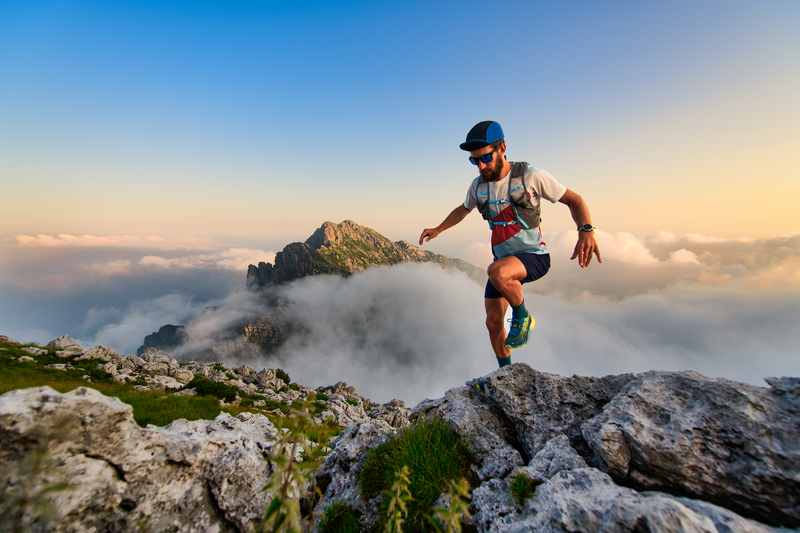
95% of researchers rate our articles as excellent or good
Learn more about the work of our research integrity team to safeguard the quality of each article we publish.
Find out more
ORIGINAL RESEARCH article
Front. Energy Res. , 03 July 2024
Sec. Solar Energy
Volume 12 - 2024 | https://doi.org/10.3389/fenrg.2024.1399020
Over the past few years, there has been a growing interest in renewable energy sources. Among them, photovoltaic (PV) technology is advancing rapidly. Solar insolation is the most crucial factor for PV installations. Various solutions, such as tracking mechanisms, hybrid systems, and new materials, can enhance the efficiency of PV systems. Concentrators focus solar light onto the surface of solar modules, increasing production of electricity. Implementing such solutions can reduce the number of silicon cells in installations, leading to a decrease in waste generated during production. Dye concentrators have a positive impact on the performance of silicon systems. A two-stage study on the effect of dye concentrator application on PV cell efficiency is carried out. In the first stage, specific types of dye concentrators are tested for their interaction with the silicon system. Tinted and luminescent acrylic glass (polymethyl methacrylate, PMMA) in yellow and red are used as dye concentrators. The experiment included multiple measurement calibrations, such as the temperature of the tested silicon cell and the intensity of illuminance. Results showed absolute increase of efficiency in solar cells ranging from 0.05% to 1.42%, depending on the type of concentrator used. The most significant improvements were observed with luminescent red PMMA, averaging at 1.21%. The potential of this concentrator was further explored in the second stage of the study, investigating the relationship between the surface involvement of the silicon cell and the dye concentrator. Test results indicated the potential of dye concentrators for integrating luminescent dye concentrator technology into PV systems. The effect of this integration is increase in the efficiency of the PV cell. On the other hand, it should be noted that replacing the PV cell with a dye concentrator reduces the efficiency of the entire photovoltaic system. Hence, the use of a PV cell and concentrator system is recommended especially for photovoltaic systems with a large area. As dye concentrators have the ability to operate without direct irradiance, they are also recommended for regions where natural light is dispersed.
