applications, including wastewater purification and catalysis (Li et al., 2021). In a study that was carried out by Yang et al., the nitration and reduction procedure was devised to manufacture amino-modified biochar. This biochar demonstrated improved adsorption of copper ions from synthetic wastewater. FTIR analysis reveals that biochar possesses amino groups, as expected given its composition (Yang et al., 2019). Nitrogen doping considerably improves the electrochemical activity of carbon compounds. Nitrogen molecules with higher electrochemical activity, like N-6 and N-5, enable faster Faradic reactions and consequent charge storage. As a result, the pseudo-capacitance of nitrogen-doped porous carbons is increased (Fu et al., 2022).
As mentioned earlier, functional groups have favorable reactivity towards various nanostructures, enabling the formation of high-performance composites. For instance, biochar can act as a substrate to help solve the problems of aggregation and solubility that are associated with carbon nanotubes (CNTs). By using bamboo char as a substrate, CNTs were grown through chemical vapor deposition (CVD) resulting in a CNTs/biochar composite with higher adsorption capacity. It is also possible to combine biochar with layered double hydroxides, often known as LDHs. These LDHs serve as host materials for anion exchange intercalation processes. A fine dispersion of LDH flakes on the surface of the biochar was seen after the composite materials consisting of biochar and MgAl-LDHs had been created employing liquid-phase deposition and self-assembly. These composites demonstrated a supreme acceptance capacity of 410 mg/g for phosphate surpassing other LDH adsorbents (Li et al., 2021).
Another approach is composite formation, where biochar is combined with other materials to enhance its overall performance. For instance, incorporating biochar with nanotubes or graphene can boost its conductivity and improve charge/discharge rates (Wang et al., 2017). These characterization techniques not only unlock the potential of biochar as an energy storage material but also pave the way for enhanced efficiency and effectiveness in various energy storage applications. The incorporation of heteroatoms into carbon structures through self-inclusion or self-doping from biomass sources is advantageous from both an economic and a structural point of view (Senthil et al., 2019). The specific capacitance that supercapacitors exhibit should, according to the theory, be proportional to the specific surface area (SSA) of the carbon material, and the higher the SSA, the greater the specific capacitance that the carbon material possesses. According to the findings of many studies, a rise in SSA can effectively lead to an increase in the number of active sites (Guo et al., 2021). However, contrary to what one might expect, the specific capacitance of biochar material does not increase linearly with increasing SSA. Electrolyte ions with a large diameter are unable to pass through these extremely small holes, which are principally to blame for the SSA. Numerous studies have demonstrated that materials with a high fraction of micropores do not perform electrochemically as well as they could because the ions, especially those from organic electrolytes, cannot be transported easily via the micro pores between the electrode materials. Electrolyte ions with a high diameter cannot penetrate these tiny holes, which are primarily responsible for SSA (Li et al., 2021). Numerous studies have demonstrated that materials with a high percentage of micropores do not perform electrochemically as well as they should because the micro pores do not allow for the efficient passage of ions between the electrode materials (Chen et al., 2021b). By implementing these characterizations and modifications, biochar can be transformed into a more effective and efficient energy storage device. These enhancements broaden its potential for sustainable energy storage applications while capitalizing on its environmentally friendly nature and other inherent benefits.
The porous structure and surface functional groups of biochar contribute to its hydrogen storage capacity (Sakhiya et al., 2020). Biochar can serve as an electrode material in fuel cells. Its high surface area and electrical conductivity make it suitable for this application. Biochar-based materials have been tested in electrocatalytic oxygen reduction and methanol oxidation in fuel cells (Cheng and Li, 2018; Liu et al., 2019). While there is limited information available on the specific application of biochar in redox flow batteries, biochar’s high surface area, electrical conductivity, and adaptable structure make it a promising candidate for use in these batteries. Redox flow batteries are a type of rechargeable battery where energy is stored in liquid electrolytes. The energy density and power generation capability of these batteries can be decoupled, making them flexible and cost-effective for large-scale applications (Anitha and Ramila Devi, 2023).
To understand the usefulness of biochar for energy storage, several significant properties must be considered, including energy density, power density, scalability, lifespan/cycle, cost, porosity, and surface area, among others. Energy density is the amount of energy that can be stored in a given space depending on its volume or mass, whereas power density is a system’s ability to rapidly deliver or absorb energy (AL Shaqsi et al., 2020). While biochar may not currently match the energy density of some conventional energy storage technologies, its potential lies in its abundance, low-cost production, and potential for carbon sequestration benefits. Biochar functions as an anode material, it will be enhancing lithium-ion intercalation and deintercalation when charging and discharging cycle in the lithium-ion batteries (Norouzi et al., 2019). Figure 5D depicts the general schematic of the battery charging and discharging mechanism. In the supercapacitor, the capacitive charge storage approach depends on the electrostatic adsorption of ions on the biochar surface, which is regulated by its hierarchical porous structure. Figure 5B shows that the electric double layer effect and Figure 5C depicts the faraday process. Based on the pore structure the connected 3D pore network in biochar promotes the ion transport, minimizing resistance and improving the rate charge and discharge (Cuong et al., 2021). Figure 5A shows that the schematic of the supercapacitor. The functional groups give a key impact on ion binding and electrochemical activity, and also boost the energy storage capacity (Liu et al., 2019).
Figure 5. (A) Super capacitor structure diagram. (B) Electric double layer effect schematic. (C) Faraday process schematic. [Adopted from (Dai et al., 2016) http://creativecommons.org/licenses/by/4.0/] (D) charging and discharging schematic of battery.
The terms “electrochemical double-layer capacitors” (EDLCs) and “pseudo capacitors” are both terms that can be used to refer to supercapacitors. By applying an external voltage, electrolyte ions were physically adsorbed and desorbed on the electrode surfaces of EDLCs. Because of this, EDLCs often make use of materials that have a large specific surface area (SSA), fine pore structures, high electrical conductivity, and superior chemical stability (Cheng et al., 2017). Components of electrodes that are typically found in EDLCs include activated carbon, mesoporous carbon, carbide-derived carbon, graphene, and carbon nanotubes (Hall et al., 2010). Redox interactions between the materials that make up the electrodes and the electrolytes allow for the operation of PCs. These reactions take place on the surface of the electrodes. The primary factors that determine the capacitance performance of PCs are the in-built redox reaction, as well as the huge contact surface area that exists between the electrode and the electrolyte. When compared to EDLCs, PCs often have significantly greater charge storage capacity, but they have inferior rate capabilities and cycle stability (Cheng et al., 2017). According to the findings of the study, lotus leaves were pyrolyzed in a tube-shaped furnace at a temperature of 700°C. Excellent electrochemical performance is exhibited by the biochar material that is produced (Lu et al., 2020). The biochar produced at 800°C demonstrated a rate capability of 84.1% and a specific capacitance of 228 Fg−1 at 1 Ag−1 in 1 M H2SO4 when the current density was increased to 5 Ag−1, which is 191.9 Fg-1. The unusually high energy density of 7.91 Wh kg−1 in 1 M H2SO4 electrolyte and the good cycle stability of the biochar-800 were both proved by the biochar-800, 88% capacitance retention for a lifetime of 5,000 cycles at a high current density of 10 Ag−1 (Husain et al., 2022).
