- 1Crop Health, Faculty of Agricultural and Environmental Sciences, University of Rostock, Rostock, Germany
- 2Department Technology Assessment, Leibniz Institute for Agricultural Engineering and Bioeconomy (ATB), Potsdam, Germany
Introduction: Plant seeds from weeds and energy crops have the potential to survive anaerobic digestion (AD). Species able to form physically dormant, i.e., hardseeded (HS) seeds seem particularly resistant. However, it is not yet known to what extent the storage of the digestate after AD affects seed viability.
Methods: Seed survival of five HS and six non-HS (NHS) species was investigated in three combinations of digestate storage (DS) and AD. First, untreated seeds were exposed to DS for maximum 12 weeks. To simulate short-circuited AD (ADshort) in biogas reactors, seeds were second subjected to lab-scale AD for 1 day before DS. Third, seeds of six species were exposed to full-scale AD (ADfull) followed by DS. Seed viability was determined using a combination of germination tests and tetrazolium staining. Viability was modeled as a function of exposure time.
Results and discussion: Seed viability was affected by DS, AD and AD + DS, but responses varied greatly between species and treatments. With increasing exposure time, viability decreased after a lag-phase, remained stable or even increased. The NHS species Cichorium intybus, Daucus carota, Echium vulgare, and Verbascum thapsus were most susceptible, with seed-killing close to 100% if DS was involved. The HS species Malva sylvestris, Melilotus albus and Melilotus officinalis were most resistant. They survived all treatments and were alive after 35 days of ADfull plus 3 months of DS. The resistance potential of the HS species Abutilon theophrasti and Malva alcea and of the NHS species Chenopodium album and two tomato varieties was intermediate. None of them survived ADfull + DS, but except A. theophrasti they were viable after ADshort + DS. With few exceptions, seed-killing by AD + DS was higher than that by AD alone. In conclusion, DS and AD + DS have the potential to reduce seed viability, but do not completely inactivate all species. Therefore, digestate can be contaminated with viable seeds and may lead to the spread of weeds, especially after a short-circuited AD. In order to ensure the sustainable use of digestates in terms of weeds, we recommend to investigate the factors contributing to seed inactivation and the quantity of seed introduced to AD.
1 Introduction
Anaerobic digestion (AD) of biomass in biogas plants produces both biogas and digestate. The latter, also referred to as biogas slurry, fermenting substrate or digester content can be defined as the residue that remains after the extraction of the methane-containing biogas from feedstock by AD (Schneider and Gerber, 2020). In Germany, one of the world’s leading countries in the production and use of biogas (International Energy Agency, 2020), approximately 82 million tons of digestate are produced every year (Fachagentur für Nachwachsende Rohstoffe e.V. (FNR), 2023). In recent years, digestate has been considered less of a by-product and more of a valuable raw material that can be used for various purposes (Wang and Lee, 2021). This is particularly relevant in terms of the sustainable use of resources and the circular economy. For instance, digestate can close nutrient cycles when used as organic fertilizer in agriculture (Walsh et al., 2012; Magrí, 2018). An important prerequisite for the sustainable use of organic fertilizers, however, is that it is free of environmentally harmful contaminants such as heavy metals, veterinary medical products, pathogenic microorganisms or weed seeds (Fröschle et al., 2015; Haupt et al., 2021; Nesse et al., 2022; Guan et al., 2024).
In the context of sustainable production, the portfolio of biogas feedstocks is constantly being expanded (e.g., Herrmann et al., 2016; Vollrath et al., 2016; Chen et al., 2019; Hartung et al., 2020). This also includes numerous (energy) plant species, which are mainly grown in Europe (Pavičić et al., 2022). Within Europe, two-thirds of biogas plants are located in Germany, and energy crops are an essential part of the substrate mix of its agricultural biogas plants. In 2019, the share of renewable resources, including energy crops, amounted to 46% (International Energy Agency, 2020; Fachagentur für Nachwachsende Rohstoffe e.V. (FNR), 2021). The energy crops should meet both ecological and economic requirements (e.g., Holland et al., 2015; Englund et al., 2020). Among them are flowering plants and wild plant mixtures that are intended to increase biodiversity in landscapes dominated by biogas maize (von Cossel and Lewandowski, 2016; Schulz et al., 2020). However, these species form seeds that may be mature at the time of harvest and could end up in the biomass destined as feedstock for AD. If the seeds survive the biogas production, they can contaminate the digestate and spread with it. This in turn could lead to an unwanted establishment of these species in fields not intended for them and cause costs for measures to control them. To ensure the sustainability of the digestate, it should therefore be clarified whether seeds of flowering wild plant species can survive the biogas production processes.
To date, it is known that seeds of some plant species can survive two process steps in the biogas production chain, namely, ensiling (e.g., Westerman et al., 2012; Piltz et al., 2017; Hahn et al., 2021) and AD in the biogas reactor (e.g., Šarapatka et al., 1993; Hassani et al., 2021; Hahn et al., 2022). The risk of contaminating and spreading viable seeds with digestate is mostly estimated based on these studies. However, the digestate is not applied to the fields immediately after AD, but is stored beforehand. In Germany, digestates and organic fertilizers must be stored in a (covered) digestate storage facility for at least 6 months, as methane can still be released from them (and odors occur). Furthermore, the application of organic fertilizers is subject to lock-up periods and time-spans in which the soil is optimally receptive, which is intended to ensure fertilization in line with demand and prevent leaching of nitrogen and phosphorus into the groundwater (Bundesministerium für Ernährung und Landwirtschaft, 2017; Düngebehörde, 2024). To realistically estimate whether viable seeds are still present in the digestate at the time of fertilization, both AD and the storage time of the digestate should therefore be taken into account. However, there are only a few studies on this topic. Much research on (weed) seed survival during storage of organic fertilizers has focused on raw or composted manure and slurry. The results ranged from complete destruction to reduction of viability and germinability to no response of the seeds (e.g., Shevkenek, 1934; Tompkins et al., 1998; Larney and Blackshaw, 2003; James et al., 2011; Aper et al., 2014). Survival in material that was actually anaerobically digested in a biogas reactor has, to our knowledge, been the subject of only one study to date: Strauß et al. (2012) investigated the germination of seeds after storage in an anaerobically digested silage mixture. They found that although germination was delayed, the seeds of some species remained germinable even after several weeks in the digestate store. In summary, the data available can currently only be described as rather limited. More general statements on the survival of seeds in digestate storage are therefore hardly possible.
With regard to a possible contamination of fields with viable seed through the application of digestate, it is of interest to what extent the viability of seeds changes during digestate storage. In order to gain a complete picture of seed survival probability, it is important to consider that both germinable and dormant seeds are viable and therefore able to survive (cf. Hahn et al., 2022). In this way, it can also be checked whether species with physical dormancy, also known as hardseededness (HS), which have a high survival risk in AD (Westerman and Gerowitt, 2013), also have this in the digestate. HS is based on impermeable layers in the seed or fruit coat and is common in the Fabaceae and Malvaceae families, among others (Baskin et al., 2000). When determining the survival of the seeds, it should also be considered that the storage of digestate is closely linked to the preceding AD in the biogas reactor. Without AD, there is no digestate and no digestate storage. Therefore, factors that affect seed survival in AD might also affect it in digestate. The most important being exposure time and temperature (e.g., Johansen et al., 2013; Zhou et al., 2020; Hahn et al., 2022). In particular, the duration of AD should be considered. On the one hand, because it is crucial for seed inactivation. While longer exposure times generally lead to greater seed-killing (Westerman and Gerowitt, 2013), increases in seed viability have been observed for HS species at short exposure times (Hahn et al., 2022). On the other hand, the duration of AD can vary greatly between individual biogas plants. In Germany, for example, the hydraulic retention time in mesophilic plants averages at 75 days (median), but varies between 17 and 321 days (calculated from vTI, 2009). In this context, the special case of short-circuiting plays a role, i.e., the feedstock passing through the reactor at a shorter retention time than required for optimal biogas yield and sanitization of the material (Ward et al., 2008). In this case, the seed would be exposed to AD for only 6–24 h (Turner et al., 1983; Baier et al., 2010; Eckford et al., 2012) before entering the digestate storage. A period of time that the seeds of quite some species can survive (e.g., Johansen et al., 2013; Baute et al., 2016; Hahn et al., 2022) under the mesophilic conditions (35°C and 42°C) mostly used in Europe (Kovačić et al., 2022).
The aim of this study was to determine the extent to which storage in digestate could affect the viability of plant seeds. Wild plants from a wildflower mixture designed for biogas production, two weeds, and tomato, which is the indicator for phytohygiene in compost in Germany (Bundesministerium für Umwelt, Naturschutz, nukleare Sicherheit und Verbraucherschutz, 1998), were used as model species. Half of the species were hardseeded. In detail, we aimed to answer the following questions:
(1) Does digestate storage influence seed viability in principle? Test: Digestate storage of untreated seeds at lab-scale.
(2) Does digestate storage affect seed that was previously exposed to
a. short-circuited anaerobic digestion? Test: Digestate storage of seeds short-circuited in lab-scale reactors.
b. anaerobic digestion in a full-scale, commercial biogas reactor for a common exposure time? Test: Digestate storage of seeds after full-scale anaerobic digestion.
2 Materials and methods
2.1 Plant seeds
2.1.1 Species selection
In this study, seed survival of eleven different species was investigated. All eleven species have already been investigated in mesophilic lab-scale AD (Hahn et al., 2022) and a detailed description of the species selection criteria can be found there. In short, the majority of species, was selected from a wildflower mixture specifically designed for biogas production (“BG70” by Saaten Zeller GmbH and Co. KG, Eichenbühl-Guggenberg, Germany, saaten-zeller.de). The selected wildflower species were: Cichorium intybus (blue dandelion), Daucus carota (wild carrot), Echium vulgare (viper’s bugloss), Malva alcea (rose mallow), Malva sylvestris (common mallow), Melilotus albus (white sweet clover), Melilotus officinalis (yellow sweet clover) and Verbascum thapsus (great mullein). In addition, two weed species, namely A.butilon theophrasti (velvetleaf) and Chenopodium album (common lambsquarters), were investigated. Finally, tomato (Lycopersicon esculentum) was chosen because it is an indicator organism for the phytohygiene of compost in Germany (Bundesministerium für Umwelt, Naturschutz, nukleare Sicherheit und Verbraucherschutz, 1998). Of the selected species, five were hardseeded (HS), namely Abutilon theophrasti, M. alcea, M. sylvestris, M. albus and M. officinalis. The other six species were non-hardseeded (NHS).