Climate policy in many countries, including those in the European Union, emphasizes the development of renewable energy. One of the most commonly utilized technologies for this purpose is photovoltaics, aligning with sustainable development goals (SDGs) (Poranek et al., 2022). In recent years, significant advancements in PV technology have led to its widespread adoption. The development of photovoltaic technology also provides methods for detecting and limiting the impact of PV system faults (Alam et al., 2015). An increasing number of reports are also devoted to methods of numerical optimization of the operation of PV systems (Fadakar Masouleh et al., 2016; Chen et al., 2023). Predictions for PV technology indicate further growth and an increased share in electricity production. The predominant technology in photovoltaic panels is currently crystalline silicon (c-Si). Photovoltaic panels are typically categorized into three generations: I, II, and III. The first generation includes crystalline silicon (c-Si) panels, which constitute the vast majority (approximately 95%) of currently used panels (Goetzberger and Hebling, 2000). Therefore, this study focuses on c-Si cells. It is worth noting that the production process of silicon cells results in significant waste, and their performance is closely tied to atmospheric conditions. Material losses during the production of silicon wafers are estimated to reach up to 40% (Green and Staffel, 2016; Ortega-Izquierdo and Del Rio, 2016). Consequently, solutions that conserve silicon while maximizing energy output from silicon cells are becoming increasingly important (Esen et al., 2017; Korzeniowska et al., 2020). Dye concentrators are a solution that meets these criteria, allowing solar light to be concentrated on the solar cell, thus maximizing cell efficiency while reducing the surface area of the silicon cell (Kenny et al., 2013; Zdanowicz, 2020). The concentrators act by absorbing sunlight and then emitting light from the concentrator, among others, towards the surface of the solar cell. It is possible thanks to the bending of the light beam (Assadi et al., 2016). The potential utilization of concentrators as solar light focusing elements on photovoltaic cells is particularly relevant for solar energy in Central European countries characterized by relatively low amount of solar energy reaching the surface of Earth. To show the difference one can use irradiation examples of Poland and Czech Republic (about 1,000–1,050 kWh/m2∙year). In contrast, Southern European countries have much higher values, namely, France (about 1,300 kWh/m2∙year) and Spain (about 1,700 kWh/m2∙year). Therefore, it is justified to search methods to increase PV cells efficiency in less favorable conditions (El-Bashir, 2018). The use of concentrators also allows for maximizing PV cell efficiency on cloudy days when solar radiation is largely diffuse (El Himer et al., 2020). In general, solar concentrators can be classified as imaging and non-imaging. In the case of imaging concentrators, an optical image of the Sun is created on the receiver (Mortadi and El Fadar, 2023). Due to the fact that they focus light on a line or point, there are linear concentrators (for instance, parabolic trough and linear Fresnel reflector) and point concentrators (for instance, parabolic dish and solar tower) (Chauhan et al., 2021). In the second class of concentrators, image of the Sun is not created, and the light is scattered in the receiver (Castelletto and Boretti, 2023). This type of device are characterized by a relatively low concentration coefficient in comparison to imaging concentrators (Madala and Boehm, 2017). However, the advantage of using this type of concentrator is the ability to use diffuse light under low irradiance conditions.
Many methods enable efficient concentration of solar radiation on photovoltaic installations, thereby increasing their efficiency. The simplest method to enhance the efficiency of a photovoltaic installation is sun-tracking mechanism. This mechanism allows for the rotation of the photovoltaic system to achieve the best position relative to the position of Sun on the horizon. One can distinguish here single-axis or two-axis solutions (Tan et al., 2021). This ensures optimal alignment between the surface of silicon wafers and solar light (Singh et al., 2018). Another example is the hybrid photovoltaic-thermal system. The aim of this system is to produce both electricity and heat, which also helps in reducing PV module fluctuations (Roberson et al., 2019). Many concentrator technologies facilitate increased efficiency of PV installations. The Fresnel lens is one of the commonly used optical elements in PV systems (Davis, 2011). This type of element can be utilized in circular and rectangular flat forms. Research conducted by A. Davis indicated that a circular shape has better transmittance (82%) compared to a rectangular shape (80%) (Canavarro et al., 2013). The optical efficiency of such technology is closely related to the focal length.
Another type of concentrator technology is the parabolic mirror. In this solution, the concentrator is the component that collects light from a given area and redirects it to the photovoltaic installation. The study by Canavarro et al. (2013) showed that a low concentration equal to 70 suns can achieve results of 80% optic efficiency (Hasan et al., 2010). The challenge with this type of solution lies in the requirement for long-term positioning for analysis. The performance of the system is closely tied to the sun-tracking mechanism. Proper positioning of the parabolic mirror yields the best results in the overall performance of the photovoltaic installation. Apart from the sun-tracking mechanism, the most significant issue for traditional solar concentrators is the increase in temperature of the solar cell after redirecting sunlight by the concentrator.