The researchers created functional biochar from the grass Desmostachya bipinnate to produce electrodes for supercapacitors and microbial fuel cells. They showed that biochar electrodes have excellent capacitance, power density, and energy density in supercapacitors, as well as high power output and coulombic efficiency in microbial fuel cells. They ascribed the biochar electrodes’ better performance to their increased surface area, porosity, and heteroatom doping (Mehrotra et al., 2023). Biochar is a strong contender for use in supercapacitors due to its hierarchical porous structure and heteroatom surface functionalities (Rawat et al., 2023). Micro pores that have a small pore size are the primary contributors to the SSA. Electrolyte ions that have a big diameter are unable to penetrate micropores because of their size. Numerous studies have demonstrated that materials that include a high percentage of micropores do not have perfect electrochemical performance. This is because the micro pores do not promote the movement of ions between electrode materials, particularly organic electrolyte ions. For biochar to effectively store hydrogen, the material must have a sizable surface area, a structure that is dominated by micropores, and oxygen-rich functional groups (Rawat et al., 2023). Micro pores that have a small pore size are primarily responsible for SSA, and electrolyte ions that have a big diameter are unable to enter micropores with a small pore size (Li et al., 2021).
Figure 6 indicates that the curve for the EDLCs material presents a rectangular-type cyclic voltammetry (CV) curve and galvanostatic charge-discharge (GCD) curve with a linear potential relative to time, where b = 1, as mentioned in Figure 6A. Pseudocapacitive materials are expected to display nearly rectangular cyclic voltammograms, possibly demonstrating inflections, but not flat curves, with b nearly equal to 1, as seen in Figure 6B. Conversely, in batteries, CV generally reveals redox peaks, whereas GCD demonstrates plateaus (b = 0.5) refer to Figure 6C.
Figure 6. A typical electrochemical behavior that distinguishes the (A) EDLC, (B) pseudocapacitance and (C) batteries. Reproduced with permission copyright 2019 John Wiley and Sons (Jiang and Liu, 2019).
Battery performance depends on effective ion movement made possible by the microporous structure of biochar (Bartoli et al., 2024). The reasonable surface area of the biochar material is excellent for electrochemical processes in Li-ion batteries to improve the diffusion of Li-ion because of the large electrolyte gap in Li-ion batteries (Wang et al., 2016). Puffed rice carbon (biochar) and commercial Sn and Se powder were utilized to create SnSe/PRC anodes for lithium-ion batteries using a high-energy ball grinding process for 48 h in an inert argon environment at 600 r/m. Puffed rice carbon, Sn, and Se powder were extruded together by high energy mechanical force to construct an alloy/carbon inlaid structure, which increased electrical conductivity, buffered the huge volume expansion effect, and improved structural stability (Su et al., 2019). The porous biochar synthesized showed increased specific surface area and well-developed porous structure, with moderate heteroatom doping. This enhanced specific capacitance and energy density, making it 1.6 and 6.0 times higher than samples carbonized directly by molten salt and inert reduction methods. The assembled two-electrode symmetric supercapacitor showed excellent stability after 10,000 cycles (Lei et al., 2021). The biochar-based electrodes shows that the higher charge storage capacity up to 14.14 m2g−1 from the results from increased surface area (Naseem et al., 2024). A three-dimensional network in some biochar lets electrons flow and fits volume changes during cycling (Yang et al., 2024).
The life cycle is another critical property, denoting the number of discharge-charge cycles a storage system can endure while maintaining its performance. A longer cycle life ensures the durability and longevity of the device, reducing the need for frequent replacements (Trahey et al., 2020). Cost is an influential aspect that must be considered, encompassing factors such as materials, manufacturing processes, and overall market competitiveness. Scalability is a crucial property, signifying the adaptability of the energy storage technology to various scales, from small-scale residential applications to large-scale grid-level deployments (Hunt et al., 2022). The pyrolysis process cost is much higher when comparing bioprocess, it’s around 36% of the total cost, and the feedstock collection cost is around 12%, also the transport cost maybe 9% of the total cost (Homagain et al., 2016). Lastly, safety is of utmost importance, encompassing the stability of the storage system and its capacity to handle high currents or temperatures without compromising security (Trahey et al., 2020). By meticulously evaluating these properties and employing suitable characterization techniques, researchers, engineers, and industry experts can develop efficient and reliable energy storage devices that meet the demands of specific applications, considering factors such as intended purpose, constraints, and desired performance characteristics (Poulose et al., 2018; Thomas et al., 2021). In the environmental safety aspect, biochar employing plentiful and sustainable biomass as the raw material lessens the impact on the environment and the need for fossil fuels. After usage, they may be readily recycled or biodegraded, which eliminates the issue of pollution and waste disposal (Parsimehr et al., 2023).
The sample reportedly attained a specific mesopore volume of 1.198 cm³g−1. This volume makes up around 87.1% of the total pore volume, indicating that mesopores primarily affect the material’s structure. The mesopore generation and overall textural quality of the carbon material are enhanced by the templating action of ZnO nanoparticles during the synthesis process, which accounts for the high mesopore volume. Supercapacitors’ enormous mesopore volume and surface area give them increased specific capacitance and rate capability. The three-electrode device shows that a high-performance in supercapacitor since reports state that its specific capacitance should reach 320 Fg−1 at a current density of 1.0 Ag−1 (Fu et al., 2020). The biochar produced has a large surface area, a three-dimensional hierarchical porous structure with micropores and mesopores, and strong electrochemical properties. The biochar electrode has a capacitance of 428 Fg−1, a rate capability of 82.9% at 30 Ag−1, and 96.7% retention after 10,000 cycles in a 6 M KOH aqueous electrolyte (Zhang et al., 2019). The SEM images reported by various researchers of pine lignin before activation (Figure 5A) and pine lignin biochar after activation (Figure 7B) (Li et al., 2018); Figure 7C shows raw biochar without any reduced graphene oxide, and Figure 7D shows biochar loaded with 3.74 weight percent reduced graphene oxide (Rui et al., 2020); biochar made from corn straw (Figure 7E) and porous biochar made from corn straw that has been activated by potassium hydroxide (Figure 7F) (Qu et al., 2021); and the pore structure of biochar (Figure 7G) are presented.
