- Materials Electrochemistry Laboratory, Department of Chemistry, SRM Institute of Science and Technology, Chengalpattu, India
The production of hydrogen (H2) and multi-carbon fuels through water electrolysis (oxygen evolution reaction (OER)/hydrogen evolution reaction (HER)) and water–CO2 co-electrolysis (OER/CO2 reduction reaction (CO2RR)), respectively, is supposed to be the emergent energy carrier. These electrochemical processes are essential chemical conversion pathways that initiate the changes toward production of renewable energy. This review summarizes the systematic design of earth-abundant transition metal-based nanomaterials and their electrocatalytic activities toward electrochemical energy conversion reactions such as OER, HER, and CO2RR. The primary focus is on fabricating highly effective, low-cost, and advanced transition metal-based nanostructures for both the OER/HER and OER/CO2RR systems. Developing synthetic strategies for surface morphology-controlled nanostructured electrocatalysts, engineering the electrode surface, enhancing the electrocatalytic activity, understanding the relationship between intrinsic catalytic activity and preparation approaches or precursor choices, and exploring the reaction mechanism are focused on. Furthermore, the current challenges, figure-of-merit, and prospects of transition metal-based nanomaterials and their electrocatalytic activities toward water electrolysis and water–CO2 co-electrolysis are described. This study may open new opportunities to develop shape-controlled and high-performance electrocatalysts for electrochemical energy conversion and storage reactions.
1 Introduction
With the tremendous population explosion and globalization, as well as the corresponding rise in global energy supply and consumption, the discovery of methodologies for devising sustainable power conversion systems remains a major global concern (Chen C. et al., 2024; Maduraiveeran et al., 2019; Yuan et al., 2024; Zhang M. et al., 2024). The global energy crisis and the associated environmental pollution have prompted researchers to look for renewable sources of energy that can be used to replace fossil fuels (Arias-Hernandez et al., 2024; Bonod et al., 2023; Bordet and Leitner, 2023). Owing to the energy scarcity, environmental concerns, and the excessive use of fossil fuels, fresh and renewable energy sources have emerged as a pressing need (Fang et al., 2024; Guo et al., 2024; Hatakeyama-Sato and Oyaizu, 2023). As a result, the search for sustainable alternatives such as energy conversion and energy storage technologies is vital. Electrochemical methods are deliberated as promising strategies for the generation of clean energy by using the conversion of small molecules, including water (H2O), hydrazine (N2H4), carbon dioxide (CO2), and nitrogen (N2), into valued products hydrogen (H2), ammonia (NH3), hydrocarbons (CH), and fuels (Arivazhagan et al., 2020; Fan et al., 2024; Fang et al., 2024; Geng et al., 2024; Shankar et al., 2020).
The pursuit of sustainable energy sources drives the development of progressive nanostructured catalysts for a variety of electrocatalytic processes, such as oxygen evolution reaction (OER), hydrogen evolution reaction (HER), and CO2 reduction reaction (CO2RR) (Figure 1) (Wang J. et al., 2020). In particular, the slow kinetics and high energy barriers in the OER (anode side) and HER/CO2RR (cathode side) make it difficult to achieve better results and utilize the energy conversion process. When the CO2RR is combined with electrochemical water splitting, the energy conversion technologies do not necessitate an extra hydrogen feedstock. In its place, water could deliver the protons required for the reduction of CO2. The integration of the OER (anode process) and CO2RR (cathode process) is frequently stated as water–CO2 co-electrolysis (Ebbesen et al., 2014; O’Brien et al., 2024; Zhang J. et al., 2023). The fundamental chemical conversion pathways that lead to transformations in renewable energy are these electrochemical processes. In these electrochemical reactions, the catalytic activity, mass activity, discrimination, and robustness of the catalysts play an active part in defining the energy efficacies and the performance of the device/system (Chatenet et al., 2022; Dondapati et al., 2022; Maduraiveeran, 2021; Shankar and Maduraiveeran, 2022; Shankar et al., 2023). Subsequently, the crucial goal of this electrochemical energy conversion technology is to find high-efficiency catalytic materials for several electrochemical reactions (Sun et al., 2020; Wang Y. et al., 2020; Yang et al., 2022).
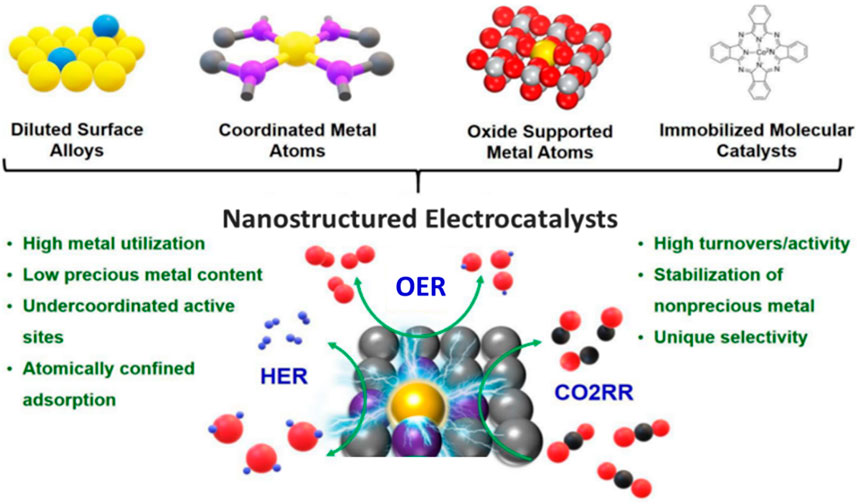
Figure 1. Nanomaterial-based electrocatalysts and their intrinsic characteristics for improved electrocatalytic reactions (Wang J. et al., 2020).
Most of the electrocatalytic reactions extensively depend on the state-of-the-art catalysts of platinum (Pt)-, ruthenium (Ru)-, iridium (Ir)-, palladium (Pd)-, gold (Au)-, and rhodium (Rh)-based metal/metal oxides (Li et al., 2024a; Liu H. et al., 2024; Zhang X. et al., 2024). However, the scarcity and high expenses involved in producing these precious metal-based catalysts often limit the wide-ranging employment on scale-up progress and the commercial feasibility of the associated electrochemical energy technologies (Cai L. et al., 2024; Chang et al., 2024a; Chang et al., 2024b). Thus, the establishment of inexpensive, high earth-abundance, great catalytic activity, and durable electrocatalysts as substitutes for possible renewable technologies is both highly preferred and a grand challenge. Due to the extraordinary catalytic activity, high earth-abundance, great durability, facile fabrication, good conductivities, and ecological sociability, the first-row transition metals including copper (Cu)-, iron (Fe)-, nickel (Ni)-, and cobalt (Co)-based metal oxides and chalcogenide, sulfide, hydroxide, phosphide, and borate-derived electrocatalysts have emerged as substitutes (Figure 2) (Wang et al., 2021) that have raised interest in numerous electrocatalytic reactions, including water splitting, CO2RR, photo-electro catalytic reactions, and metal–air batteries. (Cai M. et al., 2024; Cao et al., 2024; Chai et al., 2024; Marimuthu et al., 2023).
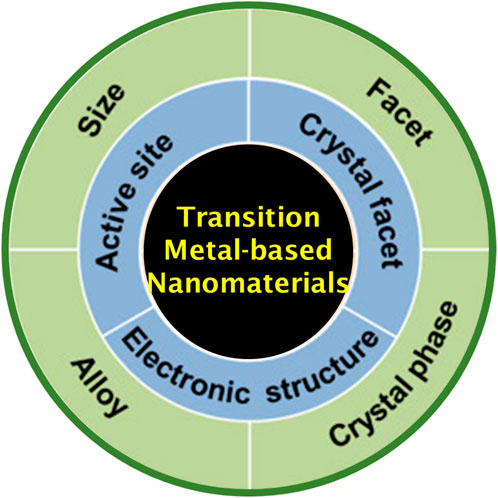
Figure 2. Transition metal-based nanomaterials for electrochemical energy conversion reactions (Wang et al., 2021).
Numerous studies have been conducted based on transition metal nitrides, phosphides, selenides, carbides, and sulfides catalysts for various electrochemical energy conversion reactions (Cai L. et al., 2024; Chang Y. et al., 2024; Chang et al., 2024a; Chen G. et al., 2023; Chen X. et al., 2023; Chen Y. et al., 2023; Chen X. et al., 2024). It has been demonstrated that the covalently synchronized transition metal centers may have good properties for adsorption and robustness in a range of electrocatalytic applications (Abdelghafar et al., 2024; Chen G. et al., 2023; Deng L. et al., 2023; Deng M. et al., 2023). Moreover, the improved turnover rates and mass activity (employment of metal efficacy) have been advantageous for engineering the catalytic performance through the captivating benefit of the atomic- and nano-scale confinement of adsorption intermediates, which increase the divergent electrocatalytic performances since their bulk equivalent stating repetitive active sites (Abdelghafar et al., 2022; Kong D. et al., 2024; Kong X. et al., 2024; Kothandam et al., 2023). Substantial research has been conducted on the progress of synthetic strategy, the size–shape-controlled fabrication of nanostructured transition metal-based electrocatalysts that demonstrate improved electrocatalytic activity owing to their fascinating physical−chemical and electrochemical properties (Lee et al., 2023; Li et al., 2024b; Li D. et al., 2024; Li Z. et al., 2024). The present review summarizes the systematic design of earth-abundant transition metal-based nanomaterials and their electrocatalytic activities toward the electrochemical energy conversion reactions such as the OER, HER, and CO2RR. Fabricating improved transition metal-based nanostructures for energy conversion reactions at low cost and high efficiency is the focus of this review. There is also significant focus on preparation techniques, monitoring of structure and composition, electrode fabrication, catalytic effect, connections between synthesis methods and precursors, intrinsic catalytic activity, and reaction mechanism comprehension and investigation.
2 Water electrolysis
Recently, there have been several studies that focused on the hydrogen energy economy (HEE), which is regarded as the ultimate replacement for fossil fuels, as a result of its high density and water being the sole byproduct (Figure 3) (Rebouillat et al., 2011; Niu and Yang, 2018). Hydrogen is an alternative fuel with a sustainable and environmentally friendly nature that has been presented as both the supreme and perfect fuel for such a futuristic world (Li et al., 2024a; Zhang W. et al., 2023; Zhu et al., 2024). Considering the dual heavy burdens of energy constraint and environmental contamination, establishing sustainable fuel sources has developed into one of the most relevant global issues (Wei et al., 2023; Yan et al., 2021). The production of green hydrogen mostly depends on electrochemical processes such as HER and OER (Figure 4A) (Xu et al., 2019). The hydrogen energy source may also provide emission-free, environmentally beneficial, and alternative/sustainable energy cycling (H2O) (You and Sun, 2018). The hydrogen system needs to perform better and be less expensive, but it is still in the pre-civilization stage. Two energy conversion methods, namely, water splitting and fuel cells, can achieve the environmentally friendly production and consumption of hydrogen (You and Sun, 2018).
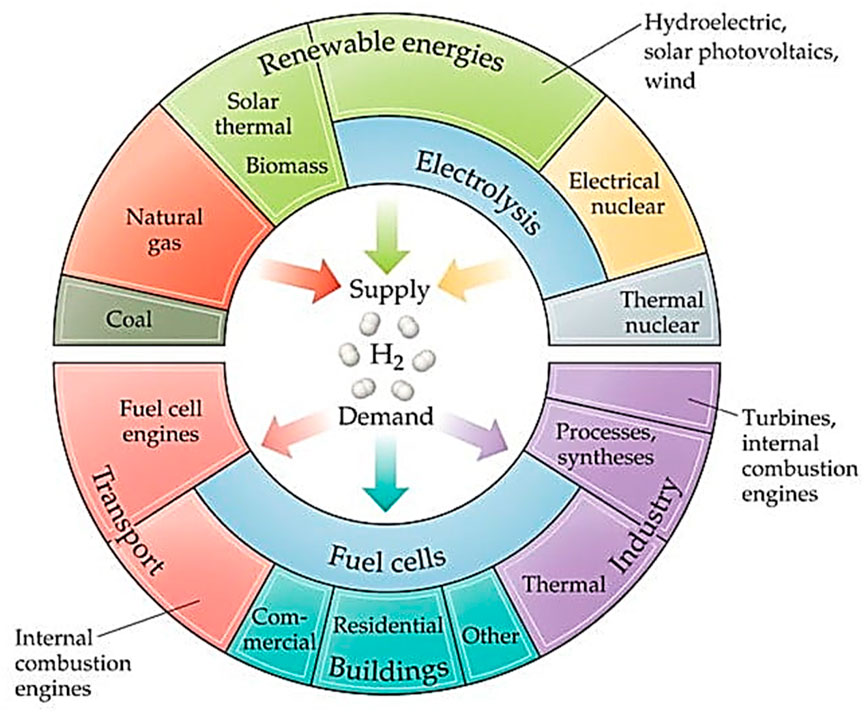
Figure 3. Pictorial illustration of hydrogen economy (Rebouillat et al., 2011).
