- 1Carbon Solutions, Saint Paul, MN, United States
- 2Department of Mathematics, Statistics, and Computer Science, University of Illinois at Chicago, Chicago, IL, United States
Introduction: Sedimentary basins are naturally porous and permeable subsurface formations that underlie approximately half of the United States. In addition to being targets for geologic CO2 storage, these resources could supply geothermal power: sedimentary basin geothermal heat can be extracted with water or CO2 and used to generate electricity. The geothermal power potential of these basins and the accompanying implication for geologic CO2 storage are, however, understudied.
Methods: Here, we use the Sequestration of CO2 Tool (SCO2TPRO) and the generalizable GEOthermal techno-economic simulator (genGEO) to address this gap by a) estimating the cost and capacity of sedimentary basin geothermal power plants across the United States and b) comparing those results to nationwide CO2 sequestration cost and storage potential estimates.
Results and discussion: We find that across the United States, using CO2 as a geothermal heat extraction fluid reduces the cost of sedimentary basin power compared to using water, and some of the lowest cost capacity occurs in locations not typically considered for their geothermal resources (e.g., Louisiana, South Dakota). Additionally, using CO2 effectively doubles the sedimentary basin geothermal resource base, equating to hundreds of gigawatts of new capacity, by enabling electricity generation in geologies that are otherwise (with water) too impermeable, too thin, too cold, or not deep enough. We find there is competition for the best sedimentary basin resources between water- and CO2-based power, but no overlap between the lowest-cost resources for CO2 storage and CO2-based power. In this way, our results suggest that deploying CO2-based power may increase the cost of water based systems (by using the best resources) and the cost of CO2 storage (by storing CO2 in locations that otherwise may not be targeted). As such, our findings demonstrate that determining the best role for sedimentary basins within the energy transition may require balancing tradeoffs between competing priorities.
1 Introduction
Dispatchable (i.e., “firm”) low-carbon power can provide substantial value to decarbonizing the electricity system (Sepulveda et al., 2018; Bistline and Blanford, 2020; Cole et al., 2021), which is an essential step towards economy-wide decarbonization (Bistline and Blanford, 2021; EPRI and GTI Energy, 2022). Geothermal power plants are one of the many technologies that could provide this service (Wongel and Caldeira, 2023): they can provide “firm” dispatchable power because they are comprised of similar components as coal or natural-gas power plants (e.g., turbines, compressors), but emit substantially less CO2 because they are driven by thermal energy from the subsurface. In contrast to burning fossil-fuels, the thermal energy is obtained by producing geothermally-heated fluids, conventionally water, from a well.
Broadly, there are three types of geothermal resources that could be used for electricity generation: 1) naturally faulted and fractured formations, 2) hot dry rock, and 3) sedimentary basins. Naturally faulted and fractured formations are the conventional resources used for geothermal power and are typically referred to as “hydrothermal” resources. While hydrothermal resources supply heat to nearly all commercial geothermal power plants, they are relatively rare, and discovering more of these resources is an active area of research (Williams et al., 2008; USDOE, 2019). In contrast, hot dry rock resources are not naturally faulted and fractured, and this otherwise unobtainable geothermal heat can thus only be accessed by artificially fracturing, or “enhancing” the impervious rock. As a result, hot dry rock resources are typically referred to as Enhanced Geothermal Systems (EGS) resources. The estimated potential of the EGS heat resources is enormous and finding ways to reduce the cost of drilling and hydraulic stimulation is an active area of research in this area (Beckers et al., 2014; USDOE, 2019; Aghahosseini and Breyer, 2020; Norbeck and Latimer, 2023). Lastly, sedimentary basins are naturally porous and permeable and do not require artificial stimulation like EGS resources. While these basins generally have lower temperatures, they are much more ubiquitous compared to hydrothermal resources. For example, at least one, if not multiple, sedimentary basins underly approximately half of the United States (USGS, 2022).
Despite these advantages, sedimentary basin geothermal power is understudied. For example, the 2019 United States Department of Energy GeoVision study excluded sedimentary basins when estimating the electricity generation potential of the United States (USDOE, 2019). The GeoVision report does not provide a justification for this exclusion, but the reason is likely because the temperatures of sedimentary basins are generally considered too low for cost-effective electricity generation: the study assumed that any resource with a temperature lower than 150°C was not hot enough to warrant consideration for electricity (USDOE, 2019). While other studies have found sedimentary basin resources with sufficient temperatures to support electricity generation (Banks and Harris, 2018; Brasnett et al., 2023), the general consensus is that the majority of thermal energy in these subsurface formations is at relatively low temperatures (Porro and Augustine, 2012).
The use of cut off temperatures for consideration for electricity generation is based on the assumption that water is used as the subsurface heat extraction fluid (i.e., “water sedimentary basin” or WSB power plants), and as a result, the use of cut off temperatures may not be appropriate when CO2 is used instead. Using CO2 as the heat extraction fluid for sedimentary basin power is typically referred to as CO2-Plume Geothermal (CPG), and our prior work has demonstrated that, compared to water, using CO2 results in more geothermal heat extraction and lower-cost electricity (Adams et al., 2015; Adams et al., 2021). As such, it is possible that CPG technology could “unlock” sedimentary basins that are conventionally too cold for electricity generation with water. But this potential that CPG may have for enabling sedimentary basin power compared to water has yet to be robustly studied using nationwide geospatial geologic data.
Our prior work, however, started a different geospatial comparison using geologic data from a single basin: CPG and geologic CO2 storage (Ogland-Hand et al., 2022). Sedimentary basins are the primary targets for geologic CO2 storage, which, in addition to firm low carbon power plants, is another technology that provides value to decarbonization (EPRI and GTI Energy, 2022). As such, there are potential mutual benefits between CPG and CO2 storage. For one, CPG was first introduced as an approach for offsetting costs of CO2 storage by creating an additional revenue stream via electricity sales (Randolph and Saar, 2011). Further, instead of drilling all new wells for a CPG plant (i.e., “greenfield” development), some costs of CPG could be offset by using wells previously drilled for geologic CO2 storage (i.e., “brownfield” development). Unfortunately, however, our prior work suggested that these mutual benefits may not hold true. For one, we found that there are geologic conditions that support low-cost CO2 storage but do not support CPG development, and that CPG may thus increase the cost of CO2 storage by requiring CO2 to be injected in more expensive locations than may otherwise be targeted. Additionally, we also found that the breakeven price of electricity required for CPG development was lower for greenfield development compared to brownfield development because comparatively fewer injection wells are needed for CO2 storage, and the increased power capacity from drilling more CPG wells outweighs the increased cost for more drilling. Overall, this prior study demonstrated that development of CPG power will likely have ramifications to geologic CO2 storage, and vice versa.