Figure 7. SEM images of biochar samples. (A) Pine lignin biochar, (B) pine lignin activated carbon (Li et al., 2018). (C) Raw biochar without reduced graphene oxide, (D) biochar loaded with 3.74 wt% reduced graphene oxide (Rui et al., 2020). (E) Corn straw biochar, (F) Porous corn straw biochar activated by KOH (Qu et al., 2021). (G) The pore structure of biochar.
Biochar production stands out among the different technologies available as one of the most environmentally beneficial and accessible ways to create electricity. Biochar can be made from a variety of biomass sources, allowing waste materials to be used to generate electricity. This procedure not only helps to reduce waste but also creates energy (Sutar et al., 2022). However, the sustainability of biochar synthesis is greatly dependent on the methods used. It is critical not to neglect the prospective destructive environmental influences of biochar use, considering parameters such as hazardous components, structure, and particle size (Selvam S and Paramasivan, 2022). This section focuses on discussing the mechanisms that contribute to these adverse effects. In 2016, biochar made from sugarcane in Brazil absorbed up to 31% of carbon (2.35 ± 0.4 tons of CO2) (Lefebvre et al., 2020). It is widely acknowledged that biochar has the potential to reduce greenhouse gas emissions, help remove CO2 from the atmosphere, and make the environment more sustainable.
Biochar-based electrochemical energy storage devices require biomass fuel, chemicals, and metals. Biochar-based electrochemical energy storage devices’ major environmental impact is chemical use. Biochar synthesis, activation, and functionalization with chemicals can harm the environment. If mismanaged, these compounds can pollute water and soil and destroy ecosystems. These resources can damage habitats, pollute water, and use energy. Heavy metals in biochar vary by feedstock type. Leaching of heavy metals from biochar generated from biomass containing high concentrations of those metals could cause an increase in the levels of those metals in the environment (Xiang et al., 2021). At higher pyrolysis temperatures, Devi and Saroha observed that biochar had a greater amount of copper, lead, and zinc. These three metals showed increases of 61%, 73%, and 65% respectively as the pyrolysis temperature was raised from 200°C to 700°C (Devi and Saroha, 2014). The decomposition of biomass’s organic components releases heavy metals due to rising temperatures. Thus, biochar heavy metal content varies on pyrolysis temperature. The feedstock used to make biochar affects the process’ environmental impact, as mentioned. One example is bio-toxic polycyclic aromatic hydrocarbons (PAHs). In many environments, PAHs can harm plants and microbes (Devi and Dalai, 2023).
The feedstock and manufacturing temperature affect biochar PAH levels. Hemp-derived biochar has more mutagens than wood-derived. Biochar may also produce dioxins and the preparation parameters affect dioxin emission (Alipour et al., 2021). The research team investigated several dioxins in different forms of biochar. The team discovered biochar, which is produced by a process called slow pyrolysis at temperatures of 250°C and 900°C and has concentrations between 84 and 92 mg/kg. The study found that when food waste is utilized as biomass for biochar, the number of dioxins increases due to the salt concentration. The development of dioxins is influenced by temperature, and it is well known that at high enough temperatures, dioxins are rendered useless above 1000°C (Yaashikaa et al., 2020). To prevent the production of dioxins that may be detected, the initial biomass feedstock should have a chlorine concentration that is as low as possible. High temperature could be utilized to eliminate dioxins, however, as was already indicated, the pyrolysis method used to create the biochar that would be employed for electrochemical energy storage calls for gradual pyrolysis and lower temperatures. Waste production may have an additional effect. These devices’ production processes may produce waste materials, including used electrodes, electrolytes, and packaging (Wiedner et al., 2013). Effective waste management is critical to preventing contamination and reducing the harmful impact of various waste streams on the environment. Lifecycle analysis, supply chain effect, and end-of-life management are all indirect environmental repercussions. Lifecycle analysis considers the environmental effects of raw material extraction, manufacturing, transportation, use, and recycling or disposal. The supply chain repercussions should be investigated because procuring and shipping raw materials for biochar-based energy storage devices may have indirect effects and result in significant carbon emissions.
The LCA considers not only the amount of energy and resources that are consumed but also the amount of waste and emissions that are produced when the product is being assembled. The manufacturing stage in the LCA helps to identify opportunities for improving energy efficiency, reducing resource consumption, and minimizing environmental impacts throughout the entire life cycle of supercapacitors. The last part is the End-of-life management of the device. At this time, the environmental consequences connected with the disposal or recycling of the device’s components, such as biochar, electrodes, and other materials, are being evaluated. There are key aspects considered in the LCA of end-of-life management, such as disposal options, recycling, material recovery, and waste management (Klöpffer and Birgit, 2014). According to the study, each kilogram of biochar applied as a conductive element in lithium-ion batteries has a carbon imprint comparable to 1.2 kg CO2. while employing petroleum-derived carbon black as a conductive component it is 2.4 kg CO2 equivalents per kg of carbon black (Kane et al., 2022). Disposal options include landfilling, incineration, or other waste management practices. The environmental impacts associated with each option, such as emissions, leachate generation, and potential soil or water contamination, are evaluated (Klöpffer and Birgit, 2014). The choice of disposal method can significantly affect the overall environmental footprint.
The scientific community’s emphasis on sustainable energy generation provides a hopeful peek into a green and secure future. Numerous studies have identified biochar as a fantastic energy source with numerous applications. However, optimization of pyrolysis process parameters for various feedstock remains a challenge. Another significant challenge is the use of activation materials, because using chemicals may cause corrosion of devices as well as potentially dangerous impacts on humans. The research is opening on the optimization of the activation process and additives. The nanomaterials have not yet met the need for energy storage efficiency of biochar-based devices. The research on biochar-based energy storage devices’ cost-effectiveness and safety aspects is still ongoing. The performance, scalability, and affordability of energy systems based on biochar are being worked on with continued attention to research and development projects. Unlocking the full potential of biochar for reducing climate change and encouraging sustainable land management practices depends on further study and innovation in this area. These initiatives establish the groundwork for a more resilient and sustainable energy system, where biochar can be crucial in meeting our energy requirements.