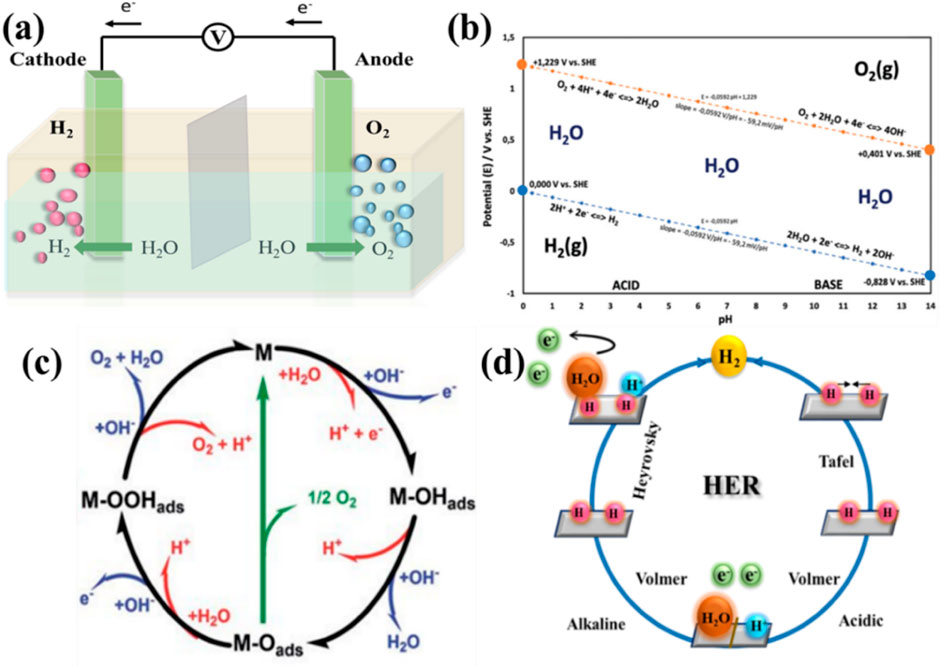
Figure 4. (A) Schematic illustration of water electrolysis (Xu et al., 2019). (B) Possible electrode potential for electrolysis of water with pH at standard conditions (McHugh et al., 2020). Schematic mechanism of the OER (C) and HER (D) under basic and acidic media (Gonçalves et al., 2021).
The electrocatalytic splitting of water offers a much more environment-conscious way to create hydrogen than these methods do (Gao et al., 2019; Gao et al., 2020). A prospective way to generate hydrogen with high purity and a carbon-neutral source for energy storage and recovery, as well as a starting point for commercial catalytic processes, is electrochemical water splitting (Kang et al., 2015; Liu et al., 2020; Pu et al., 2016; Wang et al., 2016). For the electrolysis of water to function as a strategic initiative for storing sustainable power in the energy-dense bonding of H2, both reactions must take place with high efficiency in the presence of affordable catalysts (Anantharaj and Noda, 2020). However, in contrast to the HER, the OER has slower electrode kinetics because of the four-electron transfer process (Butburee et al., 2018). On the other hand, the catalytic performance of oxygen evolution at the anode is crucial for the effectiveness of hydrogen production during the electrolysis of water. According to the pH level at which the electrolysis is performed, such half-equations vary a little (Figure 4B) (McHugh et al., 2020).
Adsorption of OH− on the catalyst (M–OH) and the adsorbed OH− ions converting into oxygen moieties on the catalysts (M–O) are the primary stages. There are two distinct ways to create O2 species from the adsorbed intermediates: either a peroxide (M–OOH) intermediate is created during the third electron transfer and then decays after the fourth electron transfer step to release O2 gas or two neighboring M–O intermediates are directly combined to yield O2 gas under the alkaline electrolyte. Electrocatalytic OER in heterogeneous OER reactions depends on the bonding interactions (M−O) in the intermediates of M−OH, MO, and M–OOH (Gonçalves et al., 2021) (Figure 4C) (Guo et al., 2023; Raveendran et al., 2023; Xu et al., 2023). There are simple processes in the HER (Figure 4D) (Gonçalves et al., 2021). The HER process may be classified into two steps, where the first one, designated Volmer reaction, is proton reduction caused by creating H* on the catalysts under alkaline media. The second step, the Heyrovsky reaction, is the formation of H2. In addition to the Heyrovsky reaction, H2 may be formed via the Tafel reaction. There are two H* in the vicinity combined on the surface of the electrode forming H2. 1) On the surface, a proton first needs to be ingested by capturing an electron. 2) Two alternative mechanisms can be used to progress to the second aspect. When two adsorbed hydrogen atoms interact together, 3) utilizing a Langmuir process, either one of the hydrogen atoms that has been adsorbed combines with a proton that has been dissolved in water by absorbing one electron, as follows the reaction mechanism.
The transition metal-derived nanostructures as emergent substitutes have been used for numerous electrocatalytic reactions due to their extraordinary catalytic activity, high earth-abundance, good conductivity, and ecological sociability (Farhan et al., 2024; He et al., 2024; Hegazy et al., 2024; Yao et al., 2023). High earth-abundance transition metal-derived catalysts are dispersed on carbon (C) or Ni conductive electrode substrates that have been generally stabilized through attachment with doped nitrogen (N) and/or an additional class of hetero-atomic molecules. It has been shown that the covalently synchronized transition metal centers could discover encouraging adsorption possession and durability for various electrocatalytic applications (Chen et al., 2024c; Sadeghi et al., 2023; Zhu et al., 2023). The intriguing benefit of the atomic- and nano-scale confinement of adsorption intermediates has also been utilized to engineer the catalytic performance through improved turnover rates and mass activity (employment of metal efficacy), which has provided an increase in divergent electrocatalytic performances since their bulk equivalent stating repetitive active site (Mao et al., 2023; Sadeghi et al., 2023; Sheng et al., 2023; Xu et al., 2023). Such nanotechnology-based techniques further enable the development of electrocatalysts and assist the production of nanomaterials mostly with improved efficacy, enhanced specificity, and preferred electrochemical performance by manipulating their surface energy, electrochemically active surface area, and physiochemical characteristics at the molecular or atomic level. Extensive dimension- and shape-controlled nanostructured transition metals and metal oxide materials such as Pt−Fe3O4, metal oxides of FeCoNiO, FeOOH/Co/FeOOH, Fe-doped NiSe2, and Fe2C are demonstrated for improved electrochemical properties (Mao et al., 2023; Mekete Meshesha et al., 2023; Reddy et al., 2023; Ren et al., 2023; Sabir et al., 2023; Sadeghi et al., 2023).
Elakkiya et al. developed a durable and efficient bi-functional electrocatalyst using hierarchical flower-like nickel cobalt oxide nanoporous (NiCo2O4-NP) materials for the overall electrocatalytic water splitting (Elakkiya et al., 2019). The NiCo2O4-NP materials are synthesized through a hydrothermal method using nickel nitrate and cobalt nitrate in a 1:3 ratio as the precursors. The NiCo2O4-NP materials displayed a flower-like surface morphology with a pore size of ⁓11.2 nm (Figures 5A,B). Cubic NiO had a flower-like morphology that measured a few microns in length (Figures 5C,D), whereas spinel Co3O4 had a rice-flower-like structure that measured a few nanometers ((Figures 5E,F)). The crystalline nature of NiCo2O4-NP nanostructures can be indexed to the (111), (220), (311), (200), (511), and (440) planes, suggesting a spinel crystalline structure (Farhan et al. 2024). The developed NiCo2O4-NP, NiO, and Co3O4 nanostructures showed polycrystallinity, and the corresponding crystalline planes also agreed well with the XRD results. The high-resolution XPS spectrum of Ni 2p, Co 2p, and O 1s species for the NiCo2O4-NP materials is reported to assess the chemical state of nickel (Ni2+and Ni3+), cobalt (Co3+ and Co2+), and oxygen (M–O bonds and defect spots with little O). It is highly understood that the imperfect O-sites may expedite the electrocatalytic OER activity of the spinel oxide nanomaterials. The electrocatalytic measurements of NiCo2O4-NP materials for the OER and HER were examined in 1.0 M KOH. The LSV curves of the NiCo2O4-NP nanomaterial were compared to those of NiO, Co3O4, commercial IrO2, bare NiF, and commercial Pt/C (Figure 5G). In particular, the NiCo2O4-NP material shows a reduced overpotential of ∼360 mV and increased OER activity at 10 mA cm−2. The NiCo2O4-NP materials produced a low onset potential and a Tafel slope of approximately 1.52 V and 150 mV dec−1, respectively (Figure 5H). It was discovered that the maximal mass activity of the NiCo2O4-NP nanomaterial was approximately 112.3 Ag−1, and its TOF value was 0.103 s−1. The electrocatalytic OER activity of the NiCo2O4-NP materials was investigated further using the EIS, potentiometric, and chronoamperometric techniques (Figures 5I,J). The electrode potentials of ∼−0.32, ∼−0.38, and ∼−0.42 V were delivered by the NiCo2O4-NP nanomaterials for the applied constant current densities of −5.0, −10.0, and −15.0 mA cm-2, respectively, as seen in Figures 5I,J. On the other hand, by providing constant applied potentials of −0.33, −0.38, and −0.43 V, respectively, the NiCo2O4-NP nanomaterials displayed the current densities of ∼−3.3, ∼−9.9, and ∼−17.7. The potential of 1.96 V and 1.70 V was revealed by NiCo2O4||NiCo2O4 and Pt/C||IrO2, indicating that the well-established hierarchical NiCo2O4 nanostructures found on Earth had nearly the same potential as cutting-edge Pt/C catalysts for the alkaline water electrolyzer.
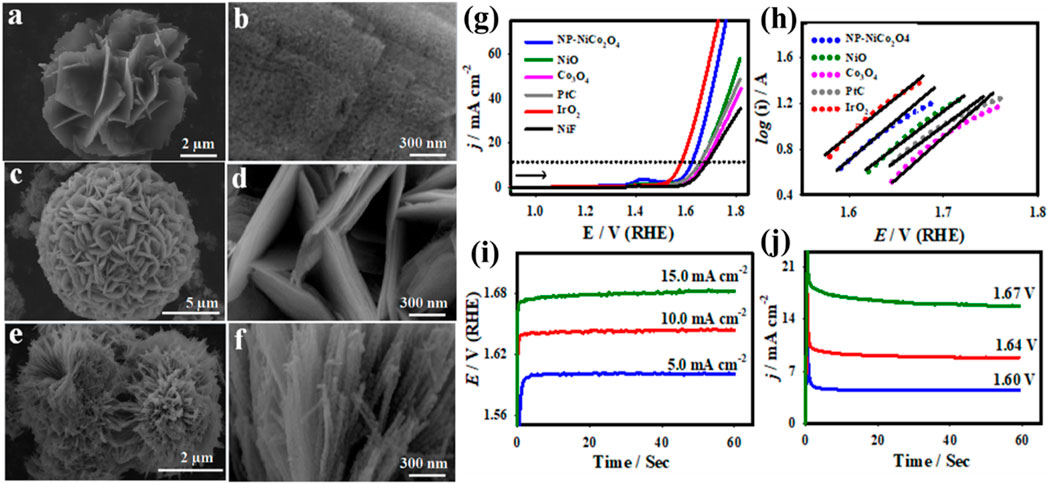
Figure 5. SEM images of NP-NiCo2O4 (A, B), NiO (C, D), and Co3O4 (E, F) nanostructures and their OER catalytic activity (G–J) (Elakkiya et al., 2019).