Collectively, our prior work and that of others suggests that there are multiple ways to use a sedimentary basin for decarbonization, but the tradeoffs and potential synergies between using a sedimentary basin for CO2 storage, CPG power generation, or water sedimentary basin (WSB) power generation across the country remains understudied. Here, for the first time, we present the geothermal electricity potential of sedimentary basins across the United States and the implications of this potential for CO2 storage. Additionally, our work is also novel because it considers the area required for CO2 storage and sedimentary basin power as part of the analysis. As recent work has demonstrated, there are likely to be many land-use trade-offs to consider when deploying clean-energy technologies (i.e., wind or solar power, transmission lines) at the scale required for economy-wide decarbonization (Bennett et al., 2023; Lopez et al., 2023; Patankar et al., 2023). As such, considering the areal implications of sedimentary basin development as part of this first study quantifying their potential may better inform decision-makers on how to deploy this underutilized resource base. Our methods and materials are described in Section 2; Results in Section 3; Discussion in Section 4; and Conclusion in Section 5. Table 1 provides an explanation for abbreviations commonly used throughout the manuscript.
2 Materials and methods
Our prior work has developed and applied the Sequestration of CO2 Tool (SCO2TPRO) to quantify the cost and CO2 storage potential of sedimentary basins (Middleton et al., 2020a; Middleton et al., 2020b; Ogland-Hand et al., 2023). SCO2TPRO is commercially available and is a coupled geologic database of sedimentary basin properties and a techno-economic tool that was originally developed to estimate the cost and capacity of CO2 storage. Most recently, we modified SCO2TPRO to also estimate the levelized cost of electricity (LCOE) of CPG using data generated using the generalizable GEOthermal techno-economic simulator (genGEO) (Adams et al., 2021; Ogland-Hand et al., 2022). Here, we continue to modify SCO2TPRO for sedimentary basin geothermal applications and then apply it to a nationwide database of sedimentary basin properties that we developed in prior work (Ogland-Hand et al., 2023). More details on these two steps are found in Sections 2.1, 2.2, respectively.
2.1 Modifications to SCO2TPRO
Here, we make two additional modifications to SCO2TPRO beyond our prior work (Ogland-Hand et al., 2022):
1. To calculate the LCOE for WSB power plants, we follow the same approach of using genGEO data within SCO2TPRO that we previously established for CPG (Ogland-Hand et al., 2022). As a result, for a given set of geologic conditions (i.e., reservoir depth, net thickness, permeability, porosity, and temperature), we use SCO2TPRO to estimate: 1) the cost and CO2 storage potential of geologic CO2 storage; 2) the cost and electricity generation potential of CPG; and 3) the cost and electricity generation potential of WSB.
2. We implement the genGEO cost model as an option within SCO2TPRO when estimating the cost of CO2 storage (Adams et al., 2021). As a result, when SCO2TPRO is run, the costs of all three approaches for using the sedimentary basin are calculated with the same cost assumptions.
2.2 Application of SCO2TPRO
To estimate the geospatial cost and capacity of sedimentary basin power across the country, we apply the modified version of SCO2TPRO to a nationwide database of sedimentary basin properties that we developed in our prior work for geologic CO2 storage (Ogland-Hand et al., 2023). The SCO2TPRO database includes reservoir permeability, porosity, net thickness, temperature, and depth on a 10 km × 10 km resolution for over 2 million km2 of sedimentary basins across the continental United States. For each grid cell in this database, SCO2TPRO uses genGEO data to estimate the power capacity of a 5-spot power plant with a 1 km2 footprint, and following our prior work (Ogland-Hand et al., 2022), we scale this estimate up to the 10 km × 10 km resolution by multiplying by: a) the number of CO2 injection wells also calculated with SCO2TPRO for brownfield CPG (CPG-BF) estimates; or b) 78.5 for greenfield CPG (CPG-GF) estimates. As originally described in our prior work (Ogland-Hand et al., 2022), we set the number of CPG power plants to be 78.5 in every 10 × 10 km grid cell because 1) given the methodology of SCO2TPRO, the CO2 plume area across all wells is 78.5% of the user-defined area, as a circle with a diameter equal to the side of a square will encompass 78.5% of the area of the square; and 2) the current CPG power plant design assumes a 1 km2/power plant footprint (Adams et al., 2021). For WSB power plants, we multiply the 5-spot power plant capacity by 100 because each grid cell has an area of 100 km2. The assumptions used within this analysis are described in Section 2.2.1.
2.2.1 Assumptions used within SCO2TPRO
Within SCO2TPRO we use the same site-level assumptions as in our prior work (Ogland-Hand et al., 2022) to match the assumptions embedded within the genGEO data. As originally described in our prior work (Ogland-Hand et al., 2022), these assumptions are: 1) a square well pattern; 2) CO2 injection well diameter of 0.41 m; 3) one CO2 injection well per site; 4) a maximum of 1 MtCO2/yr injected per site; 5) zero brine production wells per site; 6) one CO2 injection pump per well; 7) zero stratigraphic wells/site; 8) zero old oil and gas wells that must be plugged prior to CO2 injection; 9) zero old water drinking wells that need to be plugged prior to injection; 10) zero back-up CO2 injection wells drilled per site; 11) zero above-zone monitoring wells drilled per injection well; 12) one in-zone monitoring well drilled per injection well; and 13) zero drinking water monitoring wells drilled per injection well. The flowrates for CPG and WSB power plants are based on the optimization within genGEO and the maximum flowrate for CO2 storage sites in SCO2TPRO is limited to 1 MtCO2/yr, which is an accepted operational maximum for industrial CO2 injection wells (Middleton et al., 2020a).