The concentrator, which enhances the performance of the Solar System and theoretically offers opportunities to stabilize temperature of the system, should be developed. Commonly used pigments in the dye-sensitized solar cells (DSSC) industry could serve as the proposed concentrator. A liquid concentrator in the form of natural dye, typically utilized in dye solar cells, thus presents an alternative solution that could effectively complement traditional silicon cells (first generation cells) to enhance their operational efficiency. However, natural dye is not the sole available option. In the DSSC industry, two types of cells can be distinguished: metallic dye and natural dyes (derived from various plant parts or synthesized in laboratories). The most popular and effective dyes in the DSSC cell industry are ruthenium (metallic) dyes. Nonetheless, it is important to note that when a dye is used as a concentrator, its efficiency for DSSC cells cannot be evaluated with expectations of similar results. This is because the dye used in DSSC cells serves as a catalyst, initiating reactions aimed at electricity generation when stimulated by light. As a concentrator, the dye merely functions as a specific lens, focusing solar radiation on the targeted cell. An advantageous aspect of natural dyes, supporting their use as a concentrator, is their minimal environmental impact. Dyes employed to modify silicon cells should be natural and biodegradable. Dyes utilized in DSSC cell technology must exhibit the following properties:
• high stability, enabling operation for approximately 20 years under natural light conditions,
• possess the capacity to absorb light with wavelengths below 920 nm,
• characterized by a high redox potential to facilitate efficient regeneration by the electrolyte,
• that allow them to bond to the surface of the electrical conductor.
The requirements for dyes used as concentrators are not as stringent as those for dyes used in DSSC cells. As mentioned earlier, these dyes are utilized as elements that concentrate solar radiation onto a silicon cell; they do not generate electricity themselves and thus do not need to undergo a series of chemical reactions, exhibit properties enabling connection to the conductor surface, or possess redox properties. Therefore, the most important requirements for a dye concentrator are its high stability, allowing operation for the lifetime equivalent to that of a silicon cell, and its ability to focus sunlight (Tan et al., 2021). The principle of the LSC operation is shown in Figure 1. It presents the light route in the luminescent concentrator.
At first, the light falls on the surface of the concentrator (1 natural irradiance) and part of it is absorbed (3), but the other part is reflected by the surface of the concentrator (2). The absorbed light part goes through the material of the concentrator (4) and the others are absorbed and reflected by the pigments (9), reabsorbed by neighbor dye molecules (5), and lost during transmission in the material (10). The numbers (6) in the schema are an escape-cone loss, (7) is transmitted radiation, and (8) is non-radiative decay.
A concentrator in the form of luminescent PMMA, which means that it is embedded in poly (methyl methacrylate), can be integrated into luminescent solar concentrator technology (LSC) (Zhou et al., 2015). LSC, which incorporates luminescent pigments, partially bends and absorbs sunlight to fluoresce it at a longer wavelength. This phenomenon, known as the Stokes shift, describes the shift between the absorbed and emitted light in fluorescence (Brennan et al., 2018; Moraitis et al., 2018). It should be noted that the technology, which combines irradiance concentration and light emission after Stokes shifting, can only be used after analyzing the spectral response for a solar cell (Zhou et al., 2016). The luminescent solution offers several advantages over other types of concentrator technology. The most significant advantage is its ability to operate without direct irradiation, which is particularly beneficial in regions where natural light is dispersed. Thanks to this quality, it can be effectively implemented in solar installations in terms of efficiency (Goetzberger and Hebling, 2000) Another advantage of this technology is that it can function without the need for a sun-tracking mechanism and does not require large elements that occupy a large area around the cells/modules (McKenna and Evans, 2017; Baiju and Yarema, 2022). Another important issue here is the implementation of sustainable solutions (Hernández-Rodríguez et al., 2022).