2.1.2 Seed acquisition and storage
Of the eleven species investigated, only one seed lot was examined for: C. album, C. intybus, D. carota, E. vulgare, M. sylvestris, M. albus, M. officinalis and V. thapsus. Seeds were propagated in 2014 or 2015 and obtained from “Herbiseed” (Twyford, United Kingdom, herbiseed.com), “Appels Wilde Samen GmbH” (appelswilde.de, Darmstadt, Germany) or, in case of C. album harvested from plants grown at the University of Rostock (Germany). Two different seed lots of M. alcea and A. theophrasti and two tomato varieties were tested, labeled “a” and “b”. Seeds of M. alcea were obtained from “AppelsWilde Samen GmbH” (see above). Seed lot A. theophrasti-a, was collected in 2008 in Spain by Paula R. Westerman. Seed lot A. theophrasti-b originated from A. theophrasti-a grown in a greenhouse at the University of Rostock (Germany). Tomato-a belongs to variety “St. Pierre” and was obtained from “Bingenheimer Saatgut AG” (bingenheimersaatgut.de/de, Echzell, Germany)] and tomato-b, variety “paprikaförmige,” was obtained from “Culinaris – Saatgut für Lebensmittel” (culinaris-saatgut.de, Göttingen, Germany)]. Seeds were stored at room temperature in the dark. The seeds of A. theophrasti-a harvested in 2008 had previously been stored at 7°C. More details on acquisition and storage of the seed lots are given by Hahn et al. (2022).
2.2 Treatments
Seeds were exposed to three different treatments, namely lab-scale digestate storage (DS) alone, lab-scale anaerobic digestion (AD) in experimental reactors followed by DS, and full-scale AD (ADfull) in a commercial biogas reactor followed by DS. In the context of this work, digestate is any content that is removed from the digester at any point during the biogas process (Schneider and Gerber, 2020).
The digestates used were stored in lab-scale vessels at the ATB Potsdam (Germany) (Heiermann and Plogsties, 2018). The vessels had a gross volume of 10 L and a filling volume of 5 L. The cover was not gas-tight. Storage took place at room temperature (Table 1). The origin and composition of digestate differed between the experiments involving lab- and full-scale AD (Table 1, cf. following sections). For the lab-scale experiments (2.2.1), a mixed sample from four lab-scale reactor contents (substrates) operated at 35°C was used as medium for digestate storage. For the full-scale experiments (2.2.2), an aliquot from the content of a commercial biogas reactor was applied for digestate storage after AD in this very reactor.
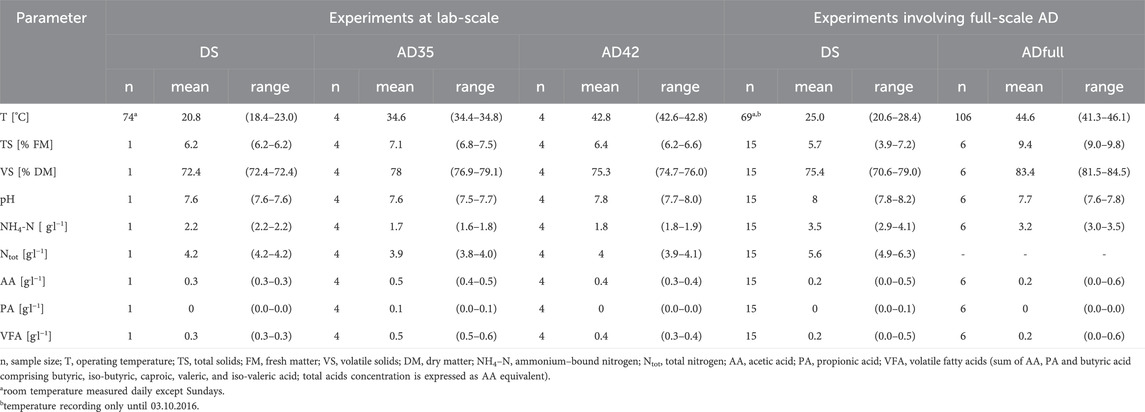
Table 1. Chemical characterization and operating temperature of digestates and substrates during lab-scale digestate storage (DS) and anaerobic digestion at 35 or 42°C (AD35, AD42) as well as full-scale anaerobic digestion in a commercial biogas reactor (ADfull).
2.2.1 Digestate storage and short-circuited AD plus digestate storage
The effect of DS alone and in combination with short-circuited AD (AD + DS) on seed viability was investigated at lab-scale. The experiments ran from March 30 to 23 June 2015. Seed exposure to mesophilic AD in the lab-scale reactors was carried out at the ATB Potsdam (Germany) as described by Hahn et al. (2022). In brief, AD took place in continuously stirred reactors with a working volume of 8 L that were fed on maize silage and cattle slurry. Half of the reactors were run at 35°C (AD35), while the other half was run at 42°C (AD42). The chemical characteristics of the substrates can be found in Table 1. For seed exposure to short-circuited AD, untreated seeds were placed in fine-mesh polyester bags that were attached to the reactors’ stirrer. The 24-h exposure of the seeds to AD35 and AD42 was conducted in two replicates per seed lot from March 30 to 31, 2015. One replicate contained 300 seeds (Supplementary Table S1). To test the effect of DS or AD + DS, untreated or short-circuited seeds were stored submerged in the digestate vessels for 27, 56 or 83 days (Supplementary Figure S1).
2.2.2 Full-scale AD plus digestate storage
Experiments to investigate the effect of full-scale AD (ADfull) and subsequent lab-scale DS on seed viability ran from May 27 to 8 December 2016. The procedure for seed exposure to mesophilic AD in the commercial biogas reactor in Wildau-Wentdorf (Germany) was described by Hahn et al. (2023). In brief, the continuously stirred reactor with an effective volume of 800 m³ was fed on maize silage, whole grain cereals and pig slurry. The reactor was operated at 44°C during seed exposure experiments from May until September 2016. The chemical characteristics of the substrate can be found in Table 1. Seeds were exposed to ADfull in six replicates for 3, 9, 18, and 35 days. Depending on the exposure time, one replicate contained 100, 200 or 300 seeds (Supplementary Table S1). After each exposure time, three replicates were tested for seed viability, while the other three were stored submerged in digestate for a further 89 days (Supplementary Figure S1).
2.3 Seed viability
2.3.1 Viability determination
Seed viability was determined based on measuring germination and metabolic activity. Germination tests took place on agar plates under sterile conditions. All seeds that did not germinate within 21 days were tested for their response to staining with 2,3,5-triphenyltetrazolium chloride. A seed was considered germinated if the radicle protruded at least 2 mm and metabolically active but dormant if the embryo – and endosperm, if relevant - was stained red.
2.3.2 Viability models
For each replicate, the proportion of viable seeds, V, was calculated by dividing the cumulative number of germinated seeds after 21 days in the germination test plus the number of dormant seeds by the total number of evaluated seeds. Seed viability as a function of exposure time in DS, AD or DS + AD, V(t), was modelled using non-linear regression (R package “drc” by Ritz et al. (2015), version 3.1.0). Models were fit species-wise setting treatment as a grouping variable. The data type was “binomial” and the total number of evaluated seeds was set as weights. The model fit was evaluated by a Chi2-test and visually. No model was fitted if all or almost all seeds had lost viability within the shortest exposure time. With one exception, log-logistic models with a lower limit at zero were fitted (Equation 1).
With V(t), proportion of viable seeds as a function of the time of exposure (t); Vmax, maximum proportion of viable seeds (upper asymptote); SLP, parameter proportional to the slope of V(t) in the inflection point; MIT (median inactivation time), the time after which V(t) reaches 50% of Vmax.
For M. sylvestris, log-logistic models did not provide a good fit when treated in DS alone. Therefore, Brain-Cousens modification of log-logistic models was applied (Ritz and Streibig, 2016; Equation 2).
With V(t), proportion of viable seeds as a function of the time of exposure (t); Vmax, maximum proportion of viable seeds (upper asymptote); H, size of the hormesis effect, i.e., stimulation of viability at t close to zero; SLP, parameter changing the slope of the model curve; E, parameter shifting and stretching the model curve.
2.3.3 Seed-killing efficacies and decimal reduction times
In order to compare the seed-killing effect of the different treatments, viability models were used to determine seed-killing efficacies (SKEs) and decimal reduction times (DRTs). The SKEs indicate the percentage of initial viability lost after a certain number of days of treatment (Equation 3).
With SKE, seed-killing efficacy; V, proportion of viable seeds either according to the viability models or, if no model could be fitted, according to measured values; x was 84 days, 1 day and 36 days for DS, lab-scale AD, and full-scale AD, respectively.
The DRTs indicate the exposure time required to reduce seed viability to 10% of the initial viability. DRTs were estimated using the “drc”-built-in function ED (Ritz and Streibig, 2016).
All statistical analyses were carried out using the software environment R (version 4.3.1) (R Core Team, 2023). Further details on determining and modelling of seed viability can be found in Hahn et al. (2022).
3 Results
3.1 Untreated seeds in digestate storage
Seed viability of the different species responded differently to storage in digestate (Figure 1 according to Table 2). In seeds of NHS species, viability was clearly reduced with increasing duration of digestate storage (DS). In C. album and tomato, there was a lag phase before the onset of dying. C. album was the most resistant NHS species with a decline in viability only between 56 and 83 days in digestate. The shape of the viability curve differed between the varieties of tomato. For C. intybus, E. vulgare, and V. thapsus, it is unclear how quickly their seeds were killed because they were completely inactivated within the first 27 days of storage. The same is true for D. carota, although in this species individual seeds were still viable after 27 and after 83 days. For the HS species, responses of seed viability to DS were less pronounced than that of the NHS species, ranging within the values of the untreated controls (shaded areas in Figure 1). For M. albus, M. alcea-a, and A. theophrasti-a, a trend towards a decrease in seed viability with increasing storage time was evident. In contrast, for M. officinalis and M. sylvestris, there was a trend toward an increase in (observed) viability during DS: specifically, between 56 and 83 days for M. officinalis and between 0 and 27 days for M. sylvestris.