Lastly, the capital recovery factor, the fraction of capital assumed for operation and maintenance (O&M), and the capacity factor (a.k.a., the “financing assumptions”) can change the LCOE of power plants by upwards of 40% (Adams et al., 2021). As a result, in this study, we continue to use the same three scenarios of financing assumptions as in our prior work (Ogland-Hand et al., 2022), shown in Table 2. As originally described in our prior work (Ogland-Hand et al., 2022), the LCOECCS scenario can be thought of as representative if the CPG plant owners receive a similar cost of debt as the CO2 storage operators receive for a CO2 capture and storage (CCS) project. The LCOEOrmat scenario uses the financing assumptions from Ormat, a major geothermal power plant company (Adams et al., 2021). As such, this scenario is representative of financing conditions of the geothermal power industry. Finally, the LCOELazard scenario uses financing assumptions used by Lazard when providing their annual LCOE reports that compare the cost of different electricity generation technologies to one another (Lazard, 2019).

Table 2. Financing scenario assumptions (Ogland-Hand et al., 2022).
3 Results
3.1 Nationwide cost and capacity of sedimentary basin geothermal power
Figure 1 shows the LCOE of sedimentary basin power across the United States for WSB power, CPG-GF power, and CPG-BF power. Comparing the WSB map to the two CPG maps demonstrates that CPG technology can expand the geothermal resource base. For example, most of the sedimentary basin resource base in Illinois, Indiana, and Wyoming have insufficient reservoir transmissivity or temperature to support power generation (i.e., colored in black) when water is used but these locations become useable (i.e., not black) when CO2 is used as the heat extraction fluid. At the same time, there are still many sedimentary basins that cannot support geothermal power generation (i.e., are colored black) using water or CO2. For example, in Appalachia, Michigan, or Florida. As such, while CPG can expand the resource base in locations like Illinois, Indiana, and Wyoming, locations like Appalachia, Michigan, or Florida simultaneously demonstrate that CPG cannot expand the geothermal resource base to any location within a sedimentary basin.
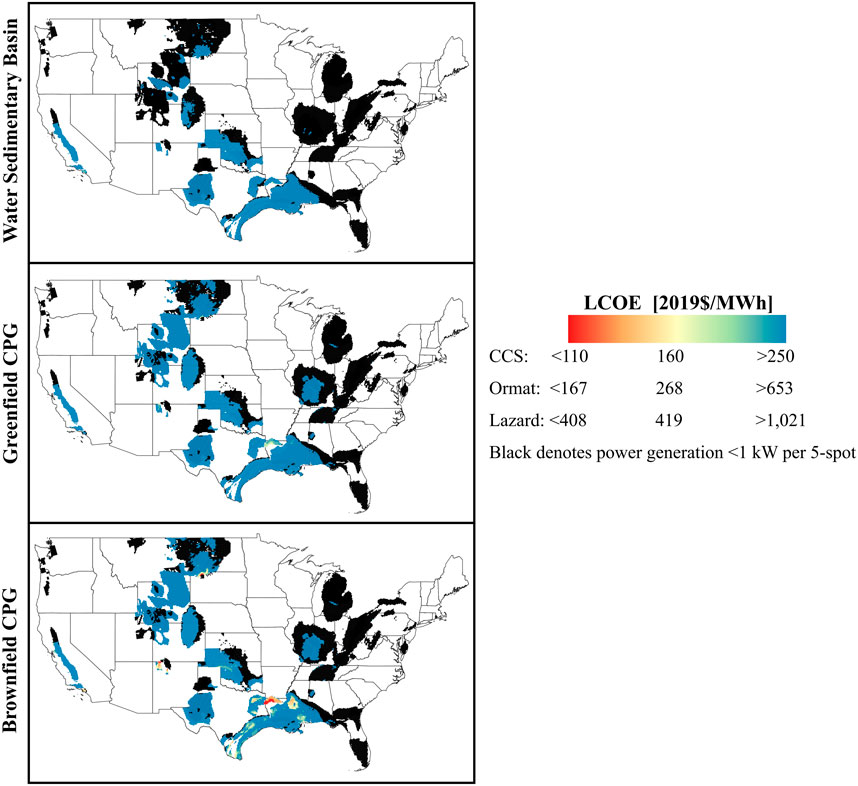
Figure 1. Geospatial LCOE of Sedimentary Basin Power. Please see our prior work for the geospatial cost of geologic CO2 storage across the country (Ogland-Hand et al., 2023).
While Figure 1 shows that CPG can “unlock” sedimentary basin resources that are otherwise unusable for WSB, the LCOE of the electricity is high in these “unlocked” locations. In contrast, there are locations amenable to power with WSB but in which the LCOE of CPG, and particularly CPG-BF, is much lower. Interestingly, these are largely locations not conventionally known for having geothermal resources amenable for electricity generation: Mississippi, Louisiana, New Mexico, and South Dakota [in addition to California and New Mexico, which have existing geothermal power plants (Robins et al., 2021)]. While the values of the LCOE are highly sensitive to the financing assumptions (Table 1), overall, Figure 1 suggests the primary “unlocking” capabilities of CPG is not that it expands the resource base to otherwise unusable locations, but that it decreases the cost of electricity in locations in which the cost would be too high for cost-competitive electricity generation (i.e., > $250/MWh).
Figure 2 compares the area and cost between WSB, CPG-GF, and CPG-BF as a function of cumulative power capacity. In both Figures 2A, B, the sedimentary basin resources are ordered from lowest to highest cost for each technology. Additionally, the grey bars in Figure 2A show the proportion of the lowest-cost WSB sedimentary basin resource also included in the lowest-cost CPG-GF sedimentary basin resource for a given power capacity.