Santos et al. fabricated the NiMo−NiCu0.06 nanostructures by a simple electrodeposition method that exhibited a low overpotential of ⁓86 mV (@ j of ⁓10 mA cm−2) toward HER (Santos et al., 2020). Interestingly, the single atomic catalyst of cobalt and nitrogen co-doped with graphene was successfully developed by the annealing method, which delivers the overpotential ⁓384 mV (@ j of ⁓20 mA cm−2) in 1.0 M KOH (Zhang Q. et al., 2020). Bose et al. reported the preparation of CoSe2|CoP-DO electrode materials grown on carbon fiber paper using the two-step process (solvothermal and phosphidation). The synthesized CoSe2|CoP-DO showed excellent performance in the OER with a low overpotential (η) of ⁓240 mV (@ current density (j) of ⁓10 mA cm−2) and outstanding stability for ⁓120 h (Bose et al., 2018). A facile preparation of earth-abundant two-dimensional (2D) transition metal oxide (TMO) nanosheets such as cobalt oxide (Co3O4-NS), nickel oxide (NiO-NS), copper oxide (CuO-NS), and iron oxide (Fe3O4-NS) for the OER under alkaline electrolyte was developed by Elakkiya and Maduraiveeran (2020). These TMO nanosheets are synthesized using chemical reduction accompanied by annealing treatment. Because 2D metal oxides have high surface-to-volume ratios and electron confinement, there has been significant interest in developing a generic synthetic technique for them. When comparing 2D metal oxides to graphene and/or metal hydroxides, it is much easier to grasp how the intrinsic layered atomic structures of these compounds form. One possible explanation for the construction of 2D layered nanosheets of metal oxide is that, even before the calcination process, the facets with strong energy in the lateral path may have progressed to a greater degree than the low-energy facets in the vertical direction. By selectively changing some aspects’ energy or initiating the directed attachment of primary blocks, the resulting NH3- molecules can function as a template agent for the formation of 2D nanosheets. From the volcano plot, RuO2 and Co3O4 adsorb oxygen OER intermediates at almost ideal binding energy, while CuO and Fe3O4 nanosheets bind to the intermediates weakly, and NiO binds with them strongly. The created 2D Co3O4 nanosheet was shown to have suitable adsorption energy, indicating its potential as an effective OER catalyst (Figure 6A). The most frequently generated intermediates, as shown in Figure 6B, are M–OH and M–O, which are most likely centered on the OER that generates oxygen. The bonding interactions (M−O) in the intermediates of M−OH, MO, and M–OOH are essential for the electrocatalytic OER. Due to its high BET-specific surface area, 2D morphological architecture, organized thin-sheet-like nanostructures with homogenous mesopores, and enormously abundant active sites, the Co3O4-NS significantly outperformed the other materials investigated in this work in terms of OER activity, as shown by all the results. Alnaser co-workers prepared the laser-induced NiCoCr(V) electrode materials, with their richness of oxidation states, and exhibited high-performance electrochemical water splitting that is comparable to those of commercial electrodes (Ahmad et al., 2024). The NiCoCr(V) electrode demonstrated an overall water splitting potential of 1.61 V@10 mA cm−2, which is very close to 1.56 V of Pt/C||Ir/C electrodes. By constructing laser-induced periodic structures on the surface, femtosecond laser surface structuring has been utilized to expand the electrochemically active surface area and further enhance the oxidation states through customized laser–matter interactions.
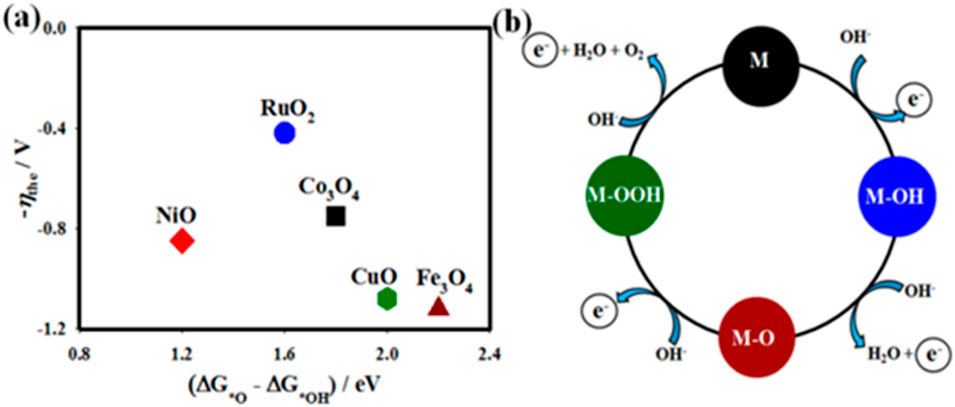
Figure 6. (A) Plot of theoretical overpotential (η the) on adsorption energy of intermediates vs. the investigated materials. (B) OER mechanistic for the fabricated metal oxides (Elakkiya and Maduraiveeran, 2020).
The development of several nanostructured iron sulfide (FeS) materials, including rice grains (RGS), nanoflowers (NFS), and nanoparticles (NPS), has been accomplished by electrodeposition, solvothermal, and chemical techniques, respectively (Elakkiya and Maduraiveeran, 2021a). The systematic investigation of the connection between the morphology-controlled nanostructure FeS production processes (Figures 7A–C) and the electrocatalytic OER activity is examined (Figures 7D–G). As the common S-source for all the preparation techniques used in this work, DMSO was specifically used to generate different nanostructured FeS as the emerging OER electrocatalysts. The solvothermal-supported FeS nanomaterials revealed a flower-like morphology, the chemically assisted production of FeS particles showed a high distribution of sphere-like surface morphology, and the electrochemically produced FeS nanomaterials appeared to have a rice grain-like morphology according to the SEM and TEM images (Figures 7A–C). In comparison with the developed nanostructures in this work, the electrodeposited FeS-RGS|NF electrode demonstrated high OER catalytic activity with low onset potential of ∼1.37 V, low overpotential of ∼0.20 V at 10 mA cm−2, small Tafel slope of ∼54.2 mV dec−1, and high mass activity of ∼5.4 Ag−1, as displayed in Figures 7D–G. The FeS-RGS nanostructures also showed excellent durability and ECASA during 24 h at a constant current density of 17 mA cm−2. The FeS-RGS || PtC shows the stable OER current density that was reached. The real-world capability of FeS-RGS nanostructures was found, as evidenced by the minimal fluctuation in the overpotential of approximately 4 mV. The high mass activity and low overpotential of the as-developed FeS-RGS nanostructures were demonstrated. Thanks to the constructive in situ oxidation of Fe-species, synergistic effects, numerous accessible electrochemical active sites, direct growth of rice grain structures, and constructive in situ oxidation, the nanostructured FeS–RGS electrode exhibited improved OER catalytic activity.
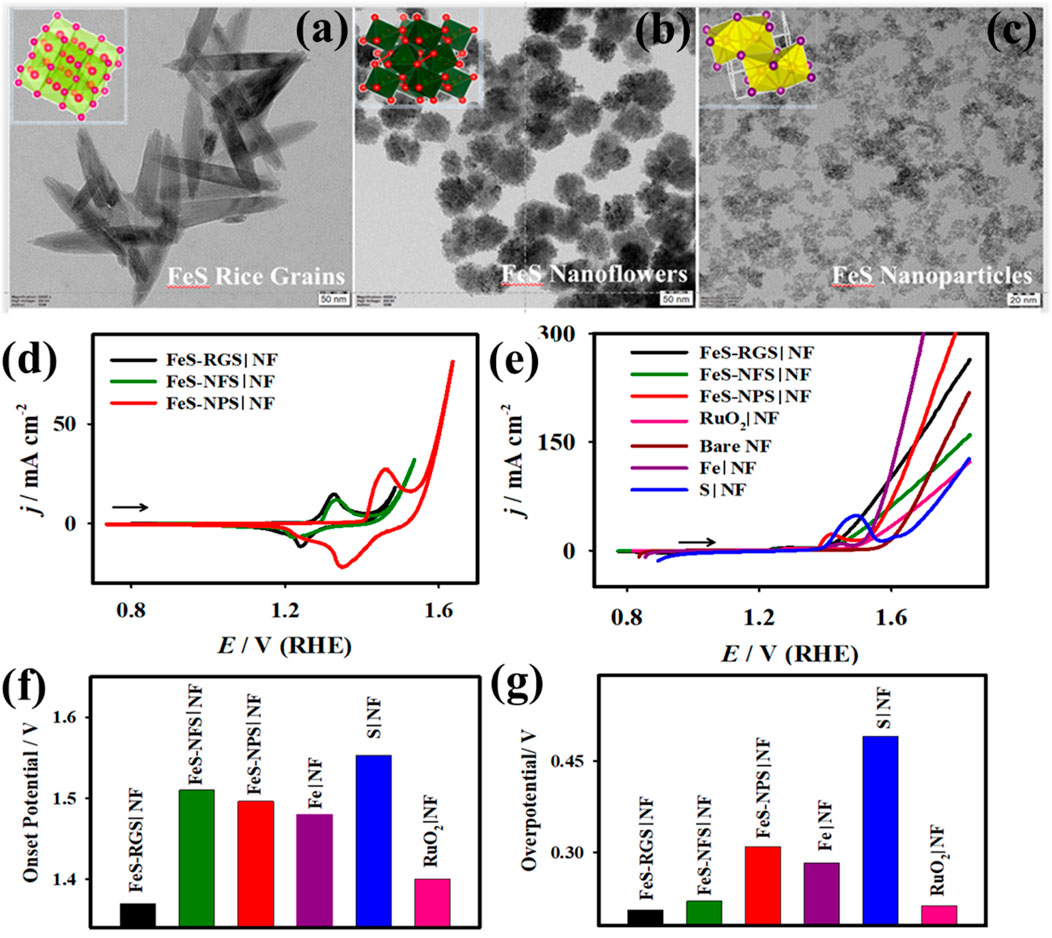
Figure 7. TEM images of the FeS nanomaterials with rice grain- (A), flower- (B), and nanoparticle-like (C) nanostructures. Inset: corresponding crystalline structure and their OER activity. Figure 5. CV curves (D), LSV curves (E), and the plots of onset potential (F) and overpotential (G) vs. the developed FeS nanomaterials with rice grain-, flower-, and nanoparticle-nanostructures (Elakkiya and Maduraiveeran, 2021a).
3 Water–CO2 electrolysis
The electrochemical conversion of CO2 in the atmosphere (CO2) into extremely energy-dense carbon molecules that could be used as energy and feedstocks, such as a leading “carbon-neutral” energy source, is one of the most viable methods (Bhargava et al., 2021). Worldwide, the energy economy and chemical industry rely heavily on fossil fuels, such as carbon dioxide (CO2), which are powered by renewable resources. This puts environmental safety at risk since it increases the atmospheric concentration of CO2 (Dinges et al., 2024; Hua et al., 2024; Jiang et al., 2024; Pei et al., 2021). The electrochemical carbon dioxide reduction reaction (CO2RR) must be optimized for high-performance and low-cost electrocatalysts to produce value-added chemicals and fuels (Liu Y. et al., 2024; Yang et al., 2024; Yu et al., 2024; Zhang Y. et al., 2024).
The application of CO2 in an electrochemical reduction process yields hydrocarbon and alcohol (Timoshenko et al., 2022). Research and enhancement of heterogeneous and homogeneous catalysts for the CO2RR under organic electrolytes and alkaline electrolytes have received much attention in scientific research (Bagemihl et al., 2023; Barecka et al., 2021; Bi et al., 2023). The primary benefits of converting CO2 electro-catalytically to value-added chemicals are as follows: 1) reusing its supporting electrolyte is conceivable, 2) limiting the carbon dioxide emissions, 3) possible modifications to the electrochemical cell, and 4) regulating the mechanism using voltage and temperature. The efficiency and selectivity of the electrochemical reduction of carbon dioxide are primarily influenced by the CO2 concentration in the electrolyte, the form of the electrode and electrolyte, and the applied current or voltage (Song et al., 2019; Ye et al., 2017; Zheng et al., 2017).
When the CO2RR is combined with electrochemical water splitting, the energy conversion technologies do not necessitate an extra hydrogen feedstock (Hua et al., 2024; Jiang et al., 2024; Pimlott et al., 2023). Water might provide the protons required to reduce CO2 in its place. The term “water–CO2 co-electrolysis” is often used to describe the combination of the anode process (OER) and the cathode process (CO2RR) (Figure 8A) (Meng et al., 2019). Several criteria, including the Faradaic efficiency, current density, onset potential, and energy efficiency, related to core electrochemical performance may be systematically determined. Three key phases in the CO2RR mechanism take place at the cathode and electrolyte interaction. The chemical adsorption or the activation of carbon dioxide molecules onto the interface of the catalysts is the initial step. Although it has a greater energy boundary, it is typically a rate-determining step throughout the reduction process of CO2. The C–O bonds are then broken, causing related reaction products to develop as protons and electrons are transferred (Figure 8B) (Kortlever et al., 2015). These mediators are crucial for producing hydrocarbon products. Finally, these products undergo a rearrangement to become products and separate into the electrolyte from the catalytic surface. The anodic process takes place on the anode surface concurrently with the charge carrier of the whole electrochemical reaction. Figure 8C shows the pictorial representation of CO2RR at homogeneous, heterogeneous electrocatalysts, and when immobilized (Varela et al., 2018). A variety of nanomaterials comprising several elements, including nitrogen, carbon, and non-noble metals, extending from distinct restrained complexes to carbon materials doping have been considered as appropriate substitutes.