Using sustainable solutions for the growing worldwide energy consumption is vital. Derived from biomass, biochar with its carbon-negative methods and carbon sequestration potential shows promises in sustainable energy storage. Rapid modernization and the recent explosion in electric vehicles are predicted to cause notable increase in the worldwide energy storage market. Between 2023 and 2033, the 15.8% Compound Annual Growth Rate worldwide need for energy storage will rise. For energy storage systems, biomass-based carbon compounds as activated carbon show good characteristics. Essential in the pyrolysis process of biochar creation are parameters like temperature, heating rate, residence duration, and feedstock properties. Temperature effects physicochemical characteristics and biochar yield. Production of biochar with required characteristics for particular applications depends on optimizing these factors.
Future usage of biochar-based supercapacitors seems indicated by their great specific surface area, mesoporous content, and hierarchical pore structure. Several techniques exist to activate biochar from a range of feedstocks and produce a valuable product. While pseudo-capacitors use materials that allow redox reactions, EDLCs rely on materials including activated carbon, mesoporous carbon, and graphene. Physical, chemical, and co-activation mechanisms produced biochar as a capacitance material. Thanks to improving advancements, biochar has become a magical material for uses in enhanced energy storage. Though there are certain negative consequences, biochar will be a more ecologically friendly substitute for the now utilized conventional materials for uses in energy storage. More study and development in these areas is needed to realize the full possibilities of biochar-based energy storage systems considering their environmental consequences. All things considered; biochar-based energy storage systems present a viable path for environmentally friendly energy source. By using sustainable methods all through their lifetime and optimizing their environmental benefits, biochar systems can help to promote a better and more resilient future.
PP: Conceptualization, Methodology, Visualization, Writing–original draft. KM: Writing–original draft. LM: Formal Analysis, Supervision, Writing–review and editing. KS: Data curation, Methodology, Writing–review and editing.
The author(s) declare that financial support was received for the research, authorship, and/or publication of this article. The work has been supported by Vellore Institute of Technology and is gratefully acknowledged by the authors.
The work has been supported (Lab and Library Facilities) by Vellore Institute of Technology and is gratefully acknowledged by the authors.
The authors declare that the research was conducted in the absence of any commercial or financial relationships that could be construed as a potential conflict of interest.
All claims expressed in this article are solely those of the authors and do not necessarily represent those of their affiliated organizations, or those of the publisher, the editors and the reviewers. Any product that may be evaluated in this article, or claim that may be made by its manufacturer, is not guaranteed or endorsed by the publisher.
The Supplementary Material for this article can be found online at: https://www.frontiersin.org/articles/10.3389/fenrg.2024.1448520/full#supplementary-material
Alipour, M., Asadi, H., Chen, C., and Rashti, M. R. (2021). Bioavailability and eco-toxicity of heavy metals in chars produced from municipal sewage sludge decreased during pyrolysis and hydrothermal carbonization. Ecol. Eng. 162, 106173. doi:10.1016/J.ECOLENG.2021.106173
Al-Rumaihi, A., Shahbaz, M., Mckay, G., Mackey, H., and Al-Ansari, T. (2022). A review of pyrolysis technologies and feedstock: a blending approach for plastic and biomass towards optimum biochar yield. Renew. Sustain. Energy Rev. 167, 112715. doi:10.1016/J.RSER.2022.112715
Al-Sarihi, A., and Bello, H. (2019). “Socio-economic and environmental implications of renewable energy integrity in Oman: scenario modelling using system dynamics approach,” in Climate change and energy dynamics in the Middle East: modeling and simulation-based solutions. Editor A. A. Qudrat-Ullah Hassan (Cham: Springer International Publishing), 17–46. doi:10.1007/978-3-030-11202-8_2
AL Shaqsi, A. Z., Sopian, K., and Al-Hinai, A. (2020). Review of energy storage services, applications, limitations, and benefits. Energy Rep. 6, 288–306. doi:10.1016/J.EGYR.2020.07.028
Anitha, A., and Ramila Devi, N. (2023). “Prospects of biochar as a renewable resource for electricity,” in Biochar - productive technologies, properties and applications (IntechOpen). Chapter 9, 187–202. doi:10.5772/intechopen.108161
Bartoli, M., Piovano, A., Elia, G. A., Meligrana, G., Pedraza, R., Pianta, N., et al. (2024). Pristine and engineered biochar as Na-ion batteries anode material: a comprehensive overview. Renew. Sustain. Energy Rev. 194, 114304. doi:10.1016/j.rser.2024.114304
Bi, Z., Kong, Q., Cao, Y., Sun, G., Su, F., Wei, X., et al. (2019). Biomass-derived porous carbon materials with different dimensions for supercapacitor electrodes: a review. J. Mater Chem. A Mater 7, 16028–16045. doi:10.1039/c9ta04436a
Bridgwater, A. V. (2007). The production of biofuels and renewable chemicals by fast pyrolysis of biomass. Int. J. Glob. Energy Issues 27, 160–203. doi:10.1504/IJGEI.2007.013654
Chakraborty, M. R., Dawn, S., Saha, P. K., Basu, J. B., and Ustun, T. S. (2022). A comparative review on energy storage systems and their application in deregulated systems. Batteries 8, 124. doi:10.3390/batteries8090124
Chen, Z., Mo, F., Wang, T., Yang, Q., Huang, Z., Wang, D., et al. (2021a). Zinc/selenium conversion battery: a system highly compatible with both organic and aqueous electrolytes. Energy Environ. Sci. 14, 2441–2450. doi:10.1039/d0ee02999h
Chen, Z., Zhao, S., Zhao, H., Zou, Y., Yu, C., and Zhong, W. (2021b). Nitrogen-doped interpenetrating porous carbon/graphene networks for supercapacitor applications. Chem. Eng. J. 409, 127891. doi:10.1016/J.CEJ.2020.127891