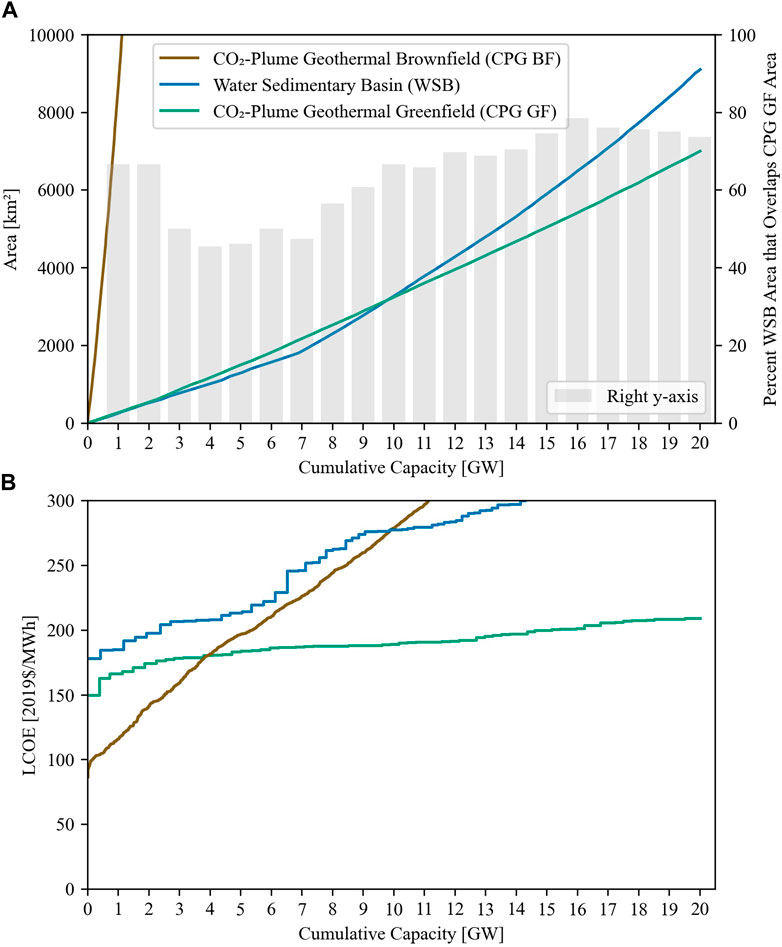
Figure 2. Area as a Function of the Cumulative Power Capacity (A) and the LCOE of Geothermal Power as a Function of the Cumulative Power Capacity (B). These results assume the “CCS” scenario financing assumptions (see Table 2). Supplementary Figure S1 contains a zoomed-out version of the supply curve shown in subplot (B).
Figure 2A demonstrates that CPG-BF requires more of the sedimentary basin resource to generate the same amount of power compared to CPG-GF. For example, to supply 1 GW of power capacity, CPG-GF requires ∼300 km2 of area while CPG-BF requires ∼8,700 km2 of area, a ×29 increase. This difference occurs because the power capacity of CPG-BF is limited to the number of wells drilled for CO2 storage (Ogland-Hand et al., 2022). In contrast, CPG-GF capacities are higher because of the additional wells drilled to generate electricity.
Figure 2A also suggests that CPG-GF and WSB require comparable area to provide a given power capacity, but that these two uses may compete for the lowest-cost sedimentary basin resources. For example, the lowest-cost 4 GW of sedimentary basin resources for WSB have 45.45% overlap with the lowest-cost 4 GW CPG-GF, and at 20 GW the overlap increases to 73.63%. Additionally, while CPG-BF requires much more area than CPG-GF to reach the same power capacity, Supplementary Figure S2 shows that the order of lowest-cost sedimentary basin resources is roughly the same for both CPG-GF and BF. Overall, these results suggest that there may be competition between WSB and CPG for sedimentary basin resources, all else equal.
Like Figures 1, 2B also demonstrates that CPG technology can reduce the cost of sedimentary basin power across the United States compared to using water. Unlike Figures 1, 2B suggests CPG could reduce the cost for substantial amounts of capacity. For example, CPG-GF power is always lower cost than WSB power and there are approximately 4 GW of CPG-BF capacity with lower cost than WSB power and CPG-GF power. For context, as of 2021, the total geothermal power capacity in the US was 3.6 GW (Robins et al., 2021). The reason the cost of CPG-BF power increases at a faster rate compared to CPG-GF and WSB is because it uses area less efficiently, as previously discussed with Figure 2A, so the lowest-cost resources supply less electricity. As such, in addition to showing the cost-saving potential of CPG technology, Figure 2B also suggests that choosing between CPG-BF and CPG-GF may require a tradeoff between efficient use of area and money.
3.2 Comparing different uses for sedimentary basis and implications for CO2 storage
Figure 3 compares the lowest-cost sedimentary basin resources for CO2 storage, WSB, and CPG by area. Figure 3A shows the cumulative potential for CO2 storage and the cumulative power capacity for geothermal as a function of area. It also shows the proportion of lowest-cost resources used for CO2 storage that are also used by either WSB or CPG geothermal power plants. Figure 3B shows the CO2 storage cost and the LCOE for WSB and CPG as a function of area. In both subplots, the sedimentary basin resources are ordered from lowest to highest cost for each technology.
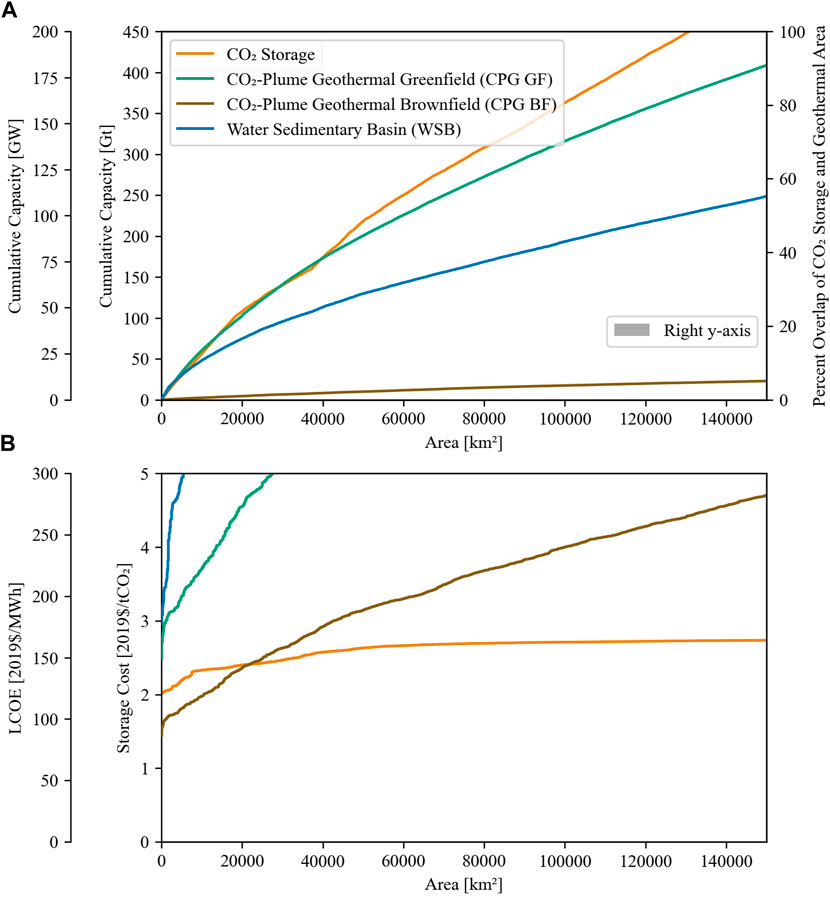
Figure 3. The Cumulative Power Capacity and CO2 Storage Potential as a Function of Area (A) and the CO2 Storage Cost and LCOE of Geothermal Power as a Function of Area (B). These results assume the “CCS” scenario financing assumptions (see Table 2).