There are many scientific reports on LSC systems with PMMA as the host polymer. Theoretical considerations on this topic often deal with the update of LSC technology, as well as economic issues. Castelletto and Boretti (2023) in their review work focused, among other things, on the possibility of reducing optical losses and improving the efficiency of photon capture. Van Sark (2012) assessed the LSC solution as a low-cost alternative, especially for north-central areas of Europe. Practical research focuses on determining the properties of a number of LSC base compounds embedded in the polymer, as well as examining the possibilities of new applications of the created photovoltaic systems. One example combining these issues is the study of Frias et al. (2019), who developed a transparent lanthanide based LSC embedded in PMMA as transparent coatings. It is suggested to use them on facades and windows in order to achieve zero-emission buildings. Ahmed et al. (2024) studied LSC in a system with PMMA doped with zinc or nickel complexes. The Zn(II)/PMMA LSC complex performed favorably here, primarily in terms of degradation speed. This study investigates the alterations in operational parameters of silicon cells when enveloped by pigmented and luminescent planar PMMA. The selected materials was chosen primarily due to their high optical efficiency. This applies especially luminescent concentrators, which was tested by, among others, Zetti et al. (2016). The author considers this solution to be the most optimal, as it involves covering the silicon cells with luminescent plates, leading to reduced operational parameters for the solar cells under examination. In this study, four types of dye concentrators are proposed to surround the solar cell. A multi-variant study of the efficiency of individual photovoltaic systems (PV cell with a dye concentrator) is carried out in conditions of variable sunlight and temperature. The obtained results are related to the base system consisting only of PV cells. The findings presented in this paper are based on a basic laboratory-scale experiments. Then, the material with the best properties will be subjected to a broader analysis to check the course of changes in the efficiency of the photovoltaic system depending on the share of the photovoltaic cell surface area and the selected dye concentrator.
The presented research utilized crystalline silicon cells. The basic parameters of the tested silicon cells in STC (Standard Testing Conditions) are presented in Table 1. The values are experimentally measured.
Measurements were conducted using a photovoltaic research stand, which includes: Keithley SMU2401 meter for current measurement < 1 nA–1 A, voltage measurement up to 20 V; measurement table with integrated SS05SA LED solar simulator (class AAA; the table allows determining the temperature of the tested cell in the range of 10°C–60°C using an air-cooled Peltier module), solar cell suction system, and Kelvin probe; auxiliary unit version 3.0 (computer workstation with software enabling control of temperature, irradiation level, cell suction, probes, and other functions). Figure 2 presents the laboratory stand.
The first step in the research was to determine the level of irradiance and temperature at which the measurements would be conducted. All results presented in the article are the average of (at least) three consecutive measurements. This procedure reduces errors caused by light instability. The basic characteristics of the cell were measured using the Keithley meter. The dye concentrators used in the experiment were 4 PMMA plates surrounding the tested solar cell. Each plate had a width of 2.5 cm, thickness of 0.3 cm, and their total length was up to 5 cm, while the total length of the other two was 10 cm. Figure 3 depict the arrangement connecting the PV cells with the dye concentrator.
The fluctuations in the illumination level were approximately 10 W/m2. This means that measurements for irradiance of 1,000 W/m2 could be conducted within the range of 990–1,010 W/m2. The output power was calculated using Eq. 1:
where:
A – surface of the silicon cell, m2,
Pin – amount of solar irradiance reaching the solar cell, W/m2,
ƞ – efficiency of the silicon cell.
Table 2 presents measurements for a clear polycrystalline silicon cell under various irradiance levels at defined temperatures. Upon analyzing the results in terms of illumination, it can be observed that the efficiency of the cell remains stable. Lowering the temperature of the cell led to an enhancement in the performance of the system, a phenomenon well-recognized among photovoltaic module manufacturers. Each module employed in a photovoltaic installation has its designated operating temperature, outlined in its data sheet included in the technical specifications. Tables 2–6 present the measurement results for each tested variant. The following variants of photovoltaic systems are tested:
• PV - clear PV cell,
• PV + PR - clear PV cell and pigmented red PMMA,
• PV + PY - clear PV cell and pigmented yellow PMMA,
• PV + LR - clear PV cell and luminescent red PMMA,
• PV + LY - clear PV cell and luminescent yellow PMMA.
Table 2. Results for the clear PV cell (EFF av, average efficiency of PV cell; Pm av, average power output; Voc av, average open circuit voltage; I sc av, average short circuit current).
Tables 3, 4 present the results for systems utilizing pigmented red and yellow PMMA.