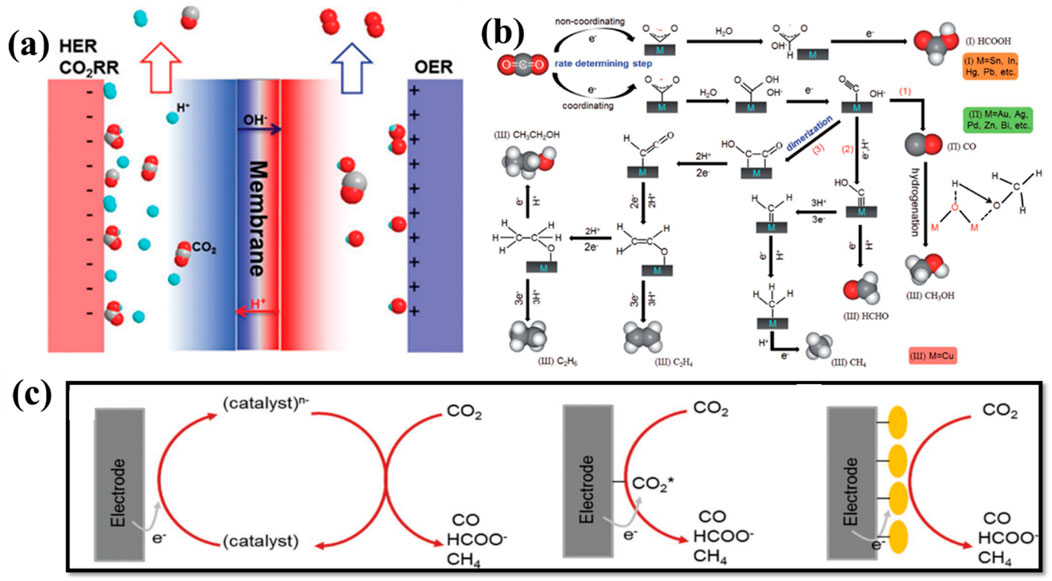
Figure 8. (A) Schematic illustration of water–CO2 electrolysis toward the OER at the anode and syngas production at on the cathode. (B) Mechanism for the electrochemical carbon dioxide reduction reaction on the catalyst surface (Zhang et al., 2018). (C) Pictorial representation of CO2RR homogeneous (left), heterogeneous electrocatalysts (mid), and immobilized catalyst (right) (Varela et al., 2018).
A key tactic in the creation of high-performance electrocatalysts for enhanced CO2RR is the morphology- and dimension-controlled production of nanostructured electrode materials based on transition metals, oxides, and chalcogenides (Zhang Z. et al., 2024; Zhang M. et al., 2024; Zhang et al., 2019). Several research studies have been conducted to create high-efficiency electrode substances with the selective synthesis of compounds with added value, high mass activity, long-term durability, low onset potential, and overpotential (Li J. et al., 2023; Li P. et al., 2023; Lu et al., 2024). Modern noble metal-based catalysts (Au, Pt, Ag, Pd, etc.) have limited commercial application due to their high cost, low selectivity, and less earth abundance (Kim et al., 2020; Ko et al., 2024; Lv et al., 2021). Numerous catalysts, including semiconductors, organic compounds, proteins, ionic liquids, and organometallic complexes, for CO2RR have been studied in the past (Patra and Gopinath, 2023; Pimlott et al., 2024; Roy et al., 2024). Recently, Zhao et al. produced the Cu/ZnO2/Al2O3@ Cu/ZnO/MgO electrocatalysts for the formation of methanol during CO2 hydrogenation using a template-assisted chemical deposition process, followed by an incipient wetness impregnation method (Guo et al., 2019). Huang and colleagues created partially oxidized 5-nm cobalt nanoparticles scattered on single-layer nitrogen-doped graphene (PO-5 nm Co/SL-NG) to manufacture methanol with a maximum faradaic efficiency of 71.4% at the potential of −0.90 V vs. RHE (Huang et al., 2018). Zhang et al. developed the MOF-derived nanostructures of Cu GNC-VL to achieve a high faradaic efficiency of 70.52% at −0.87 V against RHE in the electro-reduction of CO2 to ethanol (Zhang Y. et al., 2020). Recent research demonstrates that copper (Cu) nanoparticles are among the most highly electrically conductive and catalytically active, and they inhibit the HER activity (Mavrokefalos et al., 2020).
Moreover, metallic Cu or Cu-based compounds have been extensively employed for diverse purposes such as photo-thermal, electrical, organic transformation and for electronic devices (Li L. et al., 2022). Researchers are particularly interested in copper chalcogenides because of their fascinating mechanical, electrical, optical, and catalytic properties (Shin et al., 2015; Song et al., 2024; Song et al., 2019). Better electrochemical properties were provided by the CuSe-based electrode materials, which had various oxidation states and good electrical conductivity. Furthermore, the heterostructures including unsaturated Se atoms and their edges might increase the number of the exposed active sites and electrocatalytic activity in CO2RR. To create materials with Cu-derived chalcogenides, several synthetic methods have been established. In addition to having a variety of crystallographic patterns (monoclinic, hexagonal, tetragonal, and cubic), it can exist in a broad range of stoichiometric (Cu3Se2, Cu2Se, Cu7Se4, CuSe2, and Cu5Se4) and nonstoichiometric (Cu2-xSe) compositions (Wang, 2023; Wen et al., 2023; Woldu et al., 2023).
Several techniques have been successfully employed for the preparation of CuSe nanomaterials: microwave, colloidal, electrodeposition, chemical bath, sputtering, hot-injection, sol–gel, spin coating, solid-state reaction, hydrothermal, dip coating, and vapor-based methods. Maduraiveeran et al. established a one-step electrochemical strategy for the fabrication of highly efficient and stable nanocube-like copper selenide nanostructures (CuSe NCBs) for the enhanced electrochemical carbon dioxide reduction reaction (CO2RR), representing a new path for environmental and energy sustainability concerns (Elakkiya and Maduraiveeran, 2021b). A sequence of highly ordered 3D CuSe NCBs gathered as domain nanocube-, branched nanocube-, and dendrite nanocube-like surface morphology on copper microelectrodes is developed (Figure 9). The as-fabricated nanostructures were chosen as CuSe NCs-A, CuSe NCs-B, and CuSe NCs-C for the Cu/Se molar ratios of 1:1; 1:3, and 3:1, respectively. Moreover, the major electrocatalytic CO2RR was examined at the modified hierarchically arranged 3D CuSe NCBs under both aqueous (KHCO3) and organic ([Bmim]PF6/MeCN) electrolytes. In 1.0 M aqueous KHCO3, the CuSe NCs-B-based Cu microelectrodes showed a less negative onset potential of −0.5 V vs. RHE, a high catalytic current density of −120.3 mA cm−2, a low cathodic potential of −0.65 V vs. RHE, and a high mass activity of 41.6 mA g−1 (Figure 10). In [Bmim]PF6 in the MeCN electrolyte, the CuSe NCBs-B based Cu microelectrodes exhibited a less negative onset potential of −1.1 V vs. Ag/AgCl and high catalytic current density of −23.4 mA cm−2. These results indicated that the 3D CuSe-B nanocube electrode possessed many electrochemical active sites, which led to enhanced CO2RR activity. The initial intermediate of absorbed CO can be improved by including appropriate Cu and Se active sites on the catalysts. This allows the intermediate to absorb protons and electrons, becoming absorbed CHO, which is then reduced to methanol. According to all the electrochemical reaction results, CuSe NCs-B/CuMEs were the most developed CuSe nanocube microelectrodes. They demonstrated the highest catalytic CO2RR activity in both aqueous and organic electrolytes, with a less negative onset potential and cathodic potential to reach −10 mA cm−2 and low polarization resistance, high mass activity, and stability. Due to the CuSe NCs-B-based Cu microelectrodes’ high crystallinity, large volume of electrochemical active sites, low polarization resistance, hierarchical 3D nanocube-like morphology, and integrated intrinsic catalytic activity, they were able to achieve high electrocatalytic performance. Recently, Alnaser co-workers reported femtosecond laser-assisted tuning of the selectivity Cu for generating ethane and propanol (Ali et al., 2024). The conversion from C1 products to ethane and propanol is mainly triggered by the laser-assisted modification of the surface of Cu. Simultaneously, the so-formed hierarchical porous structures may cause the [111], [200], and [220] Cu2O facets for the stabilization. These processes are responsible for the change in the product composition. Furthermore, the adsorption strength is enhanced, which increases the tendency for C–C coupling and, as a result, the selectivity for C2+ products.
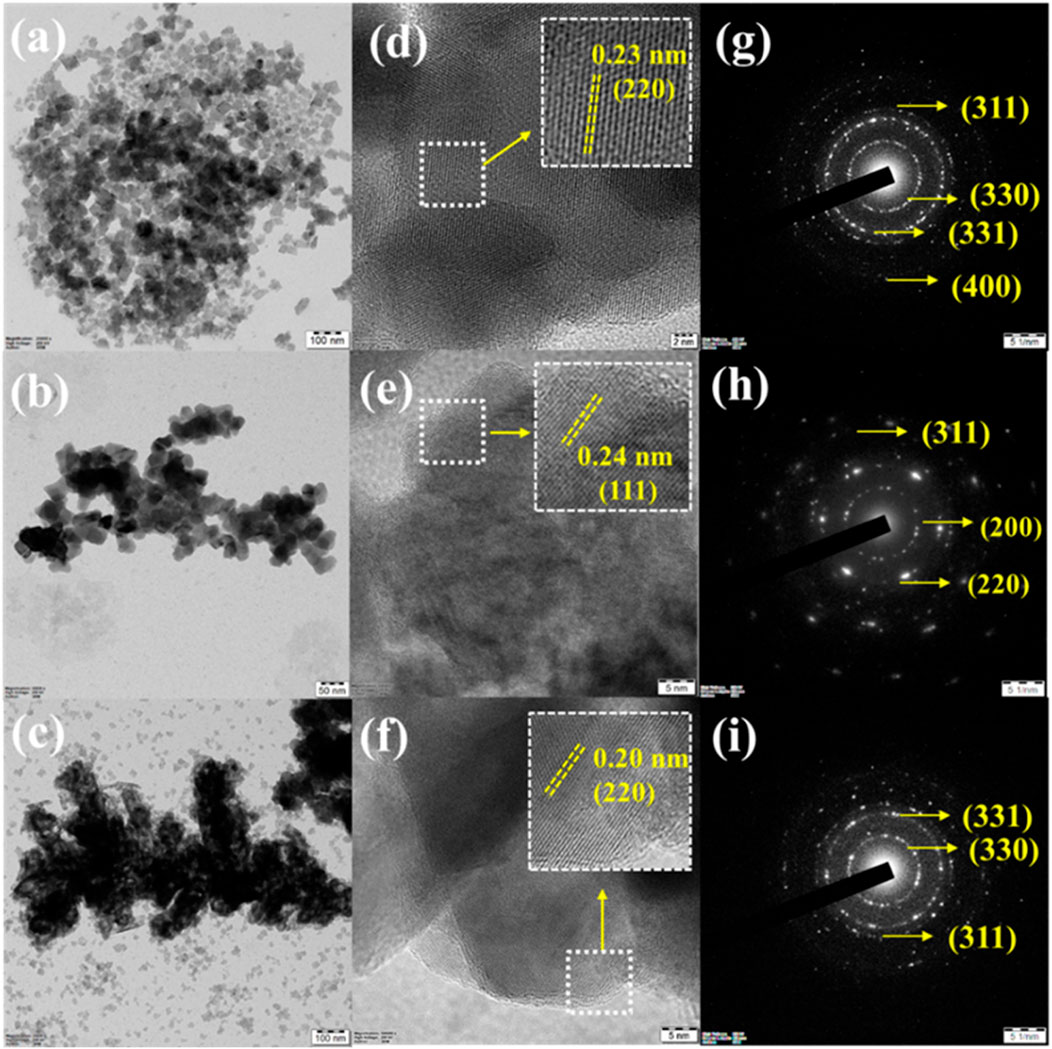
Figure 9. TEM images for CuSe NCs-A (A), CuSe NCs-B (B), and CuSe NCs-C (C). HRTEM images for CuSe NCs-A (D), CuSe NCs-B (E), and CuSe NCs-C (F). SAED patterns of CuSe NCs-A (G), CuSe NCs-B (H), and CuSe NCs-C (I). The as-fabricated nanostructures were called CuSe NCs-A, CuSe NCs-B, and CuSe NCs-C for the Cu/Se molar ratios of 1:1; 1:3, and 3:1, respectively (Elakkiya and Maduraiveeran, 2021b).
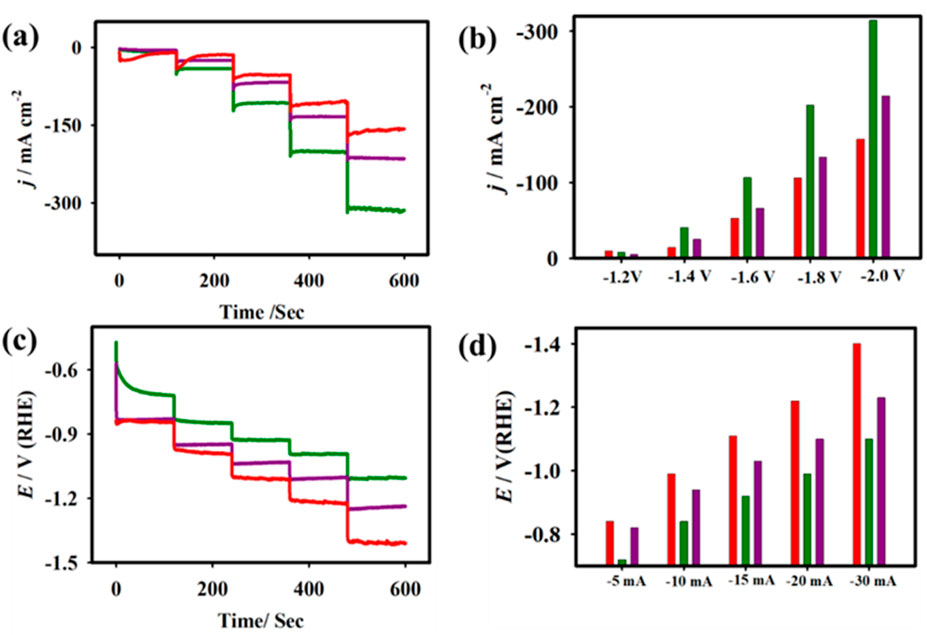
Figure 10. Chronoamperometric studies (A) and the plot of i vs. Eapp (B). Chronopotentiometric studies (C) and the plot of electrode potential vs. japp (D) for the CuSe NCs-A (red), CuSe NCs-B (green), and CuSe NCs-C (violet) electrodes. Electrolyte: 1.0 M aqueous KHCO3 solution under saturated CO2 (Elakkiya and Maduraiveeran, 2021a).