The grey bars indicating the overlap in Figure 3A are always zero, which means that CO2 storage has no overlap in the lowest-cost (i.e., <$300/MWh) sedimentary basin resources with WSB or either type of CPG. For reference, Supplementary Figure S1 shows that across the country, there are approximately 30 GW, 180 GW, and 450 GW of CPG-BF capacity, WSB capacity, and CPG-GF capacity, respectively. As such, the capacities shown in Figure 3A represent about 40%, 61%, and 40% of these respective totals. This lack of overlap over this larger percent of the resource base suggests that CO2 storage and WSB will not compete for the lowest-cost geologic resources. However, it also means that the sedimentary basin resources that support lowest-cost CPG are different than the sedimentary basin resources that support lowest-cost CO2 storage.
Figure 4 shows the subsurface parameters for all sedimentary basins in the United States where each datapoint corresponds to a 100 km2 grid cell: Figure 4A shows the subsurface parameters of all sedimentary basin resources where only CO2 storage is viable; Figure 4B shows the parameters for the resources where CPG and CO2 storage are viable, but WSB is not; and Figure 4C shows the parameters for the resources where CPG, CO2 storage, and WSB are viable. Each subplot also includes the percentage of total sedimentary basin resource in each category. In subplots D, E, and F, the subsurface parameters for the lowest-cost 30,000 km2 of resources for CO2 Storage, CPG-BF, and WSB, respectively, are shown.
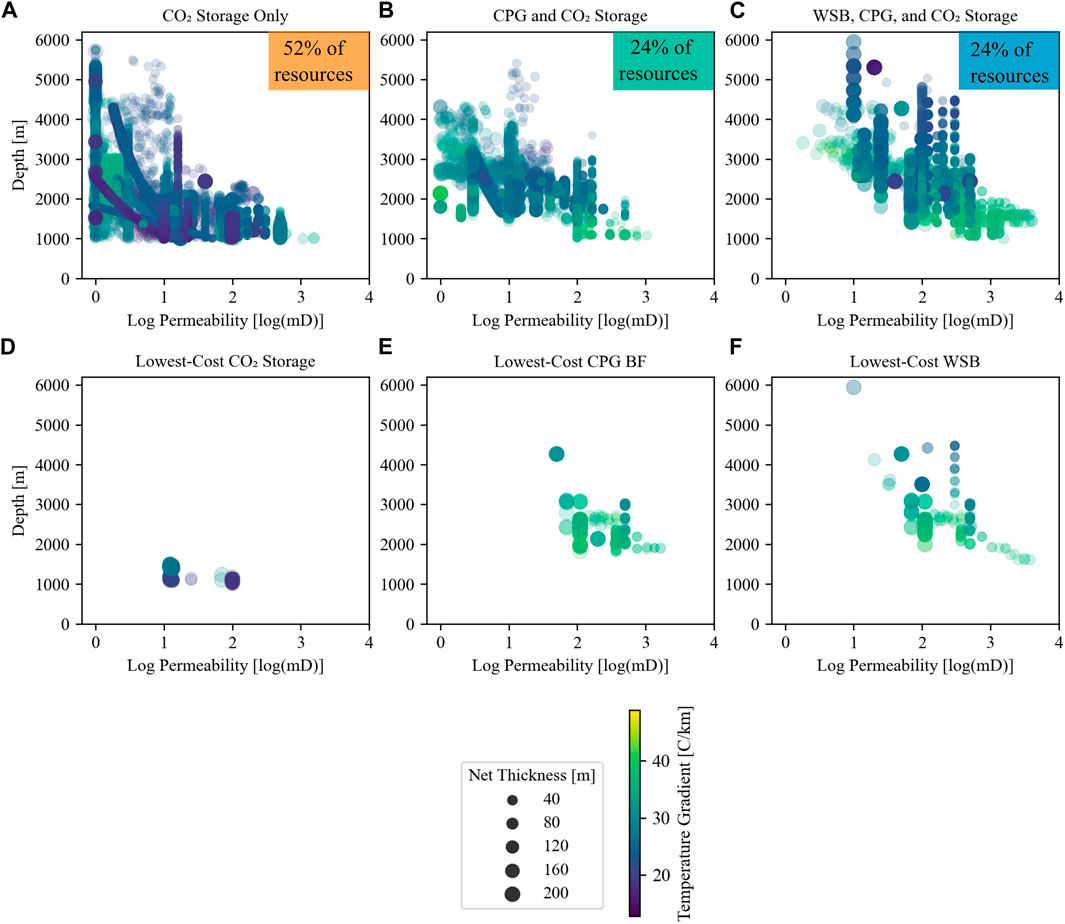
Figure 4. Geologic Properties of Sedimentary Basin Resources. (A) shows the subsurface parameters of all sedimentary basin resources where only CO2 storage is viable; (B) shows the parameters for the resources where CPG and CO2 storage are viable, but WSB is not; (C) shows the parameters for the resources where CPG, CO2 storage, and WSB are all viable; (D–F) show the lowest-cost 30,000 km2 of resources for CO2 storage, CPG-BF, and WSB, respectively.