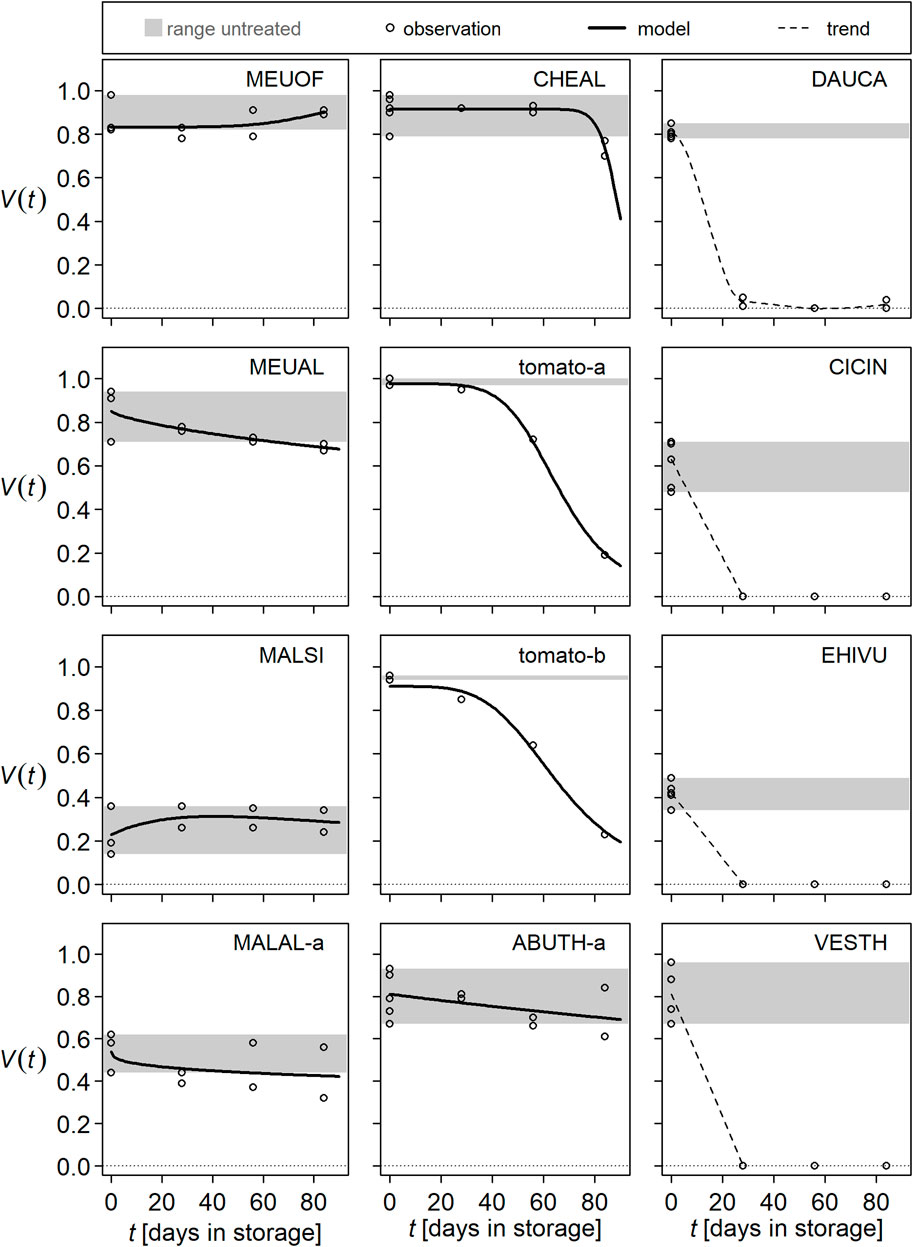
Figure 1. Seed survival of wild plant species and tomato during storage in digestate. Lines represent seed viability (V) as a function of storage time (t), and symbols represent observations containing at least 100 seeds each. The gray shaded area shows the range between minimum and maximum viability of untreated seeds. For clarity, panels were labeled with EPPO codes (https://www.eppo.int/RESOURCES/eppo_databases/eppo_codes) and arranged according to the survival probability of the respective species: Melilotus officinalis (MEUOF), Melilotus albus (MEUAL), Malva sylvestris (MALSI), Malva alcea seed lot a (MALAL-a), Chenopodium album (CHEAL), tomato variety a or b, Abutilon theophrasti seed lot a (ABUTH-a), Daucus carota (DAUCA), Cichorium intybus (CICIN), Echium vulgare (EHIVU), and Verbascum thapsus (VESTH).
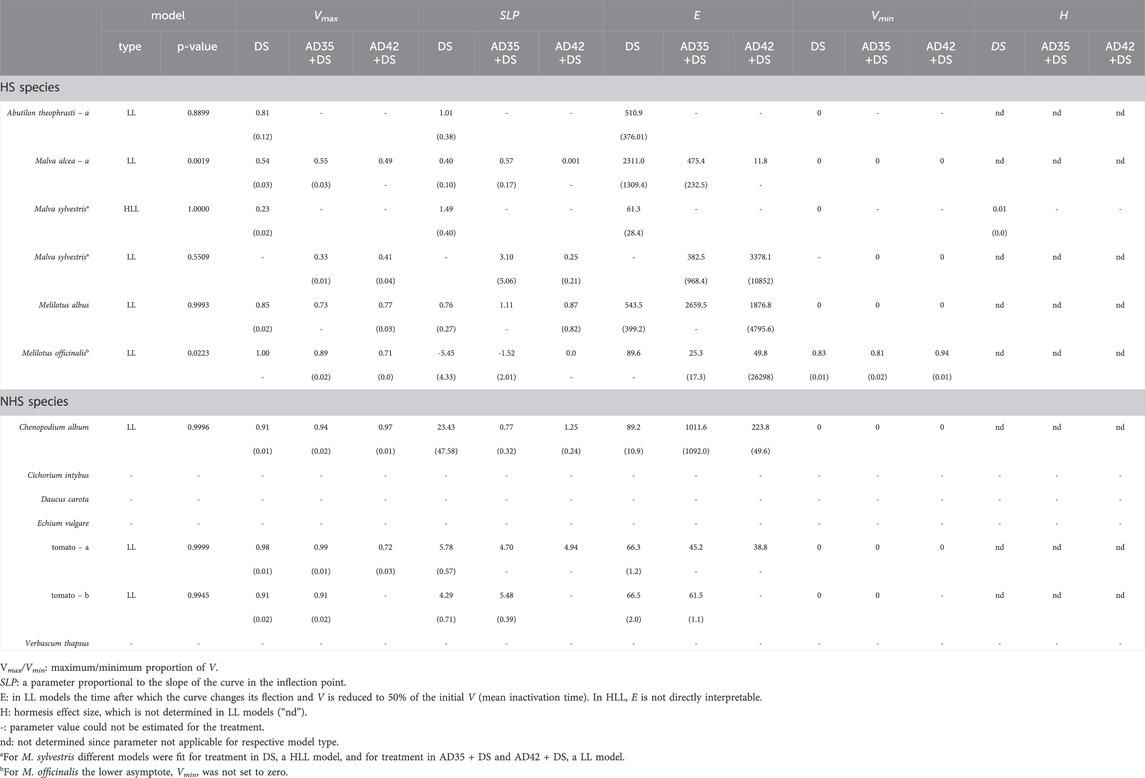
Table 2. Model type and fit (Chi2-test) and parameter estimates (standard errors in parentheses) obtained from the log-logistic (LL) and log-logistic models modified to capture hormesis (HLL) used to describe seed viability, V, during exposure to digestate storage (DS) and previous short-term anaerobic digestion at 35°C (AD35+DS) or 42°C (AD42 + DS) at lab-scale. Species are sorted according to their ability to produce hardseeded seeds (HS) or not (NHS).
3.2 Short-circuted seeds in digestate storage
3.2.1 Responses to short-circuited AD
The species responded very differently to short-circuited AD, i.e., 1 day in lab-scale reactors at 35°C (AD35) and 42°C (AD42), respectively (Figure 2). There was no clear distinction between HS and NHS species, instead their responses overlapped. Seed viability of the NHS species D. carota, C. intybus, E. vulgare, and V. thapsus was reduced at both temperatures compared to untreated controls (Figure 2, see shaded area and observations at t = 0). Inactivation was complete in AD42 but not in AD35, with the exception of V. thapsus. In tomato and A. theophrasti, viability was reduced only by AD42. Tendentially, i.e., within the range of untreated controls, viability decreased in M. albus, whereas it remained unaffected in M. officinalis, M. alcea, and C. album. Finally, M. sylvestris was a special case in which a 1-day AD treatment resulted in an increase in (observed) viability, especially in AD42.
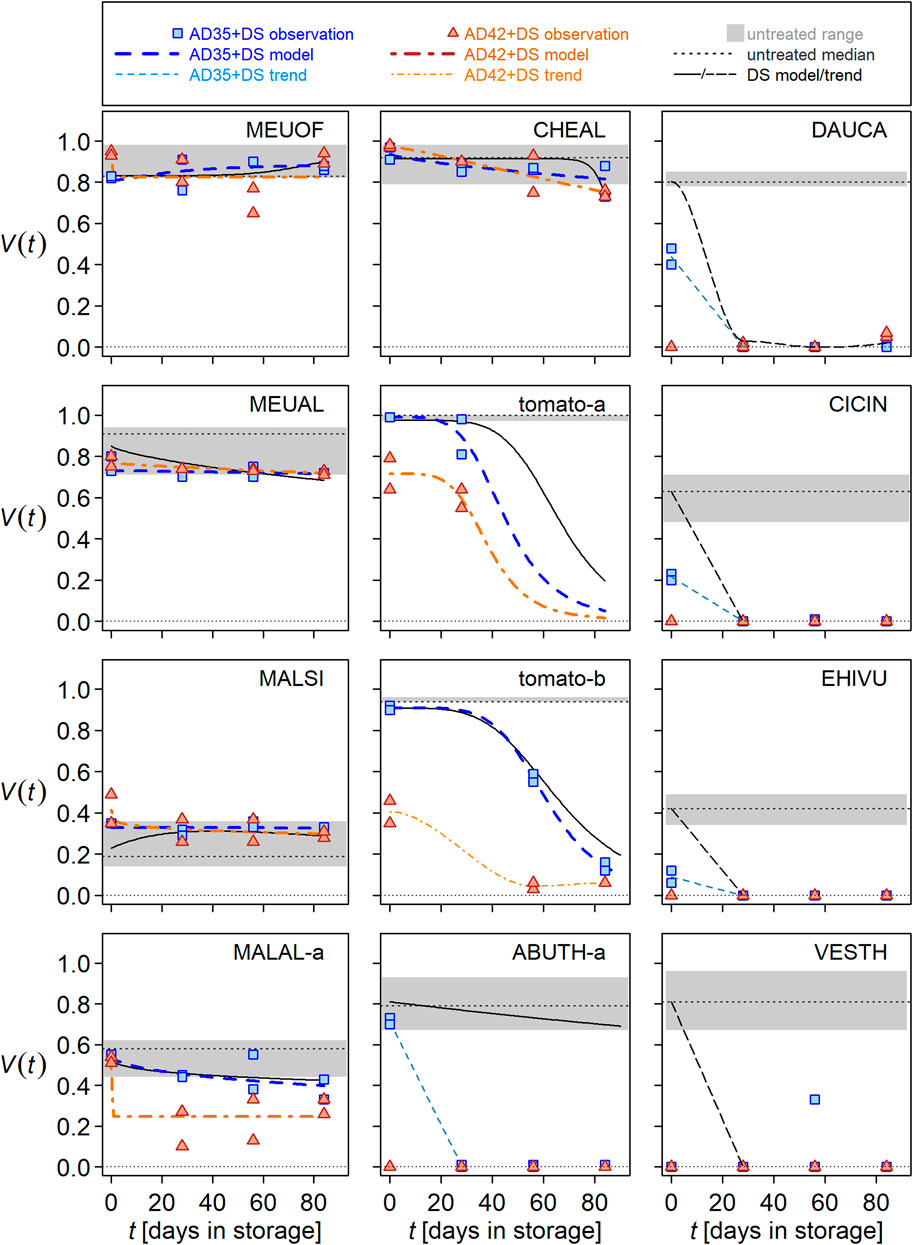
Figure 2. Seed survival of wild plant species and tomato during storage in digestate (DS) after 1-day treatment in anaerobic digestion at 35°C (AD35 + DS, blue lines and symbols) or at 42°C (AD42 + DS, orange lines and symbols). Lines represent seed viability (V) as a function of storage time (t) in the digestate, and symbols represent observations with at least 100 seeds each. Black lines represent average seed viability during DS as displayed in Figure 1. The grey horizontal dotted line indicates the median viability of untreated seeds. The gray shaded area shows the range between minimum and maximum viability of untreated seeds. Panels were labeled with EPPO codes (for explanation see Figure 1) and arranged according to the survival probability of the respective species.