Chen and co-workers carried out co-electrolysis of CO2 and H2O to generate hydrocarbon with proton-conducting electrolysis cells (PCECs) (Figure 11) (Ye et al., 2023). The rough surface of CeO2 modified with the BaCe0.7Zr0.1Y0.1Yb0.1O3-δ (BZCYYb) electrode was employed for the production of CH4 at a low current density. The CeO2-modified electrode showed low polarization resistance and possessed a high-intensity interface process on the solid–gas interface. In particular, at low current density, the additional hydrogen-induced component analysis interference may be effectively removed. On the other hand, the hydrogen gas produced during electrolysis may prevent CO2 from oxidizing the fuel electrode. The adsorption of CO and proton bonding on the surface of CeO2 was weak compared to that on the BZCYYb surface. Although the first factor results in a more robust hydrogen bond with CO and makes it easy for CO hydrogenation, the second factor encourages the protons to migrate after being driven to the electrode surface. The higher CH4 selectivity is attained on the CeO2-modified electrode, majorly lowering the energy barrier for further CO hydrogenation. The advanced spectroscopic measurements integrated with density functional theory computations reveal that the modified CeO2 improves the formation of CH4 by modifying reactant adsorption and facilitating proton transport during the hydrogenation process.
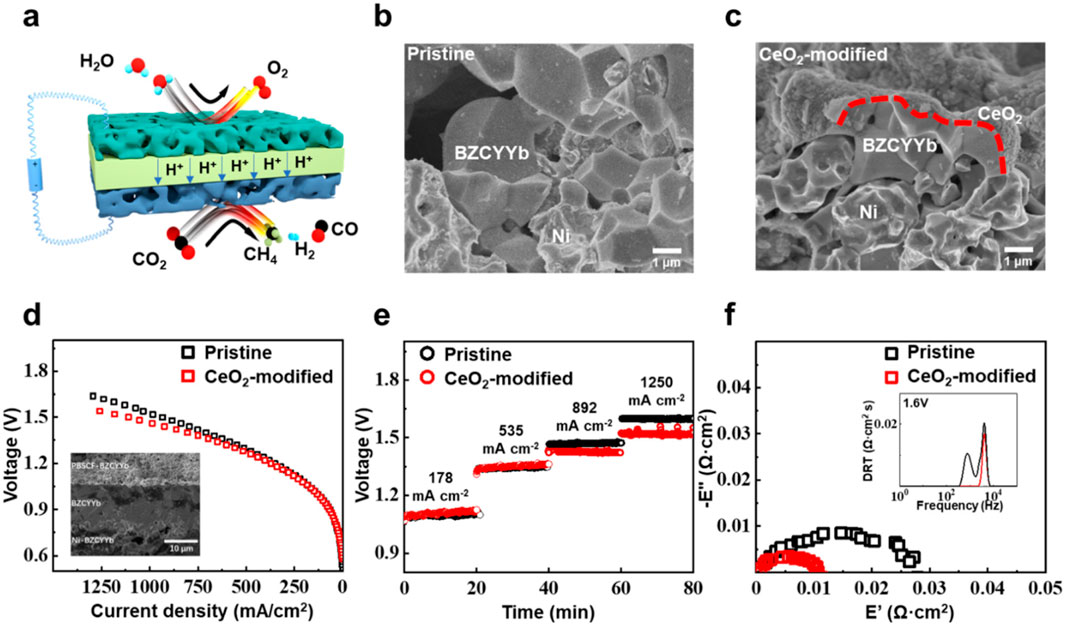
Figure 11. Pictorial representation of water electrolysis in integration with CO2 reduction using PCECs (A). SEM images of the pure (B) and CeO2-modified electrodes with Ni-BZCYYb (C); i-E curves of the electrode (D). Inset: SEM image of the Ni–BZCYYb|BZCYYb|PBSCF-BZCYYb electrode (cross-sectional area). Measured j at different Eapp (E). EIS of the electrode (F). Inset: DRT measurements of the EIS with the pure and CeO2-modified electrodes (Ye et al., 2023).
4 Conclusion
Owing to future socio-economic development, the replacement of fossil fuels with sustainable energy sources and carriers that are environment-friendly and inexpensive is crucial. The generation of hydrogen and hydrocarbon through water electrolysis (OER/HER) and water–CO2 co-electrolysis (OER/CO2RR) is supposed to be the emergent energy carrier (Li W. et al., 2022). These electrochemical reactions are key chemical conversion courses that facilitate renewable energy transformations. Thus, the establishment of alternative low-cost, high earth-abundance, great catalytic activity, and durable electrocatalysts for the possible renewable technologies is both highly preferred and a grand challenge (Marimuthu et al., 2024; Yao et al., 2024). The search for renewable energy motivates the establishment of progressive nanostructured catalysts for various electrocatalytic reactions, including OER, HER, and CO2RR, for efficient energy conversion and storage systems. In the present review, a variety of low-cost and high-performance electrocatalysts based on transition metal-based nanomaterials are discussed for water electrolysis (OER/HER) and water–CO2 co-electrolysis (OER/CO2RR).
5 Challenges and outlook
The advantages of the catalysts mentioned in this study include the employability at room pressure and temperature, the utilization of plentiful water molecules rather than complex and costly H2 for hydrogenation reactions, an extremely dispersed infrastructure, and the capability to attain good specificity toward the optimal products limiting the development of wasteful/toxic co-products. The as-developed nanostructured transition metal-based catalysts in this study demonstrated good catalytic activity in terms of low onset potential, high current densities, low overpotential, high mass activities, and long-term stability. The improved catalytic performance is attributed to the collective effect of heterostructures, a substantial volume of electrochemical active situates, and the establishment of fast electrode kinetics of the nanostructured catalytic materials.
In general, the design of electrocatalysts based on non-metals can still not compete with metal-based candidates concerning efficiency for OER, HER, and CO2RR electrocatalysis. Thus, the establishment of nanocomposites as multifunctional electrocatalysts warrants a deep synergistic interaction among the catalytic sites, increasing the number of active sites, and interfaces is a practical approach that should be pursued. The incorporation of S, P, and Se on transition metal nanomaterials is an effective strategy for engineering the electronic structure and electrochemical properties for improved catalytic activity and durability. In addition to the optimization of nanostructured electrode materials, there are several approaches for reducing the overpotential, increase of current density, and total Ohmic resistance in energy systems through enhanced ionic movement (using ultrasonography at the gas-evolving electrodes and electrolyte, centrifugal acceleration field, mechanical stirring, and gravity). Most of these non-homogenous electrochemical processes share certain features, such as occurring at solid–liquid interfaces and consisting of multi-step ion/electron coupled electron flow, which leads to extremely slow electron transfer kinetics and highly demanding efficient electrocatalysts, even though the precise mechanism varies. The restricted functionality, capacity, effectiveness, and durability of the catalysts prevent them from being industrialized, even though great efforts have been made to improve the kinetics of electrochemical reactions. Moreover, the interchange of issues faced in the various domains of devices among these groups is greatly needed and stimulated.
Author contributions
GM: conceptualization, funding acquisition, validation, writing–original draft, and writing–review and editing.
Funding
The author(s) declare that financial support was received for the research, authorship, and/or publication of this article. Author GM expresses his thanks to the Central Power Research Institute (CPRI), Bangalore (Ref.: CPRI/R&D/TC/GDEC/2022), for financial support.
Conflict of interest
The author declares that the research was conducted in the absence of any commercial or financial relationships that could be construed as a potential conflict of interest.
Publisher’s note
All claims expressed in this article are solely those of the authors and do not necessarily represent those of their affiliated organizations, or those of the publisher, the editors, and the reviewers. Any product that may be evaluated in this article, or claim that may be made by its manufacturer, is not guaranteed or endorsed by the publisher.
References
Abdelghafar, F., Xu, X., Guan, D., Lin, Z., Hu, Z., Ni, M., et al. (2024). New nanocomposites derived from cation-nonstoichiometric bax(Co, Fe, Zr, Y)O3−δ as efficient electrocatalysts for water oxidation in alkaline solution. ACS Mater. Lett. 6 (7), 2985–2994. doi:10.1021/acsmaterialslett.4c00789
Abdelghafar, F., Xu, X., Jiang, S. P., and Shao, Z. (2022). Designing single-atom catalysts toward improved alkaline hydrogen evolution reaction. Mater. Rep. Energy 2 (3), 100144. doi:10.1016/j.matre.2022.100144
Ahmad, S., Boltaev, G., Egilmez, M., Abuzaid, W., Alawadhi, H., Kannan, A., et al. (2024). Impact of femtosecond laser surface structuring on NiCoCr and NiCoV medium entropy alloy systems for an overall electrochemical water splitting. Int. J. Hydrogen Energy 59, 1094–1105. doi:10.1016/j.ijhydene.2024.02.056
Ali, A., Mathew, S., Ahmad, S., Ialyshev, V., Mustafa, F., Boltaev, G., et al. (2024). Femtosecond laser-enabled facile tuning of Cu selectivity towards long-chain products in CO2 electroreduction. J. CO2 Util. 85, 102880. doi:10.1016/j.jcou.2024.102880
Anantharaj, S., and Noda, S. (2020). Amorphous catalysts and electrochemical water splitting: an untold story of harmony. Small 16 (2), e1905779. doi:10.1002/smll.201905779
Arias-Hernandez, L. A., Valencia-Ortega, G., Martinez-Garcia, C. R., and Angulo-Brown, F. (2024). Energy conversion theorems for some linear steady states. Phys. Rev. E 109 (1-1), 014107. doi:10.1103/physreve.109.014107
Arivazhagan, M., Shankar, A., and Maduraiveeran, G. (2020). Hollow sphere nickel sulfide nanostructures-based enzyme mimic electrochemical sensor platform for lactic acid in human urine. Mikrochim. Acta 187 (8), 468. doi:10.1007/s00604-020-04431-3
Bagemihl, I., Cammann, L., Pérez-Fortes, M., van Steijn, V., and van Ommen, J. R. (2023). Techno-economic assessment of CO(2) electrolysis: how interdependencies between model variables propagate across different modeling scales. ACS Sustain Chem. Eng. 11 (27), 10130–10141. doi:10.1021/acssuschemeng.3c02226
Barecka, M. H., Ager, J. W., and Lapkin, A. A. (2021). Techno-economic assessment of emerging CO(2) electrolysis technologies. Star. Protoc. 2 (4), 100889. doi:10.1016/j.xpro.2021.100889
Bhargava, S. S., Azmoodeh, D., Chen, X., Cofell, E. R., Esposito, A. M., Verma, S., et al. (2021). Decreasing the energy consumption of the CO2 electrolysis process using a magnetic field. ACS Energy Lett. 6 (7), 2427–2433. doi:10.1021/acsenergylett.1c01029
Bi, J., Li, P., Liu, J., Wang, Y., Song, X., Kang, X., et al. (2023). High-rate CO(2) electrolysis to formic acid over a wide potential window: an electrocatalyst comprised of indium nanoparticles on chitosan-derived graphene. Angew. Chem. Int. Ed. Engl. 62 (36), e202307612. doi:10.1002/anie.202307612
Bonod, N., Brianceau, P., Daurios, J., Grosjean, S., Roquin, N., Gleyze, J. F., et al. (2023). Linear-to-circular polarization conversion with full-silica meta-optics to reduce nonlinear effects in high-energy lasers. Nat. Commun. 14 (1), 5383. doi:10.1038/s41467-023-40709-9
Bordet, A., and Leitner, W. (2023). Adaptive catalytic systems for chemical energy conversion. Angew. Chem. Int. Ed. Engl. 62 (33), e202301956. doi:10.1002/anie.202301956
Bose, R., Jothi, V. R., Velusamy, D. B., Arunkumar, P., and Yi, S. C. (2018). A highly effective, stable oxygen evolution catalyst derived from transition metal selenides and phosphides. Part. and Part. Syst. Charact. 35 (8), 1800135. doi:10.1002/ppsc.201800135
Butburee, T., Bai, Y., Wang, H., Chen, H., Wang, Z., Liu, G., et al. (2018). 2D porous TiO(2) single-crystalline nanostructure demonstrating high photo-electrochemical water splitting performance. Adv. Mater 30 (21), e1705666. doi:10.1002/adma.201705666
Cai, L., Bai, H., Kao, C., Jiang, K., Pan, H., Lu, Y., et al. (2024a). Platinum-ruthenium dual-atomic sites dispersed in nanoporous Ni(0.85) Se enabling ampere-level current density hydrogen production. Small 20, e2311178. doi:10.1002/smll.202311178