In Tables 3, 4, one can observe the outcomes for systems employing a dye concentrator to bend and concentrate the illumination onto the surface of a functioning silicon cell. By comparing these results with those of the basic system utilizing only a clear polycrystalline silicon cell, one can infer absolute change in performance ranging from −0.4% to + 0.26% for red PMMA. The negative value is notable under low irradiance and temperature conditions. For yellow PMMA, the performance results range from −0.54% to + 0.7%. The negative value for yellow PMMA was only recorded under very low irradiance (equal to 200 W/m2) and a low temperature of 16°C. This data suggests that PMMA pigmented with yellow color may bend light better than similar red PMMA. Additionally, a slight improvement in amperage value for the tested cell is observed. The voltage value fluctuation falls within the measurement error of the device utilized for the measurements. For yellow PMMA, the average absolute increase in Isc was approximately 14.24 mA and 1.05 mV for Voc, while for red PMMA - for Isc (3.63 mA) and around 1.21 mV for Voc. These findings indicate that yellow pigmented PMMA exhibits superior bending capabilities compared to the red pigmented PMMA.
Tables 5, 6 present the results of the investigations into the system with luminescent PMMA. This type of solution not only allows for bending sunlight through the PMMA plate but also utilizes the luminescence effect to enhance the efficiency of the solar cell. The Stokes shift is a well-known concept in luminescence. The idea of the Stokes shift describes the change in the spectrum of light before and after its emission through a luminescent pigment. A red luminescent pigment will emit light shifted towards the red spectrum; theoretically, this means that for silicon cell technology, which performs better under such conditions, its efficiency will increase.
Upon analysis of the provided data and comparison with results for the pure silicon cell, we observe higher efficiency in the system utilizing red luminescent PMMA. The average absolute change in results obtained for red luminescent PMMA (PV + LR) were 1.21%, while for yellow (PV + LY), it was 0.25%. The highest result for the first one was achieved with an irradiance of 400 W/m2 and a temperature of 28°C, yielding 1.42%. Similarly, for the yellow option, irradiance at 400 W/m2 and a temperature of 25°C resulted in 0.54%. It is noteworthy that three out of five measurements for the yellow option at the lowest irradiance showed a negative value. The results obtained for red luminescent PMMA demonstrated an increase in the efficiency of the silicon cell. Comparing the results obtained for pigmented and luminescent concentrator, it is evident that pigmented yellow PMMA (PV + PY) exhibits better bending characteristics than its red counterpart (PV + PR). Also, the results obtained for pigmented yellow PMMA were higher than for its luminescent counterpart (PV + LY). Conversely, the opposite situation occurred for the red dye.
Figure 4 presents average efficiency of PV cell in tested variants.
As mentioned above, the variant with red luminescent PMMA (PV + LR) outperforms the others in terms of PV cell efficiency. The results demonstrate the highest efficiency and the greatest improvement compared to the system without concentrators. The PV + LR variant achieved the highest average efficiency under conditions of 16°C and irradiation of 200 W/m2. Additionally, the peak absolute increase of efficiency for this variant was observed at a temperature of 28°C and irradiance of 400 W/m2, reaching 1.42%. All results using the red luminescent PMMA indicate an enhancement in the performance of the silicon cell. The situation is slightly different for the other variants. Although their average performance is similar, the PV + PY variant, with yellow pigmented PMMA, stands out as the most favorable. The highest efficiency for this variant was recorded at 16°C with an irradiance of 400 W/m2, while the greatest increase in efficiency compared to the PV variant was at the same irradiance level but at 25°C, with absolute increase of 0.58%. Taking into account test results, red luminescent PMMA was selected for further research.
To evaluate the potential application of organic dyes as a focusing component for radiation on a photovoltaic module, it is essential to analyze systems with a constant surface area depending on the amount of dye concentrator used and compare them. This method involves establishing multiple systems with a consistent surface area but varying proportions of the silicon cell and dye concentrator surface areas. In this adopted approach, the base system utilizing only silicon cells (without modification) is denoted as System 0. Subsequent systems gradually increase the concentration of the dye surface area at the expense of the silicon cell surface area. The following percentages of dye concentrator were employed: 0% (System 0), 25% (System 1), 56% (System 2), 69% (System 3), 79% (System 4), 88% (System 5), and 93% (System 6). The silicon cells utilized in the conducted tests demonstrated an efficiency of 15%. This is a baseline value of efficiency of solar cell without dye concentrators in a given conditions. Source of this value are measurements. The measurement were performed for various lighting and temperature conditions, reflecting the conditions throughout the year. The reference were the conditions of a temperate continental climate – South of Poland, Central Europe.