3.2.2 Responses to storage in digestate after short-circuited AD
All seeds of A. theophrasti, D. carota, C. intybus, E. vulgare, and V. thapsus that had survived 1 day in AD35 were killed in the subsequent DS within 27 days. However, there was one outlier in V. thapsus (AD35 + DS) and some surviving seeds in D. carota (AD42 + DS). In both tomato varieties, some seeds survived the 83 days of DS, regardless of whether AD35 or AD42 preceded it. In both varieties, the shape of the viability curves was similar in all three treatments: seed viability decreased after a lag phase of different duration in DS, AD35 + DS and AD42 + DS. Tomato seed inactivation was greatest in AD42 + DS. The response of the two varieties was similar but not identical; for example, only in tomato-b were the curves of DS and AD35 + DS congruent (Figure 2). The extent of inactivation of M. alcea seed was considerably less than in tomato, and viability appeared to approach a minimal plateau rather than zero. Nevertheless, there were similarities with the tomato response: Inactivation was strongest at AD42 + DS, and as with tomato-b, the DS and AD35 + DS curves were congruent.
Seed of C. album, M. albus, and M. officinalis showed little response to post-AD storage in digestate. Viability values were consistently in the lower range of untreated controls (M. albus and M. officinalis) or decreased to these during storage (C. album). There was hardly any difference between treatments in DS, AD35 + DS and AD42+DS.
The viability of M. sylvestris remained consistently within the range of untreated controls during AD + DS. Remarkably, the viability of AD42 + DS- treated seeds was in the upper range or slightly higher than that of the controls, while the seeds treated solely with DS only reached this range after about 27 days (see Figure 1).
3.3 Seeds after full-scale AD in digestate storage
In full-scale AD at 44°C, the decrease in seed viability followed a log-logistic curve, with the greatest decrease during the first 3 days of exposure (Figure 3 according to Table 3). The only exception was M. sylvestris, which hardly lost viability during this treatment. In contrast, A. theophrasti-b was completely inactivated during the first 3 days of exposure. Compared to full-scale AD, additional DS had little no additional killing effect on the seed. Only C. album and M. albus tended to be inactivated slightly faster and stronger when stored for 89 days in digestate after full-scale AD (Figure 3).
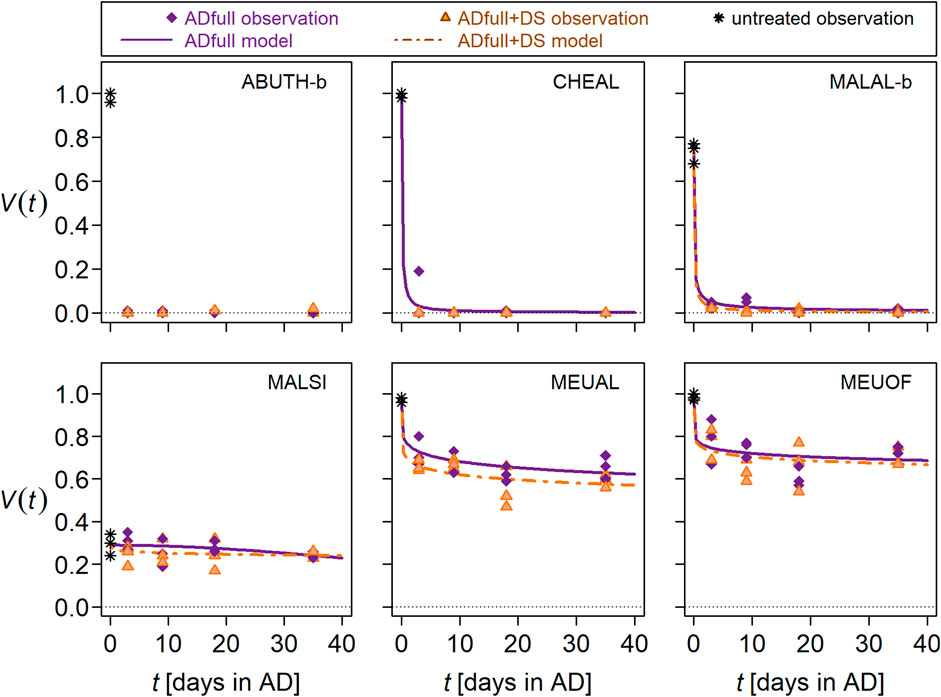
Figure 3. Seed viability (V) of selected wild plant species depending on exposure time (t) in anaerobic digestion at 44°C in a full-scale commercial biogas reactor (ADfull, purple lines and symbols) and after AD plus storage in digestate (DS, orange lines and symbols). Lines represent V as a function of t, and symbols represent observations containing at least 100 seeds each. Panels were labeled with EPPO codes (for explanation see Figure 1). Please note, that seed lots “b” was examined for ABUTH and MALAL.
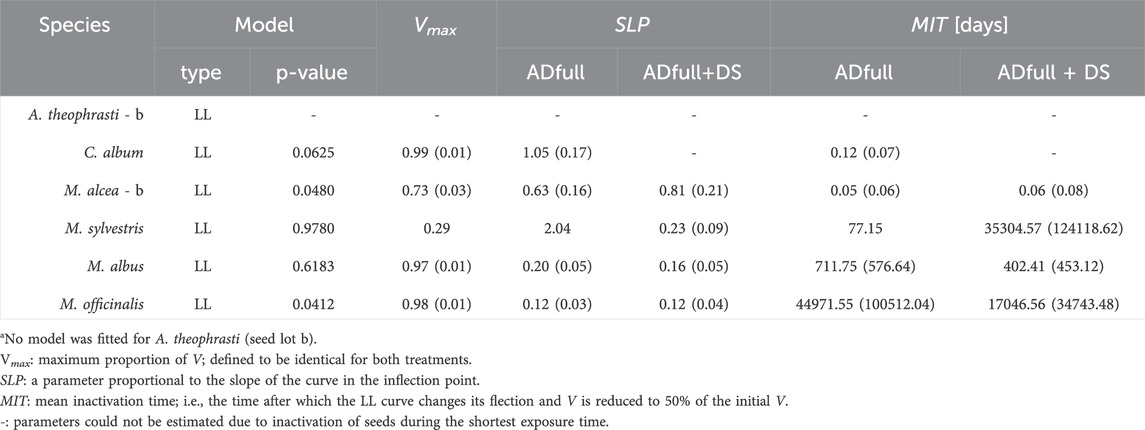
Table 3. Model type and fit (Chi2-test) and parameter estimates (standard errors in parentheses) obtained from the log-logistic (LL) models used to describe seed viability, V, during exposure to full-scale anaerobic digestion at 44°C (ADfull) or ADfull plus storage in digestate (DS). The lower asymptote was set to zero for all models.
3.4 Seed-killing effect of treatments
Seed-killing efficacies (SKEs) varied widely between species, seed lots and treatments. For the NHS species exposed only to lab-scale treatments, namely, tomato, D. carota, C. intybus, E. vulgare, V. thapsus, there was a trend to higher SKEs when short-circuited AD was combined with DS (Figure 4). SKE values ranged from 1% for tomato-a in AD35 to 100% in all treatments for V. thapsus. For species exposed to treatments in lab- and full-scale AD, only A. theophrasti, M. alcea and C. album were completely inactivated in some treatments (SKE = 100%). Further, two trends were observed: (1) SKEs seemed to be higher when full-scale AD was involved, and (2) responses were more variable in treatments involving short-circuited AD (Figure 5). In addition, SKEs were higher in A. theophrasti, M. alcea and M. albus when an AD treatment was combined with DS - just as in the NHS species mentioned above. For C. album, M. officinalis and M. sylvestris, negative SKEs were calculated for some lab-scale treatments, i.e., an increase in observed viability compared to the median of untreated control seeds. Maximum values for negative SKEs were −6%, −13%, and −121% for C. album, M. officinalis and M. sylvestris, respectively.
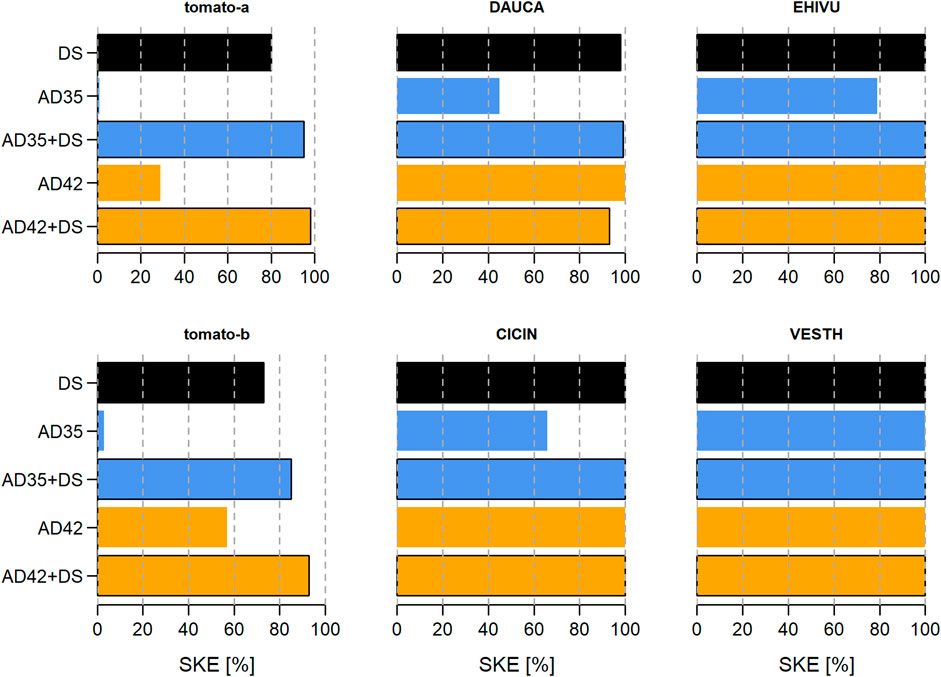
Figure 4. Seed-killing efficacies (SKEs) of digestate storage (DS) and short-circuited anaerobic digestion (AD) at lab-scale on the following species: tomato variety a or b, Daucus carota (DAUCA), Cichorium intybus (CICIN), Echium vulgare (EHIVU), and Verbascum thapsus (VESTH). Panels were arranged according to the survival probability of the respective species. Treatments were DS for 84 days (black bar), 1 day of AD at 35°C (AD35, blue bars) or 42°C (AD42, orange bars), and combinations of these (bars with black border).