Cai, M., Shi, H., Zhang, Y., Qu, J., Wang, H., Guo, Y., et al. (2024b). Rechargeable Zn-H2O hydrolysis battery for hydrogen storage and production. Angew. Chem. Int. Ed. Engl. 136, e202404025. doi:10.1002/ange.202404025
Cao, Y., Yan, Y., Wen, Y., Cao, M., Li, Y., Xie, H., et al. (2024). Fe-based metal organic framework-derived FeNiP/N-doped carbon heterogeneous core-shell structures for oxygen evolution. Inorg. Chem. 63 (7), 3599–3609. doi:10.1021/acs.inorgchem.3c04512
Chai, H., Ma, X., Dang, Y., Zhang, Y., Yue, F., and Pang, X. (2024). Triple roles of Ni(OH)(2) promoting the electrocatalytic activity and stability of Ni(3)S(4)@Ni(OH)(2) in anion exchange membrane water electrolyzers. J. Colloid Interface Sci. 654 (Pt A), 66–75. doi:10.1016/j.jcis.2023.10.021
Chang, J., Shi, Y., Wu, H., Yu, J., Jing, W., Wang, S., et al. (2024a). Oxygen radical coupling on short-range ordered Ru atom arrays enables exceptional activity and stability for acidic water oxidation. J. Am. Chem. Soc. 146, 12958–12968. doi:10.1021/jacs.3c13248
Chang, J., Song, F., Hou, Y., Wu, D., Xu, F., Jiang, K., et al. (2024b). Molybdenum, tungsten doped cobalt phosphides as efficient catalysts for coproduction of hydrogen and formate by glycerol electrolysis. J. Colloid Interface Sci. 665, 152–162. doi:10.1016/j.jcis.2024.03.119
Chang, Y., Lu, X., Wang, S., Li, X., Yuan, Z., Bao, J., et al. (2024c). Built-in electric field boosted overall water electrolysis at large current density for the heterogeneous Ir/CoMoO(4) nanosheet arrays. Small 20, e2311763. doi:10.1002/smll.202311763
Chatenet, M., Pollet, B. G., Dekel, D. R., Dionigi, F., Deseure, J., Millet, P., et al. (2022). Water electrolysis: from textbook knowledge to the latest scientific strategies and industrial developments. Chem. Soc. Rev. 51 (11), 4583–4762. doi:10.1039/d0cs01079k
Chen, C., Meng, L., Cao, L., Zhang, D., An, S., Liu, L., et al. (2024a). Phase engineering of zirconium MOFs enables efficient osmotic energy conversion: structural evolution unveiled by direct imaging. J. Am. Chem. Soc. 146 (17), 11855–11865. doi:10.1021/jacs.4c00716
Chen, G., Chen, W., Lu, R., Ma, C., Zhang, Z., Huang, Z., et al. (2023a). Near-atomic-scale superfine alloy clusters for ultrastable acidic hydrogen electrocatalysis. J. Am. Chem. Soc. 145 (40), 22069–22078. doi:10.1021/jacs.3c07541
Chen, X., Wang, X. T., Le, J. B., Li, S. M., Wang, X., Zhang, Y. J., et al. (2023b). Revealing the role of interfacial water and key intermediates at ruthenium surfaces in the alkaline hydrogen evolution reaction. Nat. Commun. 14 (1), 5289. doi:10.1038/s41467-023-41030-1
Chen, X., Zhao, J., Zhao, Z., Zhang, W., and Wang, X. (2024b). Surface reconstruction in amorphous CoFe-based hydroxides/crystalline phosphide heterostructure for accelerated saline water electrolysis. J. Colloid Interface Sci. 659, 821–832. doi:10.1016/j.jcis.2024.01.024
Chen, Y., Tan, Z., Wang, E., Yin, J., Luo, L., Shen, S., et al. (2023c). Progress and prospects of dealloying methods for energy-conversion electrocatalysis. Dalton Trans. 52 (22), 7370–7382. doi:10.1039/d3dt00449j
Chen, Y., Wang, Y., Liu, B., Zhang, C., Sun, D., Liu, H., et al. (2024c). Room-temperature sulfur doped NiMoO(4) with enhanced conductivity and catalytic activity for efficient hydrogen evolution reaction in alkaline media. J. Colloid Interface Sci. 664, 469–477. doi:10.1016/j.jcis.2024.03.079
Deng, L., Hung, S., Lin, Z., Zhang, Y., Zhang, C., Hao, Y., et al. (2023a). Valence oscillation of Ru active sites for efficient and robust acidic water oxidation. Adv. Mater 35 (48), e2305939. doi:10.1002/adma.202305939
Deng, M., Tang, Y., Lu, Z., Wang, Y., and Lin, Y. (2023b). Self-supporting Mn-RuO(2) nanoarrays for stable oxygen evolution reaction in acid. Molecules 28 (23), 7727. doi:10.3390/molecules28237727
Dinges, I., Depentori, I., Gans, L., Holtmann, D., Waldvogel, S. R., and Stöckl, M. (2024). Coupling of CO(2) electrolysis with parallel and semi-automated biopolymer synthesis - ex-cell and without downstream processing. ChemSusChem 17 (8), e202301721. doi:10.1002/cssc.202301721
Dondapati, J. S., Govindhan, M., and Chen, A. (2022). Direct growth of three-dimensional nanoflower-like structures from flat metal surfaces. Chem. Commun. (Camb) 58 (79), 11127–11130. doi:10.1039/d2cc04358k
Ebbesen, S. D., Jensen, S. H., Hauch, A., and Mogensen, M. B. (2014). High temperature electrolysis in alkaline cells, solid proton conducting cells, and solid oxide cells. Chem. Rev. 114 (21), 10697–10734. doi:10.1021/cr5000865
Elakkiya, R., and Maduraiveeran, G. (2020). Two-dimensional earth-abundant transition metal oxides nanomaterials: synthesis and application in electrochemical oxygen evolution reaction. Langmuir 36 (17), 4728–4736. doi:10.1021/acs.langmuir.0c00714
Elakkiya, R., and Maduraiveeran, G. (2021a). Hierarchical three-dimensional copper selenide nanocube microelectrodes for improved carbon dioxide reduction reactions. Sustain. Energy and Fuels 5 (24), 6430–6440. doi:10.1039/d1se01458g
Elakkiya, R., and Maduraiveeran, G. (2021b). Iron sulphide rice grain nanostructures as potential electrocatalysts for an improved oxygen evolution reaction. Nanoscale 13 (35), 14837–14846. doi:10.1039/d1nr04138j
Elakkiya, R., Ramkumar, R., and Maduraiveeran, G. (2019). Flower-like nickel-cobalt oxide nanomaterials as bi-functional catalyst for electrochemical water splitting. Mater. Res. Bull. 116, 98–105. doi:10.1016/j.materresbull.2019.04.016
Fan, K., Zhou, S., Xie, L., Jia, S., Zhao, L., Liu, X., et al. (2024). Interfacial assembly of 2D graphene-derived ion channels for water-based green energy conversion. Adv. Mater 36 (9), e2307849. doi:10.1002/adma.202307849
Fang, L., Liu, F., Ding, H., and Duan, C. (2024). High-performance reversible solid oxide cells for powering electric vehicles, long-term energy storage, and CO(2) conversion. ACS Appl. Mater Interfaces. doi:10.1021/acsami.4c00780
Farhan, A., Qayyum, W., Fatima, U., Nawaz, S., Balčiūnaitė, A., Kim, T. H., et al. (2024). Powering the future by iron sulfide type material (FexSy) based electrochemical materials for water splitting and energy storage applications: a review. Small 20, e2402015. doi:10.1002/smll.202402015
Gao, D., Liu, R., Biskupek, J., Kaiser, U., Song, Y., and Streb, C. (2019). Modular design of noble-metal-free mixed metal oxide electrocatalysts for complete water splitting. Angew. Chem. Int. Ed. 58 (14), 4644–4648. doi:10.1002/anie.201900428
Gao, D., Liu, S., Liu, R., and Streb, C. (2020). Bulk nanostructuring of janus-type metal electrodes. Chem. – A Eur. J. 26 (49), 11109–11112. doi:10.1002/chem.202001420
Geng, Y., Zhang, L., Li, M., He, Y., Lu, B., He, J., et al. (2024). Nano-confined effect and heterojunction promoted exciton separation for light-boosted osmotic energy conversion. Energy Convers. 20, e2309128. doi:10.1002/smll.202309128
Gonçalves, J. M., Martins, P. R., Araki, K., and Angnes, L. (2021). Recent progress in water splitting and hybrid supercapacitors based on nickel-vanadium layered double hydroxides. J. Energy Chem. 57, 496–515. doi:10.1016/j.jechem.2020.08.047
Guo, L. Y., Li, J., Lu, Z., and Zhang, J. (2023). Biomass-derived carbon-based multicomponent integration catalysts for electrochemical water splitting. ChemSusChem 16 (17), e202300214. doi:10.1002/cssc.202300214
Guo, Y., Guo, X., Song, C., Han, X., Liu, H., and Zhao, Z. (2019). Capsule-Structured copper–zinc catalyst for highly efficient hydrogenation of carbon dioxide to methanol. ChemSusChem 12 (22), 4904–4926. doi:10.1002/cssc.201902911
Guo, Y., Sun, X., Ding, S., Lu, J., Wang, H., Zhu, Y., et al. (2024). Charge-gradient sulfonated poly(ether ether ketone) membrane with enhanced ion selectivity for osmotic energy conversion. ACS Nano 18 (9), 7161–7169. doi:10.1021/acsnano.3c11944
Hatakeyama-Sato, K., and Oyaizu, K. (2023). Redox: organic robust radicals and their polymers for energy conversion/storage devices. Chem. Rev. 123 (19), 11336–11391. doi:10.1021/acs.chemrev.3c00172
He, Y., Liu, W., and Liu, J. (2024). MOF-based/derived catalysts for electrochemical overall water splitting. J. Colloid Interface Sci. 661, 409–435. doi:10.1016/j.jcis.2024.01.106
Hegazy, M. B. Z., Zander, J., Weiss, M., Simon, C., Gerschel, P., Sanden, S. A., et al. (2024). FeNi(2) S(4) -A potent bifunctional efficient electrocatalyst for the overall electrochemical water splitting in alkaline electrolyte. Small 20, e2311627. doi:10.1002/smll.202311627
Hua, W., Liu, T., Zheng, Z., Yuan, H., Xiao, L., Feng, K., et al. (2024). Pulse electrolysis turns on CO(2) methanation through N-confused cupric porphyrin. Angew. Chem. Int. Ed. Engl. 63 (12), e202315922. doi:10.1002/anie.202315922
Huang, J., Guo, X., Yue, G., Hu, Q., and Wang, L. (2018). Boosting CH3OH production in electrocatalytic CO2 reduction over partially oxidized 5 nm cobalt nanoparticles dispersed on single-layer nitrogen-doped graphene. ACS Appl. Mater. and Interfaces 10 (51), 44403–44414. doi:10.1021/acsami.8b14822
Jiang, X., Ke, L., Zhao, K., Yan, X., Wang, H., Cao, X., et al. (2024). Integrating hydrogen utilization in CO(2) electrolysis with reduced energy loss. Nat. Commun. 15 (1), 1427. doi:10.1038/s41467-024-45787-x
Kang, D., Kim, T. W., Kubota, S. R., Cardiel, A. C., Cha, H. G., and Choi, K. S. (2015). Electrochemical synthesis of photoelectrodes and catalysts for use in solar water splitting. Chem. Rev. 115 (23), 12839–12887. doi:10.1021/acs.chemrev.5b00498
Kim, J., Song, J. T., and Oh, J. (2020). Facile electrochemical synthesis of dilute AuCu alloy nanostructures for selective and long-term stable CO(2) electrolysis. J. Chem. Phys. 153 (5), 054702. doi:10.1063/5.0009340
Ko, Y. J., Lim, C., Jin, J., Kim, M. G., Lee, J. Y., Seong, T. Y., et al. (2024). Extrinsic hydrophobicity-controlled silver nanoparticles as efficient and stable catalysts for CO(2) electrolysis. Nat. Commun. 15 (1), 3356. doi:10.1038/s41467-024-47490-3
Kong, D., Xu, Q., Chu, N., Wang, H., Lim, Y. V., Cheng, J., et al. (2024a). Rational construction of 3D self-supported MOF-derived cobalt phosphide-based hollow nanowall arrays for efficient overall water splitting at large current density. Small 20, e2310012. doi:10.1002/smll.202310012
Kong, X., Xu, J., Ju, Z., and Chen, C. (2024b). Durable Ru nanocrystal with HfO(2) modification for acidic overall water splitting. Nanomicro Lett. 16 (1), 185. doi:10.1007/s40820-024-01384-7
Kortlever, R., Shen, J., Schouten, K. J. P., Calle-Vallejo, F., and Koper, M. T. M. (2015). Catalysts and reaction pathways for the electrochemical reduction of carbon dioxide. J. Phys. Chem. Lett. 6 (20), 4073–4082. doi:10.1021/acs.jpclett.5b01559