Data concerning the energy output of the presented solution, dependent on the surface area of the utilized concentrator and silicon cell, are depicted in Table 7. Additionally, the results were calculated based on the efficiency of the entire system (comprising the surface area of the silicon cell and the concentrator) and the efficiency attributed solely to the surface area of the silicon cell.
Table 7. Results of the second stage tests – changing surface of silicon cells and dye concentrator (Ei, energy obtained from the installation; Ssc, share of silicon cells; Sdc, share of dye concentrator; Esc, energy obtained from silicon cells; EFFsc, efficiency per m2 of silicon cells; EFFs, efficiency per m2 of the system; S, system).
Analyzing the results, two potentially conflicting conclusions can be drawn. Increasing the surface area of the concentrator allows for an enhancement in the efficiency of the silicon cell. The utilization of the concentrator enables the maximization of the amount of electrical energy generated by the silicon cell. This solution facilitates achieving higher efficiency of the installation on days with scattered sunlight (low irradiance - winter days). On the other hand, the installation with the dye concentrator introduced exhibits lower efficiency compared to the baseline comparative system. The amount of energy obtained from PV systems is closely related to the surface area of the installation converting solar energy into electrical energy. The concentrator serves as a component for focusing solar radiation on silicon cells. It does not convert solar radiation into electricity but rather acts as a component of the system responsible solely for increasing the efficiency of PV cells.
Dye concentrators are a solution that can be successfully applied in countries where the scattering of solar radiation limits the development of photovoltaics. Countries located in regions with four seasons (spring, summer, autumn, and winter) could effectively utilize the described solution to maximize energy efficiency throughout the year, especially during the winter period when the scattering of solar radiation is at its highest. Dye concentrators can also be used in countries located in regions with intense sunlight to increase the efficiency of solar cells. The key properties of dyes include high stability, the ability to focus solar radiation on the PV system, and long-term usability. This technology can contribute to reducing the consumption of materials used in the production of silicon cells, thereby minimizing the environmental impact of the production process and waste generated throughout its lifecycle. The key aspects of this technology, which are still in the research phase, include:
• placement of the dye concentrator to maximize the efficiency of the photovoltaic cell while minimizing the surface area of the photovoltaic installation,
• optimal ratio of the concentrator to PV system components,
• dyes characterized by high stability and long-life cycle, developed based on environmentally friendly materials and the principle of minimizing costs as much as possible.
The concept of concentrators is well-established in the photovoltaic industry, typically in the form of mirrors and lenses. However, the utilization of an LSC concentrator in the form of pigmented PMMA as a light-directing component for PV cells shows promise. The results presented in the article demonstrate the positive impact of using a dye concentrator, particularly in the form of luminescent PMMA. The tested cells showed an average efficiency increase of 1.21% for red luminescent PMMA, 0.25% for yellow luminescent PMMA, 0.41% for yellow pigmented PMMA, and 0.13% for red pigmented PMMA. Moreover, the results clearly suggest that implementing a dye concentrator in photovoltaic technology can lead to an increase in current intensity. During testing, the highest increase in Isc was observed with red luminescent PMMA and pigmented yellow PMMA. The study presents a basic laboratory-scale experiments. For this reason, specific physical phenomena responsible for changes in the efficiency of the photovoltaic system after adding the concentrator were not considered. The relationships between the share of the concentrator in the PV system and the efficiency of the cell and the efficiency of the PV system were, among others, examined as part of multi-variant tests.
Based on these findings, the concentrator with the most favorable properties was selected for the second stage of the study, which turned out to be red luminescent PMMA. The next part of the research allows checking the relationship between the surface of solar cells and the dye concentrator in the photovoltaic system and the efficiency of the cells and the entire PV system. For a fixed area of the PV system, seven simulations were performed, starting with a system whose area is 100% made up of solar cells, and ending with a system where the cells occupy only 7% of the area and the rest is the dye concentrator. The results clearly indicate two trends. The first is a significant increase in the efficiency of the PV cell and an increase in the energy obtained from it along with the increase in the concentrator area. This is an almost threefold increase in these parameters. The second one indicates a decrease in the efficiency of the entire PV system and a decrease in the energy obtained from the entire system under the same conditions. The decrease in efficiency and energy obtained in this case is approximately fivefold.