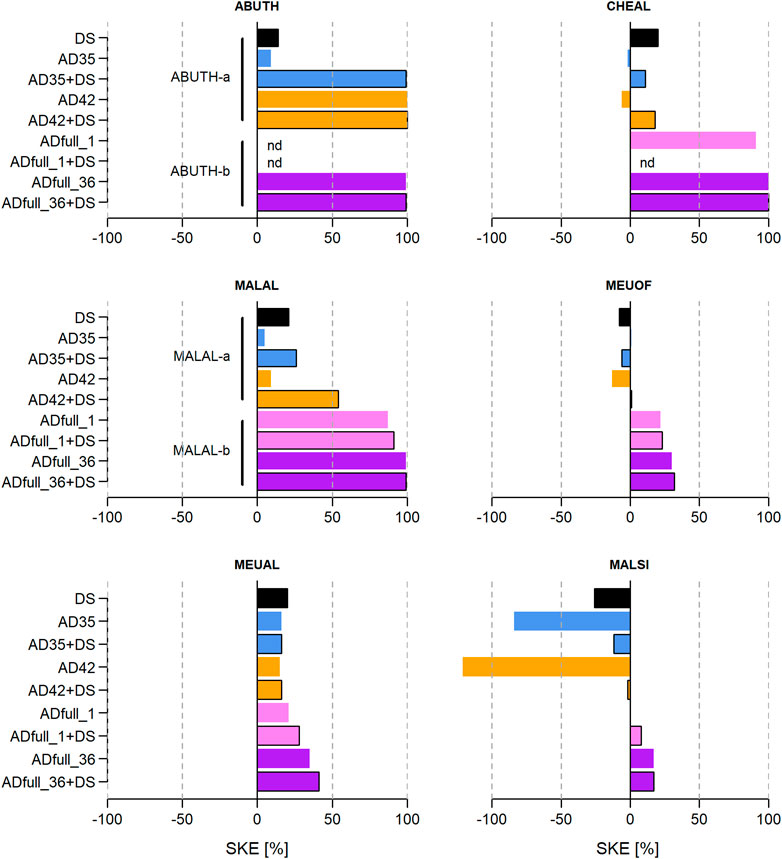
Figure 5. Seed-killing efficacies (SKEs) of digestate storage (DS), short-circuited anaerobic digestion at lab-scale (AD) and full-scale AD (ADfull) on the following species: Abutilon theophrasti seed lot a and b (ABUTH-a/-b), Malva alcea seed lot a and b (MALAL-a/-b), Melilotus albus (MEUAL), Chenopodium album (CHEAL), Melilotus officinalis (MEUOF), Malva sylvestris (MALSI). Panels were arranged according to the survival probability of the respective species. Treatments were DS for 84 days (black bar), 1 day of AD at 35°C (AD35, blue bar) or 42°C (AD42, orange bar), ADfull at 44°C for 1 day (ADfull_1, pink bar) or 36 days (ADfull_36, purple bar), and combinations of these (bars with black border). “nd” indicates that SKEs could not be determined. Negative SKEs indicate an increase in observed viability compared to the median of untreated control seeds.
The estimated decimal reduction times (DRTs) for DS with and without additional AD treatment could not be calculated for four of the NHS species (D. carota, C. intybus, E. vulgare, V. thapsus), as these died within the shortest exposure time (Supplementary Table S2). For the HS species, DRTs were mostly longer than 1 year. Interpretable DRTs could be calculated only for the tomatoes. They were 96 days in DS, 72 days in AD35 + DS, 60 days in AD42 + DS for tomato-a, and 111 days in DS, 92 days in AD35 + DS and < 56 days in AD42 + DS for tomato-b, respectively. There was a tendency towards shorter DRTs for short-circuited AD compared to DS alone.
4 Discussion
4.1 Digestate storage of untreated seeds
Digestate storage (DS) influenced the viability of the untreated seed. Quantity and quality of the responses varied greatly, particularly between hardseeded (HS) and non-hardseeded (NHS) species. While seed viability of the HS species was hardly affected, only one of the six NHS species, C. album, retained most of its viability after the 12 weeks in DS. The strong reduction in viability of NHS species is in line with findings of the only other study on seed survival in digestate by Strauß et al. (2012). They found that five of six NHS species investigated, namely Brassica napus (rapeseed, Brassicaceae), Linum usitatissimum (common flax, Linaceae), Sinapis alba (white mustard, Brassicaceae), Triticum vulgare (wheat, Poaceae) and Zea mays (maize, Poaceae) did no longer germinate after 36 days in DS. Only tomato seeds were still alive (Strauß et al., 2012). To complement this, we observed that seeds of tomato as well as C. album and D. carota could survive for up to 3 months in DS, i.e., three times longer than the period investigated by Strauß et al. (2012). Furthermore, seed viability of all HS species in this study was found to remain within the range of the untreated control throughout the 3 months in DS. This is in contrast to Strauß et al. (2012), who found Glycine max (soybean, Fabaceae) to cease germination after 10 days. However, the degree of HS, i.e., the percentage of non-germinating, dormant seeds with an intact impermeable layer in their seed coat, was much lower in the seed lot tested by Strauß et al. (2012) (96% germinating, 4% dormant or not metabolically active) than in those of the HS wild plant species investigated in this study (19% germinating, 53% dormant, and 28% not metabolically active; on average from Supplementary Table S3). Thus, HS could protect seeds from being inactivated in DS just as it does in AD, which after all is the source of the digestate (e.g., Westerman and Gerowitt, 2013).
Provided that a proportion of dormant seeds is actually present in the seed lot, HS species also tend to survive manure storage better than NHS species (Fruwirth, 1928 in Ehrenberg, 1935; Harmon and Keim, 1934; Özer, 1979; Wiese et al., 1998; Goldwasser et al., 2011; Yaacoby et al., 2015). Manure and digestate differ in their chemical properties (Horf et al., 2024). For instance, manures have lower pH values and ammonia concentrations as well as higher contents of total solids and total organic carbon, a higher carbon-to-nitrogen ratio, and a higher viscosity than digestates (Drosg et al., 2015). Given the lack of studies on seeds in DS, manure is suitable for comparisons as it is also a medium that undergoes an AD process prior to storage.
The duration of storage was a decisive factor for changes in seed viability and the success of seed inactivation in both digestate (Strauß et al., 2012; this study) and manure (e.g., Herzog, 1969; Nishida et al., 2002; Aper et al., 2014). The time required for seed inactivation during manure storage depended on the species and the storage conditions, but usually ranged from a few days to 2–3 months (e.g., Harmon and Keim, 1934; Herzog, 1969; Elema and Scheepens, 1992; Nishida et al., 2002; Yaacoby et al., 2015). In some cases, seeds were still viable after more than 3 (Rupende et al., 1998; Goldwasser et al., 2011; James et al., 2011) or even 12 months of manure storage (Mayer et al., 2000). The inactivation times determined for DS in this study are thus in a similar range to those for manure, both in terms of variation between the species and magnitude. It would be interesting to determine after what period of time the seeds of the more resistant species are completely killed and whether the viability curves differ between the two storage media.
The viability curves during exposure to DS mostly consisted of a lag phase of varying length followed by an exponential decline of varying steepness (Strauß et al., 2012; this study). In this study, however, two of the HS species deviated from this pattern, namely M. sylvestris and M. officinalis. Their seed viability did not decline but tended to increase during the 3 months of DS. For the same seed lots, this was also observed in mesophilic lab-scale AD (Hahn et al., 2022). In M. sylvestris, the viability curve in DS was very similar to that in AD at 42°C, where it was thought to be a hormetic response (Hahn et al., 2022). Hormetic responses are known from many different fields such as ecology and toxicology and describe the phenomenon that low doses of a stressor can have a stimulatory effect, whereas high doses cause inhibition (Calabrese and Baldwin, 2002; Kozumbo and Calabrese, 2019). In fact, hormesis-like responses of seed viability were not only described by Hahn et al. (2022), but also earlier for treatments in AD and water baths (e.g., Schrade et al., 2003; Tanke et al., 2019; Zhou et al., 2020). For the case of M. sylvestris in DS, the increase in seed viability continued until the end of the observation period, resulting in a negative seed-killing efficacy (SKE), although the measured values were within the range of the untreated seed. It should be noted that the relatively low initial viability of the M. sylvestris seed lot (23%) means that changes in only a few seeds could result in large percentage changes. In addition, calculation of the SKE relates the modeled value for 12 weeks of DS treatment to that of the untreated controls without considering their range. Therefore, even if there was indeed a hormetic response during DS treatment instead of the expected decline, the SKE values should be regarded as indicative and not definitive. Another aspect to be considered critically is the increase in the observed viability of M. officinalis between 8 and 12 weeks in DS. Here, too, the measured values were within the range of the untreated seed. For a hormetic response, the stimulation of seed viability had started very late, assuming that it is actually the duration of exposure that triggers hormesis. However, the response could also be based on an as yet unknown mechanism. To clarify how long HS seeds can actually survive DS and what makes them resistant to it, measurements would have to be continued in future studies until all seeds of a tested species have died.
Closely related to the question of how long seeds can survive in DS is which factors influence seed viability during DS. Temperature has been identified as one of the main factors reducing seed viability in manure storage and composting (e.g., Nishida et al., 2002; Larney and Blackshaw, 2003). It is not only the high temperatures that can arise, for example, from the self-heating of manure and compost piles, i.e., values between 50°C and 60°C and up to 80°C (e.g., Rupende et al., 1998; Wiese et al., 1998; Eckford et al., 2012), that are lethal for seeds, but also lower temperatures. Temperatures, similar to those in this study (18.4°C–28.4 °C) have been demonstrated to kill seeds in both manure storage (4, 12°C and 17°C, Elema and Scheepens, 1992) and manure composting (28°C–39°C, Tereshchuk and Lazauskas, 2002). The effect of these low temperatures on the seed is generally explained by their interaction with moisture (e.g., Ehrenberg, 1935; Eghball and Lesoing, 2000; Larney and Blackshaw, 2003; Zaller, 2007). This interaction triggers fatal germination and promotes microbial activity, which damages the seed coat, endosperm, or embryo (Shevkenek, 1934; Herzog, 1969; Özer, 1979; Rupende et al., 1998; Zaller, 2007). In addition, seed viability can be affected by numerous phytotoxins in the storage medium, such as acetic acid, uric acid, ammonia, and ethylene oxide (Wong et al., 1983; Rupende et al., 1998; Larney and Blackshaw, 2003; Kong et al., 2023). In this context, air exposure could also play a role, as Strauß et al. (2012) observed lower rates of seed survival in DS under anaerobic than under aerobic conditions. Which of the factors mentioned above caused the viability responses determined in this study remains to be investigated.