Cheng, B. H., Zeng, R. J., and Jiang, H. (2017). Recent developments of post-modification of biochar for electrochemical energy storage. Bioresour. Technol. 246, 224–233. doi:10.1016/j.biortech.2017.07.060
Cheng, F., and Li, X. (2018). Preparation and application of biochar-based catalysts for biofuel production. Catalysts 8, 346. doi:10.3390/catal8090346
Chu, M., Tian, W., Zhao, J., Zou, M., Lu, Z., Zhang, D., et al. (2022). A comprehensive review of capacitive deionization technology with biochar-based electrodes: biochar-based electrode preparation, deionization mechanism and applications. Chemosphere 307, 136024. doi:10.1016/J.CHEMOSPHERE.2022.136024
Cuong, D. V., Matsagar, B. M., Lee, M., Hossain, Md. S. A., Yamauchi, Y., Vithanage, M., et al. (2021). A critical review on biochar-based engineered hierarchical porous carbon for capacitive charge storage. Renew. Sustain. Energy Rev. 145, 111029. doi:10.1016/j.rser.2021.111029
Dai, K., Wang, X., Yin, Y., Hao, C., and You, Z. (2016). Voltage fluctuation in a supercapacitor during a high-g impact. Sci. Rep. 6, 38794. doi:10.1038/srep38794
Devi, P., and Dalai, A. K. (2023). Occurrence, distribution, and toxicity assessment of polycyclic aromatic hydrocarbons in biochar, biocrude, and biogas obtained from pyrolysis of agricultural residues. Bioresour. Technol. 384, 129293. doi:10.1016/j.biortech.2023.129293
Devi, P., and Saroha, A. K. (2014). Risk analysis of pyrolyzed biochar made from paper mill effluent treatment plant sludge for bioavailability and eco-toxicity of heavy metals. Bioresour. Technol. 162, 308–315. doi:10.1016/J.BIORTECH.2014.03.093
Ding, J., Wang, H., Li, Z., Cui, K., Karpuzov, D., Tan, X., et al. (2015). Peanut shell hybrid sodium ion capacitor with extreme energy–power rivals lithium ion capacitors. Energy Environ. Sci. 8, 941–955. doi:10.1039/C4EE02986K
Do, V., Prabhu, P., Jose, V., Yoshida, T., Zhou, Y., Miwa, H., et al. (2023). Pd–PdO nanodomains on amorphous Ru metallene oxide for high-performance multifunctional electrocatalysis. Adv. Mater. 35, e2208860. doi:10.1002/adma.202208860
Energy Institute Statistical Review of World Energy (2023). Energy consumption by source. Available at: https://www.energyinst.org/statistical-review/.
Fisher, T., Hajaligol, M., Waymack, B., and Kellogg, D. (2002). Pyrolysis behavior and kinetics of biomass derived materials. J. Anal. Appl. Pyrolysis 62, 331–349. doi:10.1016/S0165-2370(01)00129-2
Fu, F., Yang, D., Fan, Y., Qiu, X., Huang, J., Li, Z., et al. (2022). Nitrogen-rich accordion-like lignin porous carbon via confined self-assembly template and in-situ mild activation strategy for high-performance supercapacitors. J. Colloid Interface Sci. 628, 90–99. doi:10.1016/j.jcis.2022.07.070
Fu, F., Yang, D., Zhang, W., Wang, H., and Qiu, X. (2020). Green self-assembly synthesis of porous lignin-derived carbon quasi-nanosheets for high-performance supercapacitors. Chem. Eng. J. 392, 123721. doi:10.1016/j.cej.2019.123721
Guo, R., Guo, N., Luo, W., Xu, M., Zhou, D., Ma, R., et al. (2021). A dual-activation strategy to tailor the hierarchical porous structure of biomass-derived carbon for ultrahigh rate supercapacitor. Int. J. Energy Res. 45, 9284–9294. doi:10.1002/er.6458
Gupta, V., Sharma, M., Pachauri, R. K., and Dinesh Babu, K. N. (2019). Comprehensive review on effect of dust on solar photovoltaic system and mitigation techniques. Sol. Energy 191, 596–622. doi:10.1016/J.SOLENER.2019.08.079
Hall, P. J., Mirzaeian, M., Fletcher, S. I., Sillars, F. B., Rennie, A. J. R., Shitta-Bey, G. O., et al. (2010). Energy storage in electrochemical capacitors: designing functional materials to improve performance. Energy Environ. Sci. 3, 1238–1251. doi:10.1039/C0EE00004C
Homagain, K., Shahi, C., Luckai, N., and Sharma, M. (2016). Life cycle cost and economic assessment of biochar-based bioenergy production and biochar land application in Northwestern Ontario, Canada. For Ecosyst 3, 21. doi:10.1186/s40663-016-0081-8
Huang, S., Qiu, X. Q., Wang, C. W., Zhong, L., Zhang, Z. H., Yang, S. S., et al. (2023). Biomass-derived carbon anodes for sodium-ion batteries. Xinxing Tan. Cailiao/New Carbon Mater. 38, 40–66. doi:10.1016/S1872-5805(23)60718-8
Hunt, J. D., Nascimento, A., Zakeri, B., Jurasz, J., Dąbek, P. B., Barbosa, P. S. F., et al. (2022). Lift Energy Storage Technology: a solution for decentralized urban energy storage. Energy 254, 124102. doi:10.1016/J.ENERGY.2022.124102
Husain, Z., Shakeelur Raheman, A. R., Ansari, K. B., Pandit, A. B., Khan, M. S., Qyyum, M. A., et al. (2022). Nano-sized mesoporous biochar derived from biomass pyrolysis as electrochemical energy storage supercapacitor. Mater Sci. Energy Technol. 5, 99–109. doi:10.1016/j.mset.2021.12.003
Jerry Antal, M., Mochidzuki, K., and S. Paredes, L. (2003). Flash carbonization of biomass. Industrial & Eng. Chem. Res. 42, 3690–3699. doi:10.1021/ie0301839
Jiang, Y., and Liu, J. (2019). Definitions of pseudocapacitive materials: a brief review. ENERGY & Environ. Mater. 2, 30–37. doi:10.1002/eem2.12028
Jiménez-Cordero, D., Heras, F., Gilarranz, M. A., and Raymundo-Piñero, E. (2014). Grape seed carbons for studying the influence of texture on supercapacitor behaviour in aqueous electrolytes. Carbon N. Y. 71, 127–138. doi:10.1016/J.CARBON.2014.01.021
Kan, T., Strezov, V., and Evans, T. J. (2016). Lignocellulosic biomass pyrolysis: a review of product properties and effects of pyrolysis parameters. Renew. Sustain. Energy Rev. 57, 1126–1140. doi:10.1016/J.RSER.2015.12.185
Kane, S., Storer, A., Xu, W., Ryan, C., and Stadie, N. P. (2022). Biochar as a renewable substitute for carbon black in lithium-ion battery electrodes. ACS Sustain Chem. Eng. 10, 12226–12233. doi:10.1021/acssuschemeng.2c02974
Klöpffer, W., and Birgit, G. (2014). “Life cycle inventory analysis,” in Life cycle assessment (LCA) (John Wiley & Sons, Ltd), 63–180. doi:10.1002/9783527655625.ch3