Figure 4 suggests the reasoning why CPG “unlocks” portions of the sedimentary basin resource base, but not all areas are amenable to power generation, as discussed in Figure 1. First, Figure 4A shows that 52% of the sedimentary basin resource supports only CO2 storage and not sedimentary basin electricity generation. Compared to these geologic conditions, Figure 4B shows that the resources where CPG is viable require generally higher reservoir depth, temperature, and/or transmissivity, while Figure 4C shows that WSB requires these parameters to be even higher. Overall, comparing subplots A, B, and C suggests that electric power generation is possible in only about half of the resource base because of insufficient permeability, depth, thickness, or temperature in the remaining half. Further, comparing subplot B to C demonstrates that CPG can “unlock” marginal quality reservoirs for geothermal power generation (as discussed for Figure 1) by enabling electricity generation in reservoirs that are either too thin, too cold, not deep enough, or with insufficient permeability compared to WSB. As shown in Supplementary Figure S1, this additional 24% of “unlocked” resource equates to hundreds of gigawatts of additional electricity potential.
Figures 4D–F demonstrate that the geologies supporting the lowest-cost CO2 storage are different than the geologies supporting the lowest cost CPG or WSB power. For example, subplot D shows that the lowest-cost CO2 storage resources have low depth, low-to-mid permeabilities and temperatures, and high net thickness. As discussed in our prior work, these geologic preferences for low-cost geologic CO2 storage are driven largely because geologic CO2 storage injection rates are constrained to 1 MtCO2/yr within SCO2TPRO to reflect industrial-scale projects (Middleton et al., 2020a; Ogland-Hand et al., 2023). In contrast to these geologic preferences for low-cost CO2 storage, subplots E and F show that the lowest-cost geothermal resources have high permeability, thickness, depth, and temperature gradient. This difference explains the lack of overlap between geothermal power and CO2 storage in Figure 3A.
Figure 5 demonstrates the implications of prioritizing CPG development on WSB or CO2 storage development by comparing a) the supply curves for WSB with and without the lowest-cost 4 GW of CPG resources, and b) the supply curve for CO2 storage in any resources and in the lowest-cost 4 GW of CPG resources, respectively. Both subplots also include grey bars showing the percentage difference between the two supply curves.
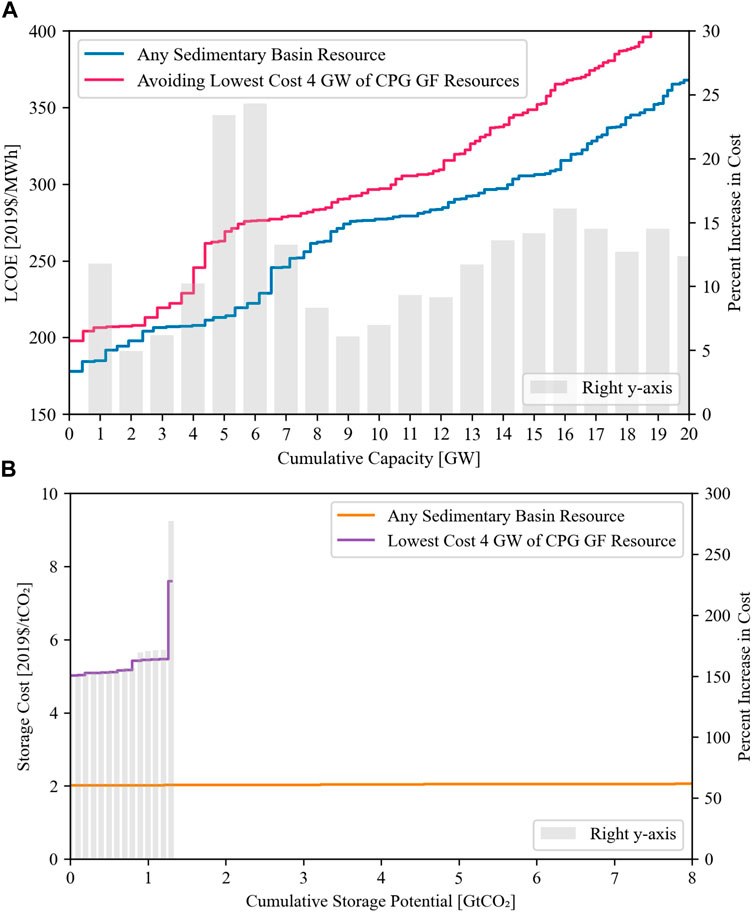
Figure 5. Comparison Supply Curve for WSB When Lowest Cost 4 GW of CPG GF Resources Are Avoided (A) and Comparison Supply Curve for CO2 Storage Only Considering the Lowest Cost 4 GW of CPG GF Resources (B). These results assume the “CCS” scenario financing assumptions (see Table 2).
Figure 5A suggests the implication of the ∼40%–∼70% overlap between lowest-cost resources for CPG and WSB (Figure 2A) is that avoiding the lowest-cost 4 GW of resources for CPG-GF will increase the LCOE of WSB by at least 5%. For example, the LCOE of the lowest-cost GW of WSB resource increases by about 14% when the resources best-suited for CPG are avoided. Further, avoiding the best CPG resources also causes the jump in cost to occur earlier, at 3–5 GW instead of 6–8 GW. In addition to making WSB at least 5% more expensive, Figure 5B suggests that the 0% overlap between lowest-cost locations for CPG and lowest-cost locations for CO2 storage (Figure 3A) means that intentionally sequestering CO2 in locations that are best for CPG will increase the cost of CO2 storage by at least 150%. Overall, Figure 5 demonstrates that there are likely to be trade-offs to consider when choosing which type of geothermal technology to deploy for a given resource.
4 Discussion
At the highest-level, our results indicate that sedimentary basin resources have potential for electricity generation. Thus, at a minimum, our study suggests that future research should include these resources within geothermal resource assessements. But our findings also suggest that determining the best role for sedimentary basins will likely require balancing tradeoffs between competing priorities (i.e., cost, areal requirements, decarbonization pathway). In this way, sedimentary basins are similar to other natural resources that may be used within the energy transition (e.g., sunlight, wind). As such, in addition to further quantifying this resource base (e.g., with resource assessments), our results also suggest that an important focus of future work could be studying how best to utilize it to reach decarbonization goals. Specifically, using energy system modeling to determine the optimal use of the sedimentary basin resource could be used in such future work if the optimal use is defined as that which has the greatest value to decarbonization.