4.2 Digestate storage of anaerobically digested seeds
The 3-month storage of seeds in the digestate after mesophilic AD in the full-scale, commercial biogas reactor (ADfull + DS) did not kill significantly more seeds than ADfull alone. For a discussion of the effect of ADfull on seed survival, see Hahn et al. (2023). That seed-killing was not greater when combining ADfull and DS is in contrast to findings that the viability of weed seeds was reduced to a considerably greater extent when manure was stored for 3 months after passing the digestive tract of cattle or sheep (Atkeson et al., 1934; Özer, 1979; Rupende et al., 1998). However, it is unlikely that the seeds will remain viable indefinitely in the stored digestate. After all, DS alone had an influence on seed viability (see above). It is possible that it simply takes longer for seed viability to decline in DS than in AD. For example, Strauß et al. (2012) found that seeds survived about twice as long in DS as in AD (38°C). Such a comparison is not feasible for this study. What we can say, however, is that the SKEs of 84 days in DS were clearly smaller than those of 36 days in ADfull, e.g., by 43% in M. albus and 80% for C. album.
The slower reduction in seed viability in DS could be due to the fact that less damaging factors are at work in DS than in AD. For example, the temperature in DS was on average about 20°C degrees lower than in ADfull. With a similar temperature difference, Strauß et al. (2012) hypothesized that the microbial activity, which is supposed to lead to the decomposition of the seed coats and thus to the death of the seeds, was reduced in DS compared to AD. In addition, the mechanical forces of stirring the substrate in ADfull may have affected the seeds of some species - as indicated by the differences in seed survival between batch and stirred biogas reactors (Westerman and Gerowitt, 2013). An experiment comparing seed survival between batch AD and DS at the same temperature, time, and substrate could shed light on this.
A special case in which the seed-inactivating potential of DS could play an important role is the short-circuiting of AD in biogas reactors. The effects of short-circuited AD at 35°C (AD35) or 42°C (AD42) differed depending on the species and temperature. Roughly, the viability responses could be assigned to three differently susceptible groups: (1) reduced viability/dead at AD35 and dead at AD42 (C. intybus, D. carota, E. vulgare, V. thapsus), (2) no response at AD35 and reduced viability/dead at AD42 (tomato, A. theophrasti), and (3) no response/inactivation at either AD temperature (C. album, M. alcea, M. albus, M. officinalis, M. sylvestris). This grouping of seed susceptibility in short circuited AD was mostly repeated for AD + DS. In other words, species that were susceptible to AD were also susceptible to AD + DS. The intensity and speed of inactivation increased in seven of the eleven species examined in the following order: DS ≤ AD35 + DS < AD42+DS. Furthermore, HS seemed to play a role, as the group with the highest susceptibility (group 1) contained only NHS species, while the most resistant group 3 contained mainly HS species, including those that had lost less than 50% of their viability after 35 days ADfull and 3 months DS (M. sylvestris, M. albus and M. officinalis). This preliminary grouping is promising. However, in order to achieve the most complete seed kill after short-circuited AD, it is necessary to clarify what causes the numerous deviations from that grouping and which factors contribute to seed inactivation or resistance.
Regarding the susceptibility grouping, the role of HS in the ability to resist DS needs to be clarified. For example, the viability of M. alcea, which barely responded to AD35 and AD42, was reduced relatively strongly in AD + DS compared to that of the other HS species of group 3. Here, the fact that A. theophrasti belongs to group 2 and is rapidly and completely killed in AD + DS may indicate that both the degree and depth of dormancy play a role in the DS resistance of HS species, just as in AD (cf. Hahn et al., 2022). In this context, the observed increase in seed viability, i.e., the potential hormetic response (see Section 4.1), of M. sylvestris, which occurs in AD and DS but not in AD + DS, can also be investigated. That 3 months in DS had a smaller effect than 1 day in AD indicates that factors such as higher temperature being stronger in AD, possibly trigger hormesis. Moreover, viability models must be viewed critically. On the one hand regarding the interpretation of the measured values and on the other the suitability for extrapolation beyond the considered exposure period under. In the case of M. officinalis, for example, the model fitted best to the considered period, is not necessarily a biologically meaningful and causal model. For C. album the model assumed too much inactivation in DS compared to AD + DS, resulting in negative SKEs (−2% to −6%), which were, however, within the range of the untreated controls. In both cases, it would be helpful to extend the measurement period until all seeds are killed and remain dead, just as already pointed out in Section 4.1.
In summary, the question of whether DS can reduce the risk of weed spread after AD or short-circuited AD cannot be answered unequivocally. Many of the species examined were killed and thus, do not pose a risk. However, it must be noted that after AD in a commercial biogas plant with a retention time representative for Germany, seeds of three HS species were still viable even after adding 3 months of DS. After short-circuited AD (1 day) and subsequent 3-month DS, there were even four HS and two NHS species. One of the surviving species was C. album, which is a problem weed (Bajwa et al., 2019) and produces many seeds that have been shown to enter the harvested biomass (Westerman and Gerowitt, 2012). Considering that a proportion of 1%–10% of the substrate leaves biogas reactors short circuited (Turner et al., 1983; Baier et al., 2010), it seems very likely that the digestate will be contaminated with viable plant seeds - even if AD and DS are combined.
4.3 Conclusions and outlook
This study showed that DS could affect the viability of seeds of wild plant species and tomato, namely, the viability of untreated seeds and of seeds previously exposed to mesophilic AD in lab- or full-scale reactors. Both in DS and AD the extent of seed inactivation varied greatly between species and seed lots. HS species tended to be most resistant. In a preliminary classification of species according to their seed susceptibility to short-circuited AD and DS, the tomato, the indicator for phytohygiene in compost in Germany (Bundesministerium für Umwelt, Naturschutz, nukleare Sicherheit und Verbraucherschutz, 1998), was assigned to the middle group. Consequently, tomato is not suited as an indicator of seed mortality in DS as it would overestimate the survival of the group of very susceptible NHS species and underestimate that of the more resistant, predominantly HS species.
Duration of DS was an important factor modulating seed inactivation. However, responses were not limited to decline in seed viabilities but increases were observed as well. Future studies aiming to determine the factors inactivating seeds in DS should take three things into account. Firstly, the type and origin of the storage medium have a decisive influence (Harmon and Keim, 1934; Cudney et al., 1992). Therefore, we recommend specifying at least the chemical properties of the digestates, similar to this study. Ideally, a series of tests should be carried out with digestates that differ in the substrate but not in the storage conditions. Secondly, the responses of the seeds differ between the species, leading, for example, to different lethal temperatures (Larney and Blackshaw, 2003). However, the expression of the underlying response mechanisms such as metabolic adjustments of storage accumulation, changes in antioxidant enzyme activity, induction of phytohormones, expression of specific proteins and gene regulation (Kong et al., 2023) depend on the current physiological and morphological state (Herzog, 1969). Thus, both inter- and intra-species variation may occur. To handle this, we recommend providing information on the age of the seeds used and the proportion of germinating or dormant seeds. If effects of different digestates are to be compared identical seed lots should be used. Thirdly, multifactorial interactions can occur between the storage medium and the seeds. For example, there are reports of the suppression of pathogens by microbial consortia colonizing the seed, thereby increasing the survival rate of seeds in compost (Chen and Nelson, 2008). We therefore recommend interdisciplinary approaches involving biochemistry, microbiology, and seed biology to identify the most important factors for seed survival in DS.
On the basis of this study the question whether there is a risk that digestates contain viable (weed) seeds must be answered with “yes”. After 35 days of mesophilic AD and subsequent 3-month DS, 25%, 60% and 70% of the seed of the HS species M. sylvestris, M. albus and M. officinalis were still alive. Even more alarming is that some seeds from six of the eleven species tested were viable despite 3-month DS when AD lasted only 1 day, i.e., only for the duration of a short-circuit. That means, that the cultivation of seed-forming wild plants as energy crops, as practiced in Germany, but also in other parts of the world (e.g., Bomgardner, 2013; Sharma and Pant, 2019; Papamatthaiakis et al., 2021), could actually pose a weed risk. In order to estimate the probability of weed spread by contaminated digestates, we suggest to test the seed survival of the most resistant species in this study at full-instead of lab-scale DS over the usual 6 months in Germany. As the conditions in full-scale DS are more variable, e.g., in terms of temperature and microbial activity, more seeds could be killed than in lab-scale DS, just as full-scale AD kills seeds faster and to a greater extent than lab-scale AD (Hahn et al., 2023). In addition, freshly harvested seeds should be used instead of stored ones. The results of this test could be combined with studies on the quantity of seed introduced to AD in order to determine the number of viable seeds that enter the fields and the soil seed bank with the digestate. After all, as Ehrenberg (1935) emphasized as early as 1935, “weed management should not [only] be carried out on the manure heap, but also through appropriate cultivation, cropping, [and] weed control measures” (p. 93). Finally, it should be borne in mind that in the field the soil type, the application methods and climatic factors will modulate the effect of the digestate on the seed.
Data availability statement
The raw data supporting the conclusions of this article will be made available by the authors, without undue reservation.
Author contributions
JH: Conceptualization, Data curation, Formal Analysis, Investigation, Methodology, Validation, Visualization, Writing–original draft, Writing–review and editing. VP: Investigation, Methodology, Validation, Writing–original draft. BG: Conceptualization, Funding acquisition, Project administration, Resources, Supervision, Writing–review and editing. MH: Conceptualization, Data curation, Funding acquisition, Methodology, Project administration, Resources, Supervision, Validation, Writing–original draft, Writing–review and editing.
Funding
The author(s) declare that financial support was received for the research, authorship, and/or publication of this article. This study was funded by the German Federal Ministry of Food and Agriculture through the “Fachagentur für Nachwachsende Rohstoffe e.V.” (FNR) under grant numbers 22401114 and 22401513.
Acknowledgments
Our sincere thanks goes to Tilman Schlief for assisting VP in running the lab-scale reactors at the ATB and inserting/removing the plant seeds. We thank F. Klinkert (Klinkert Bioenergie GmbH, Erding, Germany) for allowing us to conduct experiments at their biogas plant in Wildau and B3 (BioenergieBeratungBornim GmbH, Potsdam, Germany), namely, Matthias Plöchl and Ingo Baumstark for monitoring the full-scale anaerobic digestion and insertion and removal of numerous of seed bags. We would like to express our gratitude to Paula R. Westerman for collecting and providing seeds of A. theophrasti-a and her valuable support during the project. Further, we thank Maren Knipping, Rosa Minderlen and Ophélie Rollin for their tireless assistance during the determination of seed viability and Diana Sicard for the necessary preparations. Last but not least, we appreciate the on-time transport of the anaerobically digested seeds by Markus Weinreich, Julia Schulz, Maria Lipski, and Anne Grauholz.
Conflict of interest
The authors declare that the research was conducted in the absence of any commercial or financial relationships that could be construed as a potential conflict of interest.
Publisher’s note
All claims expressed in this article are solely those of the authors and do not necessarily represent those of their affiliated organizations, or those of the publisher, the editors and the reviewers. Any product that may be evaluated in this article, or claim that may be made by its manufacturer, is not guaranteed or endorsed by the publisher.