Kong, S. H., Loh, S. K., Bachmann, R. T., Rahim, S. A., and Salimon, J. (2014). Biochar from oil palm biomass: a review of its potential and challenges. Renew. Sustain. Energy Rev. 39, 729–739. doi:10.1016/J.RSER.2014.07.107
Kumar, A., Bhattacharya, T., Akram, H. W., Roy, A., Chakraborty, S., and Vithanage, M. (2023). Multifaceted applications of biochar in environmental management: a bibliometric profile. Biochar 5, 1–40. doi:10.1007/s42773-023-00207-z
Lefebvre, D., Williams, A., Meersmans, J., Kirk, G. J. D., Sohi, S., Goglio, P., et al. (2020). Modelling the potential for soil carbon sequestration using biochar from sugarcane residues in Brazil. Sci. Rep. 10, 19479. doi:10.1038/s41598-020-76470-y
Lei, W., Yang, B., Sun, Y., Xiao, L., Tang, D., Chen, K., et al. (2021). Self-sacrificial template synthesis of heteroatom doped porous biochar for enhanced electrochemical energy storage. J. Power Sources 488, 229455. doi:10.1016/J.JPOWSOUR.2021.229455
Li, C., Pan, X., Chen, S., Tao, H., Yang, D., Qiu, X., et al. (2024). Green synthesis of oxygen-enriched tobacco stem-derived porous carbon via pre-oxidation and self-activation for high-performance supercapacitors. Chem. Eng. Sci. 298, 120379. doi:10.1016/j.ces.2024.120379
Li, J., Jiang, Q., Wei, L., Zhong, L., and Wang, X. (2020). Simple and scalable synthesis of hierarchical porous carbon derived from cornstalk without pith for high capacitance and energy density. J. Mater Chem. A Mater 8, 1469–1479. doi:10.1039/c9ta07864a
Li, W., Zhang, Y., Das, L., Wang, Y., Li, M., Wanninayake, N., et al. (2018). Linking lignin source with structural and electrochemical properties of lignin-derived carbon materials. RSC Adv. 8, 38721–38732. doi:10.1039/c8ra08539k
Li, X., Zhang, J., Liu, B., and Su, Z. (2021). A critical review on the application and recent developments of post-modified biochar in supercapacitors. J. Clean. Prod. 310, 127428. doi:10.1016/j.jclepro.2021.127428
Liu, W. J., Jiang, H., and Yu, H. Q. (2019). Emerging applications of biochar-based materials for energy storage and conversion. Energy Environ. Sci. 12, 1751–1779. doi:10.1039/c9ee00206e
Lu, Q., Zhou, S., Li, B., Wei, H., Zhang, D., Hu, J., et al. (2020). Mesopore-rich carbon flakes derived from lotus leaves and it’s ultrahigh performance for supercapacitors. Electrochim Acta 333, 135481. doi:10.1016/J.ELECTACTA.2019.135481
Mehdi, R., Hussain Khoja, A., Naqvi, S. R., Gao, N., Aishah, N., and Amin, S. (2022). A review on production and surface modifications of biochar materials via biomass pyrolysis process for supercapacitor applications. Catalysts 12, 798. doi:10.3390/catal12070798
Mehrotra, S., Gupta, M., Dujearic-Stephane, K., Kumar, A., Singh, P., Bharti, B., et al. (2023). Functional biochar derived from Desmostachya bipinnata for the application in energy storage/conversion devices. Biomass Convers. Biorefin 14, 17263–17275. doi:10.1007/s13399-023-03991-7
Minakshi, M., Mujeeb, A., Whale, J., Evans, R., Aughterson, R., Shinde, P. A., et al. (2024a). Synthesis of porous carbon honeycomb structures derived from hemp for hybrid supercapacitors with improved electrochemistry. Chempluschem, e202400408. doi:10.1002/cplu.202400408
Minakshi, M., Samayamanthry, A., Whale, J., Aughterson, R., Shinde, P. A., Ariga, K., et al. (2024b). Phosphorous – containing activated carbon derived from natural honeydew peel powers aqueous supercapacitors. Chem. Asian J. 19, e202400622. doi:10.1002/asia.202400622
Molino, A., Larocca, V., Chianese, S., and Musmarra, D. (2018). Biofuels production by biomass gasification: a review. Energies (Basel) 11, 811. doi:10.3390/en11040811
Naji, S. Z., and Tye, C. T. (2022). A review of the synthesis of activated carbon for biodiesel production: precursor, preparation, and modification. Energy Convers. Manag. X 13, 100152. doi:10.1016/j.ecmx.2021.100152
Naseem, K., Tahir, A., Khan, A. S., Qin, F., Usman, M., Karamat, S., et al. (2024). Unlocking the potential of magnetic bio-char as a low-cost cathode material for the supercapacitor application. Mater Today Commun. 39, 108764. doi:10.1016/j.mtcomm.2024.108764
Norouzi, O., Salimi, P., Di Maria, F., Pourhosseini, S. E. M., and Safari, F. (2019). “Synthesis and design of engineered biochars as electrode materials in energy storage systems. Prod. Mater. Sustain. Biomass Resour. 233–265. doi:10.1007/978-981-13-3768-0_8
Panwar, N. L., and Pawar, A. (2022). Influence of activation conditions on the physicochemical properties of activated biochar: a review. Biomass Convers. Biorefin 12, 925–947. doi:10.1007/s13399-020-00870-3
Parsimehr, H., Ehsani, A., and Payam, S. A. (2023). Electrochemical energy storage electrodes from rice biochar. Biomass Convers. Biorefin 13, 12413–12429. doi:10.1007/s13399-021-02089-2
Poulose, A. M., Elnour, A. Y., Anis, A., Shaikh, H., Al-Zahrani, S. M., George, J., et al. (2018). Date palm biochar-polymer composites: an investigation of electrical, mechanical, thermal and rheological characteristics. Sci. Total Environ. 619 (620), 311–318. doi:10.1016/J.SCITOTENV.2017.11.076
Qu, J., Wang, Y., Tian, X., Jiang, Z., Deng, F., Tao, Y., et al. (2021). KOH-activated porous biochar with high specific surface area for adsorptive removal of chromium (VI) and naphthalene from water: affecting factors, mechanisms and reusability exploration. J. Hazard Mater 401, 123292. doi:10.1016/j.jhazmat.2020.123292
Rahman, A., Farrok, O., and Haque, M. M. (2022). Environmental impact of renewable energy source based electrical power plants: solar, wind, hydroelectric, biomass, geothermal, tidal, ocean, and osmotic. Renew. Sustain. Energy Rev. 161, 112279. doi:10.1016/J.RSER.2022.112279
Rashid Khan, H., and Latif Ahmad, A. (2024). Supercapacitors: overcoming current limitations and charting the course for next-generation energy storage. J. Industrial Eng. Chem. doi:10.1016/j.jiec.2024.07.014
Rathnayake, D., Ehidiamhen, P. O., Egene, C. E., Stevens, C. V., Meers, E., Mašek, O., et al. (2021). Investigation of biomass and agricultural plastic co-pyrolysis: effect on biochar yield and properties. J. Anal. Appl. Pyrolysis 155, 105029. doi:10.1016/J.JAAP.2021.105029