Kothandam, G., Singh, G., Guan, X., Lee, J. M., Ramadass, K., Joseph, S., et al. (2023). Recent advances in carbon-based electrodes for energy storage and conversion. Adv. Sci. (Weinh) 10 (18), e2301045. doi:10.1002/advs.202301045
Lee, G. R., Kim, J., Hong, D., Kim, Y. J., Jang, H., Han, H. J., et al. (2023). Efficient and sustainable water electrolysis achieved by excess electron reservoir enabling charge replenishment to catalysts. Nat. Commun. 14 (1), 5402. doi:10.1038/s41467-023-41102-2
Li, A., Tang, X., Cao, R., Song, D., Wang, F., Yan, H., et al. (2024a). Directed surface reconstruction of Fe modified Co(2)VO(4) spinel oxides for water oxidation catalysts experiencing self-terminating surface deterioration. Adv. Mater 36, e2401818. doi:10.1002/adma.202401818
Li, A., Wu, L., Cui, H., Song, Y., Zhang, X., and Li, X. (2024b). Unlocking a sustainable future for plastics: a chemical-enzymatic pathway for efficient conversion of mixed waste to mhet and energy-saving pet recycling. ChemSusChem 17, e202301612. doi:10.1002/cssc.202301612
Li, D., Xiang, R., Yu, F., Zeng, J., Zhang, Y., Zhou, W., et al. (2024c). In situ regulating cobalt/iron oxide-oxyhydroxide exchange by dynamic iron incorporation for robust oxygen evolution at large current density. Adv. Mater 36 (5), e2305685. doi:10.1002/adma.202305685
Li, J., Zeng, H., Dong, X., Ding, Y., Hu, S., Zhang, R., et al. (2023a). Selective CO(2) electrolysis to CO using isolated antimony alloyed copper. Nat. Commun. 14 (1), 340. doi:10.1038/s41467-023-35960-z
Li, L., Yin, Z., Gao, Z., Wang, G., Li, Z., Wei, F., et al. (2022a). Copper as a single metal atom based photo-, electro-, and photoelectrochemical catalyst decorated on carbon nitride surface for efficient CO2 reduction: a review. Nano Res. Energy 1, 9120015. doi:10.26599/NRE.2022.9120015
Li, P., Bi, J., Liu, J., Zhu, Q., Chen, C., Sun, X., et al. (2023b). A crystal growth kinetics guided Cu aerogel for highly efficient CO(2) electrolysis to C(2+) alcohols. Chem. Sci. 14 (2), 310–316. doi:10.1039/d2sc04961a
Li, W., Yin, Z., Gao, Z., Wang, G., Li, Z., Wei, F., et al. (2022b). Bifunctional ionomers for efficient co-electrolysis of CO2 and pure water towards ethylene production at industrial-scale current densities. Nat. Energy 7 (9), 835–843. doi:10.1038/s41560-022-01092-9
Li, Z., Li, X., Wang, M., Wang, Q., Wei, P., Jana, S., et al. (2024d). KIr(4)O(8) nanowires with rich hydroxyl promote oxygen evolution reaction in proton exchange membrane water electrolyzer. Adv. Mater 36, e2402643. doi:10.1002/adma.202402643
Liu, H., Yang, C., Bian, T., Yu, H., Zhou, Y., and Zhang, Y. (2024a). Bottom-up growth of convex sphere with adjustable Cu(0)/Cu(I) interfaces for effective C2 production from CO2 electroreduction. Angew. Chem. Int. Ed. 63 (28), e202404123. doi:10.1002/ange.202404123
Liu, R., Anjass, M., Greiner, S., Liu, S., Gao, D., Biskupek, J., et al. (2020). Bottom-up design of bimetallic cobalt–molybdenum carbides/oxides for overall water splitting. Chem. – A Eur. J. 26 (18), 4157–4164. doi:10.1002/chem.201905265
Liu, Y., Zhang, M., Zhang, C., Zhang, H., and Wang, H. (2024b). An IrRuOx catalyst supported by oxygen-vacant Ta oxide for the oxygen evolution reaction and proton exchange membrane water electrolysis. Nanoscale 16, 9382–9391. doi:10.1039/d3nr06211b
Lu, X., Zhou, C., Delima, R. S., Lees, E. W., Soni, A., Dvorak, D. J., et al. (2024). Visualization of CO(2) electrolysis using optical coherence tomography. Nat. Chem. 16, 979–987. doi:10.1038/s41557-024-01465-5
Lv, H., Lin, L., Zhang, X., Li, R., Song, Y., Matsumoto, H., et al. (2021). Promoting exsolution of RuFe alloy nanoparticles on Sr(2)Fe(1.4)Ru(0.1)Mo(0.5)O(6-delta) via repeated redox manipulations for CO(2) electrolysis. Nat. Commun. 12 (1), 5665. doi:10.1038/s41467-021-26001-8
Maduraiveeran, G. (2021). Nanoporous structured mixed transition metal oxides nanomaterials for electrochemical energy conversion technologies. Mater. Lett. 283, 128763. doi:10.1016/j.matlet.2020.128763
Maduraiveeran, G., Sasidharan, M., and Jin, W. (2019). Earth-abundant transition metal and metal oxide nanomaterials: synthesis and electrochemical applications. Prog. Mater. Sci. 106, 100574. doi:10.1016/j.pmatsci.2019.100574
Mao, L., Huang, Y., Deng, H., Meng, F., Fu, Y., Wang, Y., et al. (2023). Synergy of ultrathin CoOx overlayer and nickel single atoms on hematite nanorods for efficient photo-electrochemical water splitting. Small 19 (7), e2203838. doi:10.1002/smll.202203838
Marimuthu, S., Shankar, A., and Maduraiveeran, G. (2023). FeCoP nanosheets on NiO nanoparticles as electrocatalysts: tuning and stabilizing active sites for water splitting. Chem. Commun. 59 (18), 2600–2603. doi:10.1039/d2cc06386g
Marimuthu, S., Yabesh, N. R. K., and Maduraiveeran, G. (2024). Metal-organic frameworks-based single-atom catalysts: from synthesis to sustainable electrocatalytic systems. Mater. Today Chem. 37, 102035. doi:10.1016/j.mtchem.2024.102035
Mavrokefalos, C. K., Kaeffer, N., Liu, H., Krumeich, F., and Copéret, C. (2020). Small and narrowly distributed copper nanoparticles supported on carbon prepared by surface organometallic chemistry for selective hydrogenation and CO2 electroconversion processes. ChemCatChem 12 (1), 305–313. doi:10.1002/cctc.201901414
McHugh, P. J., Stergiou, A. D., and Symes, M. D. (2020). Decoupled electrochemical water splitting: from fundamentals to applications. Adv. Energy Mater. 10 (44), 2002453. doi:10.1002/aenm.202002453
Mekete Meshesha, M., Gautam, J., Chanda, D., Gwon Jang, S., and Lyong Yang, B. (2023). Enhancing the electrochemical activity of zinc cobalt sulfide via heterojunction with MoS(2) metal phase for overall water splitting. J. Colloid Interface Sci. 652 (Pt A), 272–284. doi:10.1016/j.jcis.2023.08.005
Meng, Y., Zhang, X., Hung, W. H., He, J., Tsai, Y. S., Kuang, Y., et al. (2019). Highly active oxygen evolution integrated with efficient CO2 to CO electroreduction. Proc. Natl. Acad. Sci. 116 (48), 23915–23922. doi:10.1073/pnas.1915319116
Niu, W., and Yang, Y. (2018). Graphitic carbon nitride for electrochemical energy conversion and storage. ACS Energy Lett. 3 (11), 2796–2815. doi:10.1021/acsenergylett.8b01594
O’Brien, C. P., Miao, R. K., Shayesteh Zeraati, A., Lee, G., Sargent, E. H., and Sinton, D. (2024). CO2 electrolyzers. Chem. Rev. 124 (7), 3648–3693. doi:10.1021/acs.chemrev.3c00206
Patra, K. K., and Gopinath, C. S. (2023). CO(2) electrolysis towards large scale operation: rational catalyst and electrolyte design for efficient flow-cell. Chem. Commun. (Camb) 59 (45), 6774–6795. doi:10.1039/d3cc01231j
Pei, Y., Zhong, H., and Jin, F. (2021). A brief review of electrocatalytic reduction of CO2—materials, reaction conditions, and devices. Energy Sci. and Eng. 9 (7), 1012–1032. doi:10.1002/ese3.935
Pimlott, D. J. D., Jewlal, A., Kim, Y., and Berlinguette, C. P. (2023). Oxygen-resistant CO(2) reduction enabled by electrolysis of liquid feedstocks. J. Am. Chem. Soc. 145 (48), 25933–25937. doi:10.1021/jacs.3c08930
Pimlott, D. J. D., Kim, Y., and Berlinguette, C. P. (2024). Reactive carbon capture enables CO(2) electrolysis with liquid feedstocks. Acc. Chem. Res. 57 (7), 1007–1018. doi:10.1021/acs.accounts.3c00571
Pu, Z., Luo, Y., Asiri, A. M., and Sun, X. (2016). Efficient electrochemical water splitting catalyzed by electrodeposited nickel diselenide nanoparticles based film. ACS Appl. Mater Interfaces 8 (7), 4718–4723. doi:10.1021/acsami.5b12143
Raveendran, A., Chandran, M., and Dhanusuraman, R. (2023). A comprehensive review on the electrochemical parameters and recent material development of electrochemical water splitting electrocatalysts. RSC Adv. 13 (6), 3843–3876. doi:10.1039/d2ra07642j
Rebouillat, S., Lyons, M. E., Brandon, M. P., and Doyle, R. L. (2011). Paving the way to the integration of smart nanostructures: Part II: nanostructured microdispersed hydrated metal oxides for electrochemical energy conversion and storage applications. Int. J. Electrochem. Sci. 6 (11), 5830–5917. doi:10.1016/s1452-3981(23)18448-3
Reddy, N. R., Kumar, A. S., Reddy, P. M., Kakarla, R. R., Joo, S. W., and Aminabhavi, T. M. (2023). Novel rhombus Co(3)O(4)-nanocapsule CuO heterohybrids for efficient photocatalytic water splitting and electrochemical energy storage applications. J. Environ. Manage 325 (Pt B), 116650. doi:10.1016/j.jenvman.2022.116650
Ren, H., Cheng, C., Yin, P., Qin, Q., and Dai, L. (2023). Ammonia-assisted synthesis of low-crystalline FeCo hydroxides for efficient electrochemical overall water splitting. Nanoscale 15 (26), 10985–10989. doi:10.1039/d3nr01574b
Roy, S., Li, Z., Chen, Z., Mata, A. C., Kumar, P., Sarma, S. C., et al. (2024). Cooperative copper single-atom catalyst in 2D carbon nitride for enhanced CO(2) electrolysis to methane. Adv. Mater 36 (13), e2300713. doi:10.1002/adma.202300713
Sabir, A. S., Pervaiz, E., Khosa, R., and Sohail, U. (2023). An inclusive review and perspective on Cu-based materials for electrochemical water splitting. RSC Adv. 13 (8), 4963–4993. doi:10.1039/d2ra07901a
Sadeghi, E., Peighambardoust, N. S., Chamani, S., and Aydemir, U. (2023). Designing in situ grown ternary oxide/2D Ni-bdc MOF nanocomposites on nickel foam as efficient electrocatalysts for electrochemical water splitting. ACS Mater Au 3 (2), 143–163. doi:10.1021/acsmaterialsau.2c00073
Santos, H. L. S., Corradini, P. G., Medina, M., Dias, J. A., and Mascaro, L. H. (2020). NiMo–NiCu inexpensive composite with high activity for hydrogen evolution reaction. ACS Appl. Mater. and Interfaces 12 (15), 17492–17501. doi:10.1021/acsami.0c00262
Shankar, A., Elakkiya, R., and Maduraiveeran, G. (2020). Self-supported fabrication and electrochemical water splitting study of transition-metal sulphide nanostructured electrodes. New J. Chem. 44 (13), 5071–5078. doi:10.1039/d0nj00192a
Shankar, A., and Maduraiveeran, G. (2022). Bimetallic iron cobalt oxide nanoclusters embedded on three-dimensional flower-like iron cobalt oxide nanosheets for improved oxygen evolution reaction. Energy Adv. 1 (8), 562–571. doi:10.1039/d2ya00095d
Shankar, A., Marimuthu, S., and Maduraiveeran, G. (2023). Heterostructured iron-cobalt sulfides nanoclusters entrenched in 3D-nanosheets as high-efficient electrocatalysts for oxygen evolution reaction. Int. J. Hydrogen Energy 48 (21), 7683–7697. doi:10.1016/j.ijhydene.2022.11.227
Sheng, M., Yang, Y., Bin, X., and Que, W. (2023). One-step electrochemical synthesis and surface reconstruction of NiCoP as an electrocatalyst for bifunctional water splitting. Mater. (Basel) 16 (4), 1529. doi:10.3390/ma16041529