Supplementary material
The Supplementary Material for this article can be found online at: https://www.frontiersin.org/articles/10.3389/fenrg.2024.1425213/full#supplementary-material
References
Aper, J., Cauwer, B. D, Roo, S. D., Lourenço, M., Fievez, V., Bulcke, R., et al. (2014). Seed germination and viability of herbicide resistant and susceptible Chenopodium album populations after ensiling, digestion by cattle and manure storage. Weed Res. 54, 169–177. doi:10.1111/wre.12063
Atkeson, F. W., Hulbert, H. W., and Warren, T. R. (1934). Effect of bovine digestion and of manure storage on the viability of weed seeds. J. Am. Soc. Agron. 26, 390–397. doi:10.2134/agronj1934.00021962002600050006x
Baier, U., Warthmann, R., and Schliess, K. (2010). Vergärungs-und Kompostierungsanlagen als Hygienebarrieren: Studie im Auftrag von: Amt für Abfall. Wasser, Energie and Luft Kt ZH (AWEL) and Bundesamt für Landwirtschaft (BLW).
Bajwa, A. A., Zulfiqar, U., Sadia, S., Bhowmik, P., and Chauhan, B. S. (2019). A global perspective on the biology, impact and management of Chenopodium album and Chenopodium murale: two troublesome agricultural and environmental weeds. Environ. Sci. Pollut. Res. Int. 26, 5357–5371. doi:10.1007/s11356-018-04104-y
Baskin, J. M., Baskin, C. C., and Li, X. (2000). Taxonomy, anatomy and evolution of physical dormancy in seeds. Plant Spec. Biol. 15, 139–152. doi:10.1046/j.1442-1984.2000.00034.x
Baute, K. A., Robinson, D. E., van Eerd, L. L., Edson, M., Sikkema, P. H., and Gilroyed, B. H. (2016). Survival of seeds from perennial biomass species during commercial-scale anaerobic digestion. Weed Res. 56, 258–266. doi:10.1111/wre.12202
Bomgardner, M. M. (2013). Chasing cheap feedstocks: biobased chemical companies seek RAW MATERIALS that can beat corn and natural gas. Chem. Eng. News 91, 11–15.
Bundesministerium für Ernährung und Landwirtschaft (2017). Verordnung über die Anwendung von Düngemitteln, Bodenhilfsstoffen, Kultursubstraten und Pflanzenhilfsmitteln nach den Grunds¨atzen der guten fachlichen Praxis beim Düngen vom 26.05.zuletzt geändert am 10. August 2021: DüV.
Bundesministerium für Umwelt, Naturschutz, nukleare Sicherheit und Verbraucherschutz (1998). Verordnung über die Verwertung von Bioabfällen auf landwirtschaftlich, forstwirtschaftlich und gärtnerisch genutzten Böden: Bioabfallverordnung - BioAbfV.
Calabrese, E. J., and Baldwin, L. A. (2002). Defining hormesis. Hum. Exp. Toxicol. 21, 91–97. doi:10.1191/0960327102ht217oa
Chen, M.-H., and Nelson, E. B. (2008). Seed-colonizing microbes from municipal biosolids compost suppress Pythium ultimum damping-off on different plant species. Phytopathology 98, 1012–1018. doi:10.1094/PHYTO-98-9-1012
Chen, Y., Zhao, Z., Zou, H., Yang, H., Sun, T., Li, M., et al. (2019). Digestive performance of sludge with different crop straws in mesophilic anaerobic digestion. Bioresour. Technol. 289, 121595. doi:10.1016/j.biortech.2019.121595
Cudney, D. W., Wright, S. D., Shultz, T. A., and Reints, J. S. (1992). Weed seed in dairy manure depends on collection site. Calif. Agric. 46, 31–32. doi:10.3733/ca.v046n03p31
Drosg, B., Fuchs, W., Al Seadi, T., Madsen, M., and Linke, B. (2015). Nutrient recovery by biogas digestate processing. Available at: https://www.ieabioenergy.com/wp-content/uploads/2015/08/NUTRIENT_RECOVERY_RZ_web2.pdf (Accessed April 01, 2024).
Düngebehörde, N. (2024). Anforderungen an die Lagerung von Wirtschaftsdüngern und Gärresten. Available at: https://www.duengebehoerde-niedersachsen.de/duengebehoerde/news/34881_Anforderungen_an_die_Lagerung_von_Wirtschaftsd%C3%BCngern_und_G%C3%A4rresten (Accessed March 16, 2024).
Eckford, R. E., Newman, J. C., Li, X., and Watson, P. R. (2012). Thermophilic anaerobic digestion of cattle manure reduces seed viability for four weed species. Int. J. Agric. Biol. Eng. 5, 71–75. doi:10.3965/j.ijabe.20120501.009
Eghball, B., and Lesoing, G. W. (2000). Viability of weed seeds following manure windrow composting. Compost Sci. Util. 8, 46–53. doi:10.1080/1065657X.2000.10701749
Ehrenberg, P. (1935). Zur Frage der Unkrautsamen im Stalldünger. Z. Pflanzenernaehr. Dueng. Bodenk. 39, 85–94. doi:10.1002/jpln.19350390108
Elema, A. G., and Scheepens, P. C. (1992). “Verspreiding van onkruiden en planteziekten met dierlijke mest: Een risico-analyse,” in Spreading of weeds and plant diseases with animal manure: a risk analysis. Netherlands: Lelystad.
Englund, O., Dimitriou, I., Dale, V. H., Kline, K. L., Mola-Yudego, B., Murphy, F., et al. (2020). Multifunctional perennial production systems for bioenergy: performance and progress. WIREs Energy Environ. 9, 1–24. doi:10.1002/wene.375
Fachagentur für Nachwachsende Rohstoffe e.V. (FNR) (2023). Gärprodukte-Aufbereitung. Available at: https://biogas.fnr.de/biogas-nutzung/gaerrestverwertung (Accessed July 19, 2023).
Fröschle, B., Heiermann, M., Lebuhn, M., Messelhäusser, U., and Plöchl, M. (2015). Hygiene and sanitation in biogas plants, in Biogas science and Technology. Editors G. Gübitz, A. Bauer, G. Bochmann, A. Gronauer, and S. Weiss (Cham: Springer International Publishing), 63–99.
Fruwirth, C. (1928). (Samen in stallmist). Fortschr Landw. Zeitschr Zentralbl gesamt Landw. 3, 832–833.
Goldwasser, Y., Miron, J., Sibony, M., Sorek, P., Yosef, E., and Rubin, B. (2011). Spread of weed seeds by cattly manure - is there a danger of infesting farmers fields with weeds?. in 3rd Int. Conf. Nov. Sustain. weed Manag. arid semi-arid Ecosyst. Huesca, Spain.
Guan, D., Zhao, J., Wang, Y., Fu, Z., Zhang, D., Zhang, H., et al. (2024). A critical review on sustainable management and resource utilization of digestate. Process Saf. Environ. Prot. 183, 339–354. doi:10.1016/j.psep.2024.01.029
Hahn, J., de Mol, F., and Müller, J. (2021). Ensiling reduces seed viability: implications for weed management. Front. Agron. 3, 1–13. doi:10.3389/fagro.2021.708851
Hahn, J., Westerman, P. R., de Mol, F., Heiermann, M., and Gerowitt, B. (2022). Viability of wildflower seeds after mesophilic anaerobic digestion in lab-scale biogas reactors. Front. Plant Sci. 13, 942346. doi:10.3389/fpls.2022.942346
Hahn, J., Westerman, P. R., Gerowitt, B., and Heiermann, M. (2023). Mesophilic, anaerobic digestion in a full-scale, commercial biogas reactor kills seeds more efficiently than lab-scale systems. Fermentation 9, 481. doi:10.3390/fermentation9050481
Harmon, G. W., and Keim, F. D. (1934). The percentage and viability of weed seeds recovered in the feces of farm animals and their longevity when buried in Manure. J. Am. Soc. Agron. 26, 762–767. doi:10.2134/agronj1934.00021962002600090010x
Hartung, C., Andrade, D., Dandikas, V., Eickenscheidt, T., Drösler, M., Zollfrank, C., et al. (2020). Suitability of paludiculture biomass as biogas substrate − biogas yield and long-term effects on anaerobic digestion. Renew. Energy 159, 64–71. doi:10.1016/j.renene.2020.05.156
Hassani, M., Vallius, E., Rasi, S., and Sormunen, K. (2021). Risk of invasive Lupinus polyphyllus seed survival in biomass treatment processes. Diversity 13, 264. doi:10.3390/d13060264
Haupt, R., Heinemann, C., Schmid, S. M., and Steinhoff-Wagner, J. (2021). Survey on storage, application and incorporation practices for organic fertilizers in Germany. J. Environ. Manage 296, 113380. doi:10.1016/j.jenvman.2021.113380
Heiermann, M., and Plogsties, V. (2018). Wildpflanzen-Samen in der Biogas-Prozesskette - Eintrags-und Überlebensrisiko unter dem Einfluss von Prozessparametern: Teilprojekt 2. (FKZ 22401513).
Herrmann, C., Idler, C., and Heiermann, M. (2016). Biogas crops grown in energy crop rotations: linking chemical composition and methane production characteristics. Bioresour. Technol. 206, 23–35. doi:10.1016/j.biortech.2016.01.058
Herzog, R. (1969). Einfluß einer Lagerung von Unkrautsamen in Stallmist auf deren Keimpotenz. Albr. 13, 97–102. doi:10.1080/03650346909412329
Holland, R. A., Eigenbrod, F., Muggeridge, A., Brown, G., Clarke, D., and Taylor, G. (2015). A synthesis of the ecosystem services impact of second generation bioenergy crop production. Renew. Sustain. Energy Rev. 46, 30–40. doi:10.1016/j.rser.2015.02.003
Horf, M., Gebbers, R., Olfs, H.-W., and Vogel, S. (2024). Determining nutrients, dry matter, and pH of liquid organic manures using visual and near-infrared spectrometry. Sci. Total Environ. 908, 168045. doi:10.1016/j.scitotenv.2023.168045
International Energy Agency (2020). Outlook for biogas and biomethane: prospects for organic growth. Available at: https://www.iea.org/reports/outlook-for-biogas-and-biomethane-prospects-for-organic-growth/an-introduction-to-biogas-and-biomethane (Accessed October 19, 2023).