Rawat, S., Boobalan, T., Sathish, M., Hotha, S., and Thallada, B. (2023). Utilization of CO2 activated litchi seed biochar for the fabrication of supercapacitor electrodes. Biomass Bioenergy 171, 106747. doi:10.1016/j.biombioe.2023.106747
Rawat, S., Mishra, R. K., and Bhaskar, T. (2022). Biomass derived functional carbon materials for supercapacitor applications. Chemosphere 286, 131961. doi:10.1016/j.chemosphere.2021.131961
Rodriguez-Narvaez, O. M., Peralta-Hernandez, J. M., Goonetilleke, A., and Bandala, E. R. (2019). Biochar-supported nanomaterials for environmental applications. J. Industrial Eng. Chem. 78, 21–33. doi:10.1016/j.jiec.2019.06.008
Rui, B., Yang, M., Zhang, L., Jia, Y., Shi, Y., Histed, R., et al. (2020). Reduced graphene oxide-modified biochar electrodes via electrophoretic deposition with high rate capability for supercapacitors. J. Appl. Electrochem 50, 407–420. doi:10.1007/s10800-020-01397-1
Sajdak, M., Muzyka, R., Gałko, G., Ksepko, E., Zajemska, M., Sobek, S., et al. (2022). Actual trends in the usability of biochar as a high-value product of biomass obtained through pyrolysis. Energies (Basel) 16, 355. doi:10.3390/en16010355
Sakhiya, A. K., Anand, A., and Kaushal, P. (2020). Production, activation, and applications of biochar in recent times. Biochar 2, 253–285. doi:10.1007/s42773-020-00047-1
Selvam, S. M., and Paramasivan, B. (2022). Microwave assisted carbonization and activation of biochar for energy-environment nexus: a review. Chemosphere 286, 131631. doi:10.1016/j.chemosphere.2021.131631
Senthil, C., Vediappan, K., Nanthagopal, M., Seop Kang, H., Santhoshkumar, P., Gnanamuthu, R., et al. (2019). Thermochemical conversion of eggshell as biological waste and its application as a functional material for lithium-ion batteries. Chem. Eng. J. 372, 765–773. doi:10.1016/j.cej.2019.04.171
Su, C., Ru, Q., Shi, Z., and Zhao, L. (2019). The lithium storage performance of biochar-loaded metal selenide composite material. Available at: https://api.semanticscholar.org/CorpusID:214018923.
Sudhan, N., Subramani, K., Karnan, M., Ilayaraja, N., and Sathish, M. (2016). Biomass-derived activated porous carbon from rice straw for a high-energy symmetric supercapacitor in aqueous and non-aqueous electrolytes. Energy & Fuels 31, 977–985. doi:10.1021/acs.energyfuels.6b01829
Sutar, S., Otari, S., and Jadhav, J. (2022). Biochar based photocatalyst for degradation of organic aqueous waste: a review. Chemosphere 287, 132200. doi:10.1016/j.chemosphere.2021.132200
Thomas, B., George, G., Landström, A., Concina, I., Geng, S., Vomiero, A., et al. (2021). Electrochemical properties of biobased carbon aerogels decorated with graphene dots synthesized from biochar. ACS Appl. Electron Mater 3, 4699–4710. doi:10.1021/acsaelm.1c00487
Tomczyk, A., Sokołowska, Z., and Boguta, P. (2020). Biochar physicochemical properties: pyrolysis temperature and feedstock kind effects. Rev. Environ. Sci. Biotechnol. 19, 191–215. doi:10.1007/s11157-020-09523-3
Trahey, L., Brushett, F. R., Balsara, N. P., Ceder, G., Cheng, L., Chiang, Y. M., et al. (2020). Energy storage emerging: a perspective from the joint center for energy storage research. Proc. Natl. Acad. Sci. U. S. A. 117, 12550–12557. doi:10.1073/pnas.1821672117
Usha Rani, M., Nanaji, K., Rao, T. N., and Deshpande, A. S. (2020). Corn husk derived activated carbon with enhanced electrochemical performance for high-voltage supercapacitors. J. Power Sources 471, 228387. doi:10.1016/j.jpowsour.2020.228387
Vamvuka, D. (2011). Bio-oil, solid and gaseous biofuels from biomass pyrolysis processes-An overview. Int. J. Energy Res. 35, 835–862. doi:10.1002/er.1804
Wan, C., Jiao, Y., and Li, J. (2016). Core–shell composite of wood-derived biochar supported MnO2 nanosheets for supercapacitor applications. RSC Adv. 6, 64811–64817. doi:10.1039/C6RA12043A
Wang, C., Xiong, Y., Wang, H., Jin, C., and Sun, Q. (2017). Naturally three-dimensional laminated porous carbon network structured short nano-chains bridging nanospheres for energy storage. J. Mater. Chem. A 5, 15759–15770. doi:10.1039/C7TA04178K
Wang, C., Yang, D., Zhang, W., Qin, Y., Huang, S., Liu, W., et al. (2023a). Explosion strategy engineering oxygen-functionalized groups and enlarged interlayer spacing of the carbon anode for enhanced lithium storage. ACS Appl. Mater Interfaces 15, 4371–4384. doi:10.1021/acsami.2c21638
Wang, C., Yang, D., Zhang, W., Qin, Y., Qiu, X., and Li, Z. (2024). Engineering of edge nitrogen dopant in carbon nanosheet framework for fast and stable potassium-ion storage. Carbon Res. 3, 20. doi:10.1007/s44246-024-00101-8
Wang, H., Yu, W., Shi, J., Mao, N., Chen, S., and Liu, W. (2016). Biomass derived hierarchical porous carbons as high-performance anodes for sodium-ion batteries. Electrochim Acta 188, 103–110. doi:10.1016/J.ELECTACTA.2015.12.002
Wang, L., Deng, J., Yang, X., Hou, R., and Hou, D. (2023b). Role of biochar toward carbon neutrality. Carbon Res. 2, 2. doi:10.1007/s44246-023-00035-7
Wang, Q., Song, H., Pan, S., Dong, N., Wang, X., and Sun, S. (2020). Initial pyrolysis mechanism and product formation of cellulose: an Experimental and Density functional theory(DFT) study. Sci. Rep. 10, 3626. doi:10.1038/s41598-020-60095-2
White, J. E., Catallo, W. J., and Legendre, B. L. (2011). Biomass pyrolysis kinetics: a comparative critical review with relevant agricultural residue case studies. J. Anal. Appl. Pyrolysis 91, 1–33. doi:10.1016/J.JAAP.2011.01.004
Wickramaarachchi, K., and Minakshi, M. (2022). Status on electrodeposited manganese dioxide and biowaste carbon for hybrid capacitors: the case of high-quality oxide composites, mechanisms, and prospects. J. Energy Storage 56, 106099. doi:10.1016/j.est.2022.106099
Wiedner, K., Rumpel, C., Steiner, C., Pozzi, A., Maas, R., and Glaser, B. (2013). Chemical evaluation of chars produced by thermochemical conversion (gasification, pyrolysis and hydrothermal carbonization) of agro-industrial biomass on a commercial scale. Biomass Bioenergy 59, 264–278. doi:10.1016/J.BIOMBIOE.2013.08.026
World Energy Outlook (2022). World Energy Outlook. Available at: www.iea.org/t&c/.