Our study also suggests that the most important “unlocking” capability of using CO2 as the heat extraction fluid is not enabling power generation in locations where it is otherwise not possible, but rather that it reduces the cost of sedimentary basin power compared to using water. While CPG effectively doubles the sedimentary basin resource base that is amenable for power generation, the LCOE of CPG power is greater than $250/MWh (assuming aggressive “CCS” financing assumptions) in these “unlocked” locations. In contrast, in resources amenable to power generation with WSB plants, the LCOE of sedimentary basin power can substantially decrease when CO2 is used as the subsurface heat extraction fluid instead of water.
5 Conclusion
In this paper, we modify and apply the Sequestration of CO2 Tool (SCO2TPRO) to estimate the costs and capacities of CO2 storage and both water- and CO2-based geothermal power plants in sedimentary basins throughout the United States. To our knowledge, this is the first nationwide assessment of sedimentary basin geothermal resources for power generation of its kind. We find that:
1. Compared to sedimentary basin geothermal power plants that use water to extract heat (WSB), CO2-Plume Geothermal (CPG) technology can reduce the cost of generating geothermal electricity but may require more land. Across the United States, the cost of greenfield CPG power is always lower than that of WSB power (Figure 2). Additionally, there are 4 GW of brownfield CPG power capacity at lower costs than greenfield systems (Figure 2). Greenfield CPG development requires a similar amount of area as WSB development, but brownfield development requires around 30x more area (Figure 2).
2. CPG expands the portion of the sedimentary basin resource base that can be used to generate electricity by enabling power generation in geologies that are otherwise too thin, too cold, too impermeable, or not deep enough. While about 24% of sedimentary basin resources can be used to generate electricity through water-based geothermal, an additional 24% of resources are “unlocked” with CPG (Figure 4). Thus, CPG technology effectively doubles the useable resource base for sedimentary basin geothermal across the United States, which equates to hundreds of gigawatts of new power capacity (Supplementary Figure S1).
3. There is competition between water-based and CO2-based geothermal power systems for the best sedimentary basin resources, thus the cost of WSB increases when avoiding these lowest-cost resources for CPG. States with the best resources for sedimentary basin geothermal power generation include Louisana, Texas, New Mexico, North and South Dakota, and California (Figure 1). CPG is less expensive than WSB in these locations, and prioritizing CPG in these locations causes a 5%–25% increase in LCOE for WSB (Figure 5).
4. There is no overlap between the lowest-cost sedimentary basin resources for CO2 storage and for CPG, thus storing CO2 in resources that allow for lower-cost CPG increases the cost for CO2 storage. Across the United States, the resources that provide the lowest-cost CO2 storage are generally shallower, colder, and thicker than the resources that provide the lowest-cost CPG power (Figure 4). Prioritizing sedimentary basin resources for CO2 storage that are low-cost for CPG increases CO2 storage cost by ∼150% (Figure 5).
Data availability statement
The original contributions presented in the study are included in the article/Supplementary Material, further inquiries can be directed to the corresponding author.
Author contributions
EC: Data curation, Formal Analysis, Investigation, Visualization, Writing–original draft. JO-H: Writing–original draft, Data curation, Formal Analysis, Investigation, Visualization, Conceptualization, Methodology, Software, Validation. BA: Conceptualization, Methodology, Resources, Writing–review and editing. RM: Funding acquisition, Project administration, Resources, Writing–review and editing.
Funding
The author(s) declare that financial support was received for the research, authorship, and/or publication of this article. This material is based upon work supported by the U.S. Department of Energy, Small Business Innovations Research program under Award DE-SC0021570.
Acknowledgments
EC would also like to acknowledge funding from the NSF INMAS program. The content is solely the responsibility of the authors and does not necessarily represent the official views of the Department of Energy or the National Science Foundation.
Conflict of interest
Authors EC, JO-H, BA, and RM were employed by Carbon Solutions.
Publisher’s note
All claims expressed in this article are solely those of the authors and do not necessarily represent those of their affiliated organizations, or those of the publisher, the editors and the reviewers. Any product that may be evaluated in this article, or claim that may be made by its manufacturer, is not guaranteed or endorsed by the publisher.
Supplementary material
The Supplementary Material for this article can be found online at: https://www.frontiersin.org/articles/10.3389/fenrg.2024.1422285/full#supplementary-material
References
Adams, B., Ogland-Hand, J., M. Bielicki, J., Schädle, P., and Saar, M. (2021). Estimating the geothermal electricity generation potential of sedimentary basins using genGEO (the generalizable GEOthermal techno-economic simulator). ChemRxiv Prepr. doi:10.26434/chemrxiv.13514440.v1
Adams, B. M., Kuehn, T. H., Bielicki, J. M., Randolph, J. B., and Saar, M. O. (2015). A comparison of electric power output of CO2 plume geothermal (CPG) and brine geothermal systems for varying reservoir conditions. Appl. Energy 140, 365–377. doi:10.1016/j.apenergy.2014.11.043
Aghahosseini, A., and Breyer, C. (2020). From hot rock to useful energy: a global estimate of enhanced geothermal systems potential. Appl. Energy 279 (September), 115769. doi:10.1016/j.apenergy.2020.115769
Banks, J., and Harris, N. B. (2018). Geothermal potential of foreland basins: a case study from the western Canadian Sedimentary Basin. Geothermics 76 (September 2017), 74–92. doi:10.1016/j.geothermics.2018.06.004
Beckers, K. F., Lukawski, M. Z., Anderson, B. J., Moore, M. C., and Tester, J. W. (2014). Levelized costs of electricity and direct-use heat from enhanced geothermal systems. J. Renew. Sustain. Energy 6 (1). doi:10.1063/1.4865575
Bennett, J. A., Ogland-Hand, J. D., Middleton, E. J., Eidbo, J. B., Prorok, M., Ross, B., et al. (2023). The transmission ramifications of social and environmental siting considerations on wind energy deployment. Front. Energy Res. 10 (January), 1–15. doi:10.3389/fenrg.2022.1040957
Bistline, J. E. T., and Blanford, G. J. (2020). Value of technology in the U.S. Electric power sector: impacts of full portfolios and technological change on the costs of meeting decarbonization goals. Energy Econ. 86, 104694. doi:10.1016/j.eneco.2020.104694
Bistline, J. E. T., and Blanford, G. J. (2021). The role of the power sector in net-zero energy systems. Energy Clim. Change 2, 100045. doi:10.1016/j.egycc.2021.100045
Brasnett, G., Hickson, C., and Shor, R. J. (2023). “Mapping techno-economic feasibility of geothermal resources in alberta, Canada,” in Proceedings of the 48th workshop on geothermal reservoir engineering. Available at: https://pangea.stanford.edu/ERE/db/GeoConf/papers/SGW/2023/Brasnett.pdf.