James, T. K., Rahman, A., McGill, C. R., and Trivedi, P. D. (2011). Biology and survival of broom corn millet (Panicum miliaceum) seed. NZPP 64, 142–148. doi:10.30843/nzpp.2011.64.6013
Johansen, A., Nielsen, H. B., Hansen, C. M., Andreasen, C., Carlsgart, J., Hauggaard-Nielsen, H., et al. (2013). Survival of weed seeds and animal parasites as affected by anaerobic digestion at meso- and thermophilic conditions. Waste Manag. 33, 807–812. doi:10.1016/j.wasman.2012.11.001
Kong, Y., Wang, G., Tang, H., Yang, J., Yang, Y., Wang, J., et al. (2023). Multi-omics analysis provides insight into the phytotoxicity of chicken manure and cornstalk on seed germination. Sci. Total Environ. 861, 160611. doi:10.1016/j.scitotenv.2022.160611
Kovačić, Đ., Lončarić, Z., Jović, J., Samac, D., Popović, B., and Tišma, M. (2022). Digestate management and processing practices: a review. Appl. Sci. 12, 9216–9235. doi:10.3390/app12189216
Kozumbo, W. J., and Calabrese, E. J. (2019). Two decades (1998-2018) of research Progress on Hormesis: advancing biological understanding and enabling novel applications. J. Cell. Commun. Signal 13, 273–275. doi:10.1007/s12079-019-00517-7
Larney, F. J., and Blackshaw, R. E. (2003). Weed seed viability in composted beef cattle feedlot manure. J. Environ. Qual. 32, 1105–1113. doi:10.2134/jeq2003.1105
Magrí, A. (2018). Research trends on nutrient management from digestates assessed using a bibliometric approach. Front. Sustain. Food Syst. 2. doi:10.3389/fsufs.2018.00040
Mayer, F., Albrecht, H., and Pfadenhauer, J. (2000). The influence of digestion and storage in silage and organic manure on the germinability of six wees species (Papaver argemone, P. dubium, Legousia speculum-veneris; Centaurea cyanus, Spergula arvensis, Trifolium arvense), in Ergebnisse der 20. Deutschen Arbeitsbesprechung über Fragen der Unkrautbiologie und -bekämpfung vom 14. bis 16. März 2000 in Stuttgart-Hohenheim. Editors H. U. Haas, and K. Hurle, 47–54.
Nesse, A. S., Aanrud, S. G., Lyche, J. L., Sogn, T. A., and Kallenborn, R. (2022). Confirming the presence of selected antibiotics and steroids in Norwegian biogas digestate. Environ. Sci. Pollut. Res. Int. 29, 86595–86605. doi:10.1007/s11356-022-21479-1
Nishida, T., Kurokawa, S., Yoshimura, Y., Watanabe, O., Shibata, S., and Kitahara, N. (2002). Effect of temperature and retention time in cattle slurry on weed seed viability. Jpn. J. Grassl. Sci. 48, 340–345. doi:10.14941/grass.48.340_1
Özer, Z. (1979). Über die Beeinflussung der Keimfähigkeit der Samen mancher Grundlandpflanzen beim Durchgang durch den Verdauungstrakt des Schafes und nach Mistgärung. Weed Res. 19, 247–254. doi:10.1111/j.1365-3180.1979.tb01534.x
Papamatthaiakis, N., Laine, A., Haapala, A., Ikonen, R., Kuittinen, S., Pappinen, A., et al. (2021). New energy crop alternatives for Northern Europe: yield, chemical and physical properties of Giant knotweed (Fallopia sachalinensis var. ‘Igniscum’) and Virginia mallow (Sida hermaphrodita). Fuel 304, 121349. doi:10.1016/j.fuel.2021.121349
Pavičić, J., Novak Mavar, K., Brkić, V., and Simon, K. (2022). Biogas and biomethane production and usage: Technology development, advantages and challenges in Europe. Energies 15, 2940. doi:10.3390/en15082940
Piltz, J. W., Stanton, R. A., and Wu, H. (2017). Effect of ensiling and in sacco digestion on the viability of seeds of selected weed species. Weed Res. 57, 382–389. doi:10.1111/wre.12269
R Core Team (2023). R: a language and environment for statistical computing. Vienna, Austria: R Foundation for Statistical Computing.
Ritz, C., Baty, F., Streibig, J. C., and Gerhard, D. (2015). Dose-response analysis using R. PLoS One 10, e0146021. doi:10.1371/journal.pone.0146021
Rupende, E., Chivinge, O. A., and Mariga, I. K. (1998). Effect of storage time on weed seedling emergence and nutrient release in cattle manure. Exp. Agric. 34, 277–285. doi:10.1017/s0014479798343057
Šarapatka, B., Holub, M., and Lhotská, M. (1993). The effect of farmyard manure anaerobic treatment on weed seed viability. Biol. Agric. Hortic. 10, 1–8. doi:10.1080/01448765.1993.9754646
Schneider, N., and Gerber, M. (2020). Rheological properties of digestate from agricultural biogas plants: an overview of measurement techniques and influencing factors. Renew. Sustain. Energy Rev. 121, 109709–109719. doi:10.1016/j.rser.2020.109709
Schrade, S., Oechsner, H., Pekrun, C., and Claupein, W. (2003). Einfluss des Biogasprozesses auf die Keimfähigkeit von Samen. Landtechnik 58, 90–91. doi:10.15150/lt.2003.1404
Schulz, V. S., Schumann, C., Weisenburger, S., Müller-Lindenlauf, M., Stolzenburg, K., and Möller, K. (2020). Row-intercropping maize (Zea mays L.) with biodiversity-enhancing flowering-partners—effect on plant growth, silage yield, and composition of harvest material. Agric 10, 524. doi:10.3390/agriculture10110524
Sharma, V., and Pant, S. (2019). Weed as underutilized bio-resource and management tool: a comprehensive review. Waste Biomass Valor 10, 1795–1810. doi:10.1007/s12649-018-0212-2
Shevkenek, W. (1934) Viability of weed seeds in manure and silage. Masterthesis Saskatchewan. Canada: University of Saskatchewan.
Strauß, G., Kaplan, T., and Jacobi, T. (2012). Keimfähigkeit von Samen verschiedener (gentechnisch veränderter) Nutzpflanzen in Abhängigkeit von Prozessparametern und Verweildauer in einer Biogasanlage. J. Verbr. Leb. 7, 19–25. doi:10.1007/s00003-011-0756-6
Tanke, A., Müller, J., and de Mol, F. (2019). Seed viability of heracleum mantegazzianum (apiaceae) is quickly reduced at temperatures prevailing in biogas plants. Agronomy 9, 332. doi:10.3390/agronomy9060332
Tereshchuk, V., and Lazauskas, P.-A. (2002). Weed control in manure by composting. Zeit. Pfla. Pfla., 647–651.
Tompkins, D. K., Chaw, D., and Abiola, A. T. (1998). Effect of windrow composting on weed seed germination and viability. Compost Sci. Util. 6, 30–34. doi:10.1080/1065657X.1998.10701906
Turner, J., Stafford, D. A., Hughes, D. E., and Clarkson, J. (1983). The reduction of three plant pathogens (Fusarium, corynebacterium and globodera) in anaerobic digesters. Agric. Wastes 6, 1–11. doi:10.1016/0141-4607(83)90002-1
Vollrath, B., Werner, A., Kretzer, D., Marzini, K., Illies, I., and Klemisch, M. (2016). Energetische Verwertung von kräuterreichen Ansaaten in der Agrarlandschaft - eine ökologische und wirtschaftliche Alternative bei der Biogasproduktion (Phase II): Schlussbericht.
von Cossel, M., and Lewandowski, I. (2016). Perennial wild plant mixtures for biomass production: impact of species composition dynamics on yield performance over a five-year cultivation period in southwest Germany. Eur. J. Agron. 79, 74–89. doi:10.1016/j.eja.2016.05.006
vTI (2009). Biogasmessprogramm II: 61 biogasanlagen im vergleich. Available at: https://edocs.tib.eu/files/e01fb10/62358767X.pdf (accessed March1, 2024).
Walsh, J. J., Jones, D. L., Edwards-Jones, G., and Williams, A. P. (2012). Replacing inorganic fertilizer with anaerobic digestate may maintain agricultural productivity at less environmental cost. J. Plant Nutr. Soil Sci. 175, 840–845. doi:10.1002/jpln.201200214
Wang, W., and Lee, D.-J. (2021). Valorization of anaerobic digestion digestate: a prospect review. Bioresour. Technol. 323, 124626. doi:10.1016/j.biortech.2020.124626
Ward, A. J., Hobbs, P. J., Holliman, P. J., and Jones, D. L. (2008). Optimisation of the anaerobic digestion of agricultural resources. Bioresour. Technol. 99, 7928–7940. doi:10.1016/j.biortech.2008.02.044
Westerman, P. R., and Gerowitt, B. (2012). The probability of maize biomass contamination with weed seeds. J. Plant Dis. Prot. 119, 68–73. doi:10.1007/bf03356422
Westerman, P. R., and Gerowitt, B. (2013). Weed seed survival during anaerobic digestion in biogas plants. Bot. Rev. 79, 281–316. doi:10.1007/s12229-013-9118-7
Westerman, P. R., Hildebrandt, F., and Gerowitt, B. (2012). Weed seed survival following ensiling and mesophilic anaerobic digestion in batch reactors. Weed Res. 52, 286–295. doi:10.1111/j.1365-3180.2012.00918.x
Wiese, A. F., Sweeten, J. M., Bean, B. W., Salisbury, C. D., and Chenault, E. W. (1998). High temperature composting of cattle feedlot manure kills weed seed. Appl. Engin Agric. 14, 377–380. doi:10.13031/2013.19399
Wong, M. H., Cheung, Y. H., and Cheung, C. L. (1983). The effects of ammonia and ethylene oxide in animal manure and sewage sludge on the seed germination and root elongation of Brassica parachinensis. Environ. Pollut. Ser. A 30, 109–123. doi:10.1016/0143-1471(83)90008-9
Yaacoby, T., Goldwasser, Y., Paporish, A., and Rubin, B. (2015). Germination of Phelipanche aegyptiaca and Cuscuta campestris seeds in composted farm manure. Crop Prot. 72, 76–82. doi:10.1016/j.cropro.2015.03.005
Zaller, J. G. (2007). Seed germination of the weed Rumex obtusifolius after on-farm conventional, biodynamic and vermicomposting of cattle manure. Ann. Appl. Biol. 151, 245–249. doi:10.1111/j.1744-7348.2007.00172.x
Keywords: exposure time, fertilizer, physical dormancy, short-circuiting, seed inactivation, sustainability, temperature, weed
Citation: Hahn J, Plogsties V, Gerowitt B and Heiermann M (2024) Survival of plant seeds in digestate storage—with and without prior anaerobic digestion. Front. Energy Res. 12:1425213. doi: 10.3389/fenrg.2024.1425213
Received: 29 April 2024; Accepted: 04 September 2024;
Published: 25 September 2024.
Edited by:
Cesar Ivan Torres, Arizona State University, United StatesReviewed by:
Sreenivas Rao Ravella, Epoch BioDesign Ltd., United KingdomSheikh Adil Edrisi, Thapar Institute of Engineering and Technology, India
Copyright © 2024 Hahn, Plogsties, Gerowitt and Heiermann. This is an open-access article distributed under the terms of the Creative Commons Attribution License (CC BY). The use, distribution or reproduction in other forums is permitted, provided the original author(s) and the copyright owner(s) are credited and that the original publication in this journal is cited, in accordance with accepted academic practice. No use, distribution or reproduction is permitted which does not comply with these terms.
*Correspondence: Juliane Hahn, dG8uanVsaWFuZS5oYWhuQHdlYi5kZQ==
†ORCID: Juliane Hahn, orcid.org/0000-0003-2393-0852; Monika Heiermanns, orcid.org/0000-0002-9854-2734