Xiang, L., Liu, S., Ye, S., Yang, H., Song, B., Qin, F., et al. (2021). Potential hazards of biochar: the negative environmental impacts of biochar applications. J. Hazard Mater 420, 126611. doi:10.1016/J.JHAZMAT.2021.126611
Xing, X., Farhadi, B., Wang, L., Wang, K., Zhu, Y., Wang, H., et al. (2024). Flexible aqueous supercapacitors for long cycle-life using electrode with multiple active C═S sites. Small 20, e2305692. doi:10.1002/smll.202305692
Xu, Z., He, M., Xu, X., Cao, X., and Tsang, D. C. W. (2021). Impacts of different activation processes on the carbon stability of biochar for oxidation resistance. Bioresour. Technol. 338, 125555. doi:10.1016/j.biortech.2021.125555
Yaashikaa, P. R., Kumar, P. S., Varjani, S., and Saravanan, A. (2020). A critical review on the biochar production techniques, characterization, stability and applications for circular bioeconomy. Biotechnol. Rep. 28, e00570. doi:10.1016/J.BTRE.2020.E00570
Yang, J., Yu, H., Zhen, F., Li, H., Yang, J., Zhang, L., et al. (2024). An integrated electrode material based on corn straw cellulose biochar with three-dimensional network porous structure for boosting electrochemical performance of lithium batteries. Int. J. Biol. Macromol. 268, 131569. doi:10.1016/j.ijbiomac.2024.131569
Yang, S., Wang, S., Liu, X., and Li, L. (2019). Biomass derived interconnected hierarchical micro-meso-macro- porous carbon with ultrahigh capacitance for supercapacitors. Carbon N. Y. 147, 540–549. doi:10.1016/J.CARBON.2019.03.023
Zaman, C. Z., Pal, K., Yehye, W. A., Sagadevan, S., Shah, S. T., Adebisi, G. A., et al. (2017). Pyrolysis: a sustainable way to generate energy from waste. in Pyrolysis, (InTech). doi:10.5772/intechopen.69036
Zhang, J., Gu, M., and Chen, X. (2023). Supercapacitors for renewable energy applications: a review. Micro Nano Eng. 21, 100229. doi:10.1016/j.mne.2023.100229
Zhang, K., Liu, M., Zhang, T., Min, X., Wang, Z., Chai, L., et al. (2019). High-performance supercapacitor energy storage using a carbon material derived from lignin by bacterial activation before carbonization. J. Mater Chem. A Mater 7, 26838–26848. doi:10.1039/c9ta04369a
Zhang, M., Zhang, J., Ran, S., Sun, W., and Zhu, Z. (2022). Biomass-Derived sustainable carbon materials in energy conversion and storage applications: status and opportunities. A mini review. Electrochem Commun. 138, 107283. doi:10.1016/J.ELECOM.2022.107283
Zhao, J., Gong, J., Zhou, C., Miao, C., Hu, R., Zhu, K., et al. (2020). Utilizing human hair for solid-state flexible fiber-based asymmetric supercapacitors. Appl. Surf. Sci. 508, 145260. doi:10.1016/J.APSUSC.2020.145260
Keywords: biomass, engineered biochar, doping, electrode, energy storage, sustainable
Citation: Prabakar P, Mustafa Mert K, Muruganandam L and Sivagami K (2024) A comprehensive review on biochar for electrochemical energy storage applications: an emerging sustainable technology. Front. Energy Res. 12:1448520. doi: 10.3389/fenrg.2024.1448520
Received: 13 June 2024; Accepted: 23 October 2024;
Published: 01 November 2024.
Edited by:
Manickam Minakshi, Flinders University, AustraliaReviewed by:
Caiwei Wang, South China University of Technology, ChinaCopyright © 2024 Prabakar, Mustafa Mert, Muruganandam and Sivagami. This is an open-access article distributed under the terms of the Creative Commons Attribution License (CC BY). The use, distribution or reproduction in other forums is permitted, provided the original author(s) and the copyright owner(s) are credited and that the original publication in this journal is cited, in accordance with accepted academic practice. No use, distribution or reproduction is permitted which does not comply with these terms.
*Correspondence: Krishnasamy Sivagami, c2l2YWdhbWkua3Jpc2huYUBnbWFpbC5jb20=, c2l2YWdhbWkua0B2aXQuYWMuaW4=
Disclaimer: All claims expressed in this article are solely those of the authors and do not necessarily represent those of their affiliated organizations, or those of the publisher, the editors and the reviewers. Any product that may be evaluated in this article or claim that may be made by its manufacturer is not guaranteed or endorsed by the publisher.
Research integrity at Frontiers
Learn more about the work of our research integrity team to safeguard the quality of each article we publish.