Shin, T. H., Myung, J. H., Verbraeken, M., Kim, G., and Irvine, J. T. S. (2015). Oxygen deficient layered double perovskite as an active cathode for CO2 electrolysis using a solid oxide conductor. Faraday Discuss. 182, 227–239. doi:10.1039/c5fd00025d
Song, Y., Min, J., Guo, Y., Li, R., Zou, G., Li, M., et al. (2024). Surface activation by single Ru atoms for enhanced high-temperature CO(2) electrolysis. Angew. Chem. Int. Ed. Engl. 63 (5), e202313361. doi:10.1002/anie.202313361
Song, Y., Zhang, X., Xie, K., Wang, G., and Bao, X. (2019). High-temperature CO(2) electrolysis in solid oxide electrolysis cells: developments, challenges, and prospects. Adv. Mater 31 (50), e1902033. doi:10.1002/adma.201902033
Sun, D., Xu, X., Qin, Y., Jiang, S. P., and Shao, Z. (2020). Rational design of Ag-based catalysts for the electrochemical CO2 reduction to CO: a review. ChemSusChem 13 (1), 39–58. doi:10.1002/cssc.201902061
Timoshenko, J., Bergmann, A., Rettenmaier, C., Herzog, A., Arán-Ais, R. M., Jeon, H. S., et al. (2022). Steering the structure and selectivity of CO2 electroreduction catalysts by potential pulses. Nat. Catal. 5 (4), 259–267. doi:10.1038/s41929-022-00760-z
Varela, A. S., Ju, W., and Strasser, P. (2018). Molecular nitrogen–carbon catalysts, solid metal organic framework catalysts, and solid metal/nitrogen-doped carbon (MNC) catalysts for the electrochemical CO2 reduction. Adv. Energy Mater. 8 (30), 1703614. doi:10.1002/aenm.201703614
Wang, C., Li, C., Liu, J., and Guo, C. (2021). Engineering transition metal-based nanomaterials for high-performance electrocatalysis. Mater. Rep. Energy 1 (1), 100006. doi:10.1016/j.matre.2021.01.001
Wang, G. (2023). Isolated Cu(I) sites with delocalized electron state promote selective CO(2) electrolysis to methanol. Sci. Bull. (Beijing) 68 (5), 460–461. doi:10.1016/j.scib.2023.02.008
Wang, J., Cui, W., Liu, Q., Xing, Z., Asiri, A. M., and Sun, X. (2016). Recent progress in cobalt-based heterogeneous catalysts for electrochemical water splitting. Adv. Mater 28 (2), 215–230. doi:10.1002/adma.201502696
Wang, J., Gao, Y., Kong, H., Kim, J., Choi, S., Ciucci, F., et al. (2020a). Non-precious-metal catalysts for alkaline water electrolysis: operando characterizations, theoretical calculations, and recent advances. Chem. Soc. Rev. 49 (24), 9154–9196. doi:10.1039/d0cs00575d
Wang, Y., Su, H., He, Y., Li, L., Zhu, S., Shen, H., et al. (2020b). Advanced electrocatalysts with single-metal-atom active sites. Chem. Rev. 120 (21), 12217–12314. doi:10.1021/acs.chemrev.0c00594
Wei, P., Li, H., Li, R., Wang, Y., Liu, T., Cai, R., et al. (2023). The role of interfacial water in CO(2) electrolysis over Ni-N-C catalyst in a membrane electrode assembly electrolyzer. Small 19 (25), e2300856. doi:10.1002/smll.202300856
Wen, G., Ren, B., Zhang, X., Liu, S., Li, X., Lu, H., et al. (2023). Cu-in dual sites with sulfur defects toward superior ethanol electrosynthesis from CO(2) electrolysis. Adv. Mater, e2310822. doi:10.1002/adma.202310822
Woldu, A. R., Talebi, P., Yohannes, A. G., Xu, J., Wu, X., Siahrostami, S., et al. (2023). Insights into electrochemical CO(2) reduction on SnS(2): main product switch from hydrogen to formate by pulsed potential electrolysis. Angew. Chem. Int. Ed. Engl. 62 (29), e202301621. doi:10.1002/ange.202301621
Xu, D., Stevens, M. B., Cosby, M. R., Oener, S. Z., Smith, A. M., Enman, L. J., et al. (2019). Earth-abundant oxygen electrocatalysts for alkaline anion-exchange-membrane water electrolysis: effects of catalyst conductivity and comparison with performance in three-electrode cells. ACS Catal. 9 (1), 7–15. doi:10.1021/acscatal.8b04001
Xu, H., Xu, D., Deng, S., Li, D., Jiang, T., Li, L., et al. (2023). Photochemical and electrochemical co-regulation of the BiVO(4) photoanode for water splitting. Chem. Commun. (Camb) 59 (23), 3435–3438. doi:10.1039/d2cc07093f
Yan, X., Biemolt, J., Zhao, K., Zhao, Y., Cao, X., Yang, Y., et al. (2021). A membrane-free flow electrolyzer operating at high current density using earth-abundant catalysts for water splitting. Nat. Commun. 12 (1), 4143. doi:10.1038/s41467-021-24284-5
Yang, R., Lin, W., He, Y., Singh, M., and Fan, L. (2024). Revealing the detrimental CO(2) reduction effect of La(0.6)Sr(0.4)FeO(3-delta)-derived heterostructure in solid oxide electrolysis cells. iScience 27 (5), 109648. doi:10.1016/j.isci.2024.109648
Yang, Y., Li, P., Zheng, X., Sun, W., Dou, S. X., Ma, T., et al. (2022). Anion-exchange membrane water electrolyzers and fuel cells. Chem. Soc. Rev. 51 (23), 9620–9693. doi:10.1039/d2cs00038e
Yao, H., Le, F., Jia, W., Cao, Y., Sheng, R., Lu, Z., et al. (2023). Dual electronic modulations on NiFeV Hydroxide@FeOx boost electrochemical overall water splitting. Small 19 (36), e2301294. doi:10.1002/smll.202301294
Yao, Y., Shi, T., Chen, W., Wu, J., Fan, Y., Liu, Y., et al. (2024). A surface strategy boosting the ethylene selectivity for CO2 reduction and in situ mechanistic insights. Nat. Commun. 15 (1), 1257. doi:10.1038/s41467-024-45704-2
Ye, L., Zhang, M., Huang, P., Guo, G., Hong, M., Li, C., et al. (2017). Enhancing CO(2) electrolysis through synergistic control of non-stoichiometry and doping to tune cathode surface structures. Nat. Commun. 8, 14785. doi:10.1038/ncomms14785
Ye, Y., Lee, W., Pan, J., Sun, X., Zhou, M., Li, J., et al. (2023). Tuning the product selectivity of CO2/H2O co-electrolysis using CeO2-modified proton-conducting electrolysis cells. Energy and Environ. Sci. 16 (7), 3137–3145. doi:10.1039/d3ee01468a
You, B., and Sun, Y. (2018). Innovative strategies for electrocatalytic water splitting. Accounts Chem. Res. 51 (7), 1571–1580. doi:10.1021/acs.accounts.8b00002
Yu, X., Xu, Y., Li, L., Zhang, M., Qin, W., Che, F., et al. (2024). Coverage enhancement accelerates acidic CO(2) electrolysis at ampere-level current with high energy and carbon efficiencies. Nat. Commun. 15 (1), 1711. doi:10.1038/s41467-024-45988-4
Yuan, S., Huang, X., Kong, T., Yan, L., and Wang, Y. (2024). Organic electrode materials for energy storage and conversion: mechanism, characteristics, and applications. Acc. Chem. Res. 57, 1550–1563. doi:10.1021/acs.accounts.4c00016
Zhang, J., Zhao, S., Chen, B., Yin, S., Feng, Y., and Yin, Y. (2023a). Sulfidation of CoCuOx supported on nickel foam to form a heterostructure and oxygen vacancies for a high-performance anion-exchange membrane water electrolyzer. ACS Appl. Mater Interfaces 15 (39), 45756–45763. doi:10.1021/acsami.3c07120
Zhang, M., Wang, X., Ding, J., Ban, C., Feng, Y., Xu, C., et al. (2024a). Realizing ampere-level CO(2) electrolysis at low voltage over a woven network of few-atom-layer ultralong silverene nanobelts with ultrahigh aspect ratio by pairing with formaldehyde oxidation. Nanoscale 16 (14), 7076–7084. doi:10.1039/d4nr00361f
Zhang, Q., Duan, Z., Li, M., and Guan, J. (2020a). Atomic cobalt catalysts for the oxygen evolution reaction. Chem. Commun. 56 (5), 794–797. doi:10.1039/c9cc09007j
Zhang, W., Hu, Y., Ma, L., Zhu, G., Wang, Y., Xue, X., et al. (2018). Progress and perspective of electrocatalytic CO2 reduction for renewable carbonaceous fuels and chemicals. Adv. Sci. 5 (1), 1700275. doi:10.1002/advs.201700275
Zhang, W., Liu, M., Gu, X., Shi, Y., Deng, Z., and Cai, N. (2023b). Water electrolysis toward elevated temperature: advances, challenges and frontiers. Chem. Rev. 123 (11), 7119–7192. doi:10.1021/acs.chemrev.2c00573
Zhang, X., Tong, L., Shi, X., Li, Z., Xiao, Z., Liu, Y., et al. (2024b). Tailoring atomically local electric field of NiFe layered double hydroxides with Ag dopants to boost oxygen evolution kinetics. J. Colloid Interface Sci. 668, 502–511. doi:10.1016/j.jcis.2024.04.180
Zhang, Y., Feng, Y., Li, J., Xu, T., Wu, Y., Zhang, X., et al. (2024c). Multi-interfacial bridging engineering of flexible MXene film for efficient electromagnetic shielding and energy conversion. J. Colloid Interface Sci. 665, 733–741. doi:10.1016/j.jcis.2024.03.173
Zhang, Y., Li, K., Chen, M., Wang, J., and Liu, J. (2020b). Cu/Cu2O nanoparticles supported on vertically ZIF-L-coated nitrogen-doped graphene nanosheets for electroreduction of CO2 to ethanol. ACS Appl. Nano Mater. 3 (1), 257–263. doi:10.1021/acsanm.9b01935
Zhang, Z., Li, M., Gao, R., Yang, S., Ma, Q., Feng, R., et al. (2024d). Selective and scalable CO(2) electrolysis enabled by conductive zinc ion-implanted zeolite-supported cadmium oxide nanoclusters. J. Am. Chem. Soc. 146 (9), 6397–6407. doi:10.1021/jacs.4c01061
Zhang, Z., Song, Y., Zheng, S., Zhen, G., Lu, X., Kobayashi, T., et al. (2019). Electro-conversion of carbon dioxide (CO(2)) to low-carbon methane by bioelectromethanogenesis process in microbial electrolysis cells: the current status and future perspective. Bioresour. Technol. 279, 339–349. doi:10.1016/j.biortech.2019.01.145
Zheng, Y., Wang, J., Yu, B., Zhang, W., Chen, J., Qiao, J., et al. (2017). A review of high temperature co-electrolysis of H(2)O and CO(2) to produce sustainable fuels using solid oxide electrolysis cells (SOECs): advanced materials and technology. Chem. Soc. Rev. 46 (5), 1427–1463. doi:10.1039/c6cs00403b
Zhu, T., Han, J., Sun, T., Chen, J., Wang, S., Ren, S., et al. (2023). Interface-enhanced SiOx/Ru heterocatalysts for efficient electrochemical water splitting. ACS Appl. Mater Interfaces 15 (6), 8200–8207. doi:10.1021/acsami.2c21953
Keywords: transition metal-based nanomaterials, electrochemical methods, water electrolysis, CO2 electrolysis, energy conversion process
Citation: Maduraiveeran G (2024) Transition metal nanomaterial-based electrocatalysts for water and CO2 electrolysis: preparation, catalytic activity, and prospects. Front. Energy Res. 12:1433103. doi: 10.3389/fenrg.2024.1433103
Received: 15 May 2024; Accepted: 11 October 2024;
Published: 24 October 2024.
Edited by:
Sunday Olayinka Oyedepo, Bells University of Technology, NigeriaReviewed by:
Zongping Shao, Curtin University, AustraliaTianyi Liu, Brewer Science, United States
Dandan Gao, Johannes Gutenberg University Mainz, Germany
Copyright © 2024 Maduraiveeran. This is an open-access article distributed under the terms of the Creative Commons Attribution License (CC BY). The use, distribution or reproduction in other forums is permitted, provided the original author(s) and the copyright owner(s) are credited and that the original publication in this journal is cited, in accordance with accepted academic practice. No use, distribution or reproduction is permitted which does not comply with these terms.
*Correspondence: Govindhan Maduraiveeran, bWFkdXJhaWdAc3JtaXN0LmVkdS5pbg==