Cole, W. J., Greer, D., Denholm, P., Frazier, A. W., Machen, S., Mai, T., et al. (2021). Quantifying the challenge of reaching a 100% renewable energy power system for the United States. Joule 5, 1732–1748. doi:10.1016/j.joule.2021.05.011
EPRI and GTI Energy (2022). Net-zero 2050: U.S. Economy-wide deep decarbonization scenario analysis. Available at: https://lcri-netzero.epri.com/.
Lazard (2019). Levelized cost of energy analysis - version 13.0. Available at: https://www.lazard.com/media/451086/lazards-levelized-cost-of-energy-version-130-vf.pdf.
Lopez, A., Cole, W., Sergi, B., Levine, A., Carey, J., Mangan, C., et al. (2023). Impact of siting ordinances on land availability for wind and solar development. Nat. Energy 8, 1034–1043. doi:10.1038/s41560-023-01319-3
Middleton, R. S., Chen, B., Harp, D. R., Kammer, R. M., Ogland-Hand, J. D., Bielicki, J. M., et al. (2020a). Great SCOT! Rapid tool for carbon sequestration science, engineering, and economics. Appl. Comput. Geosciences 7 (May), 100035. doi:10.1016/j.acags.2020.100035
Middleton, R. S., Ogland-Hand, J. D., Chen, B., Bielicki, J. M., Ellett, K. M., Harp, D. R., et al. (2020b). Identifying geologic characteristics and operational decisions to meet global carbon sequestration goals. Energy and Environ. Sci. 13, 5000–5016. doi:10.1039/d0ee02488k
Norbeck, J. H., and Latimer, T. M. (2023). Commercial-scale demonstration of a first-of-a-kind enhanced geothermal system. EarthArXiv. doi:10.31223/X52X0B
Ogland-Hand, J., Adams, B. M., Bennett, J. A., and Middleton, R. S. (2022). A geospatial cost comparison of CO2-plume geothermal (CPG) power and geologic CO2 storage. Front. Energy Res. 10. doi:10.3389/fenrg.2022.855120
Ogland-Hand, J., Cox, K. J., Adams, B. M., Bennett, J. A., Johnson, P. J., Middleton, E. J., et al. (2023). How to net-zero America: nationwide cost and capacity estimates for geologic CO2 storage. Eng. Arch. doi:10.31224/3293
Patankar, N., Sarkela-Basset, X., Schivley, G., Leslie, E., and Jenkins, J. (2023). Land use trade-offs in decarbonization of electricity generation in the American west. Energy Clim. Change 4 (February), 100107. doi:10.1016/j.egycc.2023.100107
Porro, C., and Augustine, C. (2012). An estimate of the geothermal energy resource in the major sedimentary basins in the United States. Trans. - Geotherm. Resour. Counc. 36, 1359–1369.
Randolph, J. B., and Saar, M. O. (2011). Combining geothermal energy capture with geologic carbon dioxide sequestration. Geophys. Res. Lett. 38 (May), 1–7. doi:10.1029/2011GL047265
Robins, J. C., Kolker, A., Flores-Espino, F., Pettitt, W., Schmidt, B., Beckers, K., et al. (2021). 2021 U.S. Geothermal power production and district heating market report. Available at: https://www.nrel.gov/docs/fy21osti/78291.pdf.
Sepulveda, N. A., Jenkins, J. D., de Sisternes, F. J., and Lester, R. K. (2018). The role of firm low-carbon electricity resources in deep decarbonization of power generation. Joule 2 (11), 2403–2420. doi:10.1016/j.joule.2018.08.006
USDOE (2019). GeoVision: harnessing the heat beneath our feet. Available at: https://www.energy.gov/sites/prod/files/2019/06/f63/GeoVision-full-report-opt.pdf.
USGS (2022). USGS CO2Viewer. Available at: https://co2public.er.usgs.gov/viewer/%0A.
Williams, C. F., Reed, M. J., and Mariner, R. H. (2008). A Review of Methods Applied by the U.S. Geological Survey in the Assessment of Identified Geothermal Resources. Open-File Rep. 2008–1296. Available at: https://pubs.usgs.gov/of/2008/1296/pdf/of2008-1296.pdf. doi:10.3133/ofr20081296
Keywords: sedimentary basin geothermal power, geologic CO2 storage, CPG, genGEO, SCO2TPRO
Citation: Cairncross E, Ogland-Hand JD, Adams BM and Middleton RS (2024) Nationwide cost and capacity estimates for sedimentary basin geothermal power and implications for geologic CO2 storage. Front. Energy Res. 12:1422285. doi: 10.3389/fenrg.2024.1422285
Received: 23 April 2024; Accepted: 03 July 2024;
Published: 30 July 2024.
Edited by:
Stuart M. V. Gilfillan, University of Edinburgh, United KingdomReviewed by:
Juan Alcalde, Geosciences Barcelona, Spanish National Research Council (CSIC), SpainDaniel Elliott Campbell, University of Rhode Island, United States
Copyright © 2024 Cairncross, Ogland-Hand, Adams and Middleton. This is an open-access article distributed under the terms of the Creative Commons Attribution License (CC BY). The use, distribution or reproduction in other forums is permitted, provided the original author(s) and the copyright owner(s) are credited and that the original publication in this journal is cited, in accordance with accepted academic practice. No use, distribution or reproduction is permitted which does not comply with these terms.
*Correspondence: Jonathan D. Ogland-Hand, am9uYXRoYW4ub2dsYW5kLWhhbmRAY2FyYm9uc29sdXRpb25zbGxjLmNvbQ==