It can be concluded that this technology holds potential regarding enhancement of solar cell efficiency especially for countries experiencing all four seasons, with significant scattered irradiation during the winter season. Additionally, the operational dynamics of this technology in larger-scale modular systems need to be considered. Each module comprises multiple cells connected in series and parallel, with the efficiency of the module theoretically equivalent to that of the weakest cell. Hence, enhancing the efficiency of several cells may not produce the anticipated outcomes. It is crucial to maintain LSC alongside the photovoltaic installation to avoid any potential reduction in expected effects due to damage to either the concentrator or the module. Furthermore, standard photovoltaic solutions necessitate regular maintenance to eliminate contaminants that accumulate on surface of the module.
The tested system was analyzed only on a laboratory scale. It has the potential to be used in large power plants. Assuming a limited area for the construction of a photovoltaic installation, the obvious solution is to use traditional panels without a concentrator (for example, on the roof surface). However, if the area occupied by the installation is not severely limited, the use of a concentrator will potentially allow for a reduction in the number of panels (less waste after the end of the LCA use phase) or a greater amount of energy obtained.
The raw data supporting the conclusions of this article will be made available by the author, without undue reservation.
EB: Conceptualization, Formal Analysis, Funding acquisition, Project administration, Supervision, Writing–original draft, Writing–review and editing. MB: Conceptualization, Data curation, Formal Analysis, Investigation, Methodology, Resources, Validation, Writing–review and editing. AW: Conceptualization, Formal Analysis, Investigation, Methodology, Validation, Visualization, Writing–original draft, Writing–review and editing. BM: Conceptualization, Data curation, Formal Analysis, Methodology, Validation, Writing–review and editing.
The authors declare that financial support was received for the research, authorship, and publication of this article. Research was funded by the statutory research grant number SUBB 08/030/BK_24/0131 by the Faculty of Energy and Environmental Engineering, Silesian University of Technology.
Author BM was employed to Huta Bankowa.
The remaining authors declare that the research was conducted in the absence of any commercial or financial relationships that could be construed as a potential conflict of interest.
All claims expressed in this article are solely those of the authors and do not necessarily represent those of their affiliated organizations, or those of the publisher, the editors and the reviewers. Any product that may be evaluated in this article, or claim that may be made by its manufacturer, is not guaranteed or endorsed by the publisher.
Ahmed, R. K., Mohamed, N. M., and Reda, S. M. (2024). Enhanced efficiency of silicon solar cell by new salophen complexes embedded PMMA as light concentrators. J. Inorg. Organomet. Polym. 2024. doi:10.1007/s10904-024-03070-y
Alam, M. K., Khan, F., Johnson, J., and Flicker, J. (2015). A comprehensive review of catastrophic faults in PV arrays: types, detection, and mitigation techniques. IEEE J. Photovoltaics 5 (3), 982–997. doi:10.1109/jphotov.2015.2397599
Assadi, M. K., Hanaei, H., Mohamed, N. M., Saidur, R., Bakhoda, S., Bashiri, R., et al. (2016). Enhancing the efficiency of luminescent solar concentrators (LSCs). Appl. Phys. A 122, 821. doi:10.1007/s00339-016-0359-2
Baiju, A., and Yarema, M. (2022). Status and challenges of multi-junction solar cell technology. Front. Energy Res. Sol. Energy 10. doi:10.3389/fenrg.2022.971918
Brennan, L. J., Purcell-Milton, F., McKenna, B., Watson, T. M., Gun’ko, Y. K., and Evans, R. C. (2018). Large area quantum dot luminescent solar concentrators for usewith dye-sensitised solar cells. J. Mater. Chem. A 6, 2671–2680. doi:10.1039/c7ta04731b
Canavarro, D., Chaves, J., and Collares-Pereira, M. (2013). New second-stage concentrators (XX SMS) for parabolic primaries; Comparison with conventional parabolic trough concentrators. Sol. Energy 92, 98–105. doi:10.1016/j.solener.2013.02.011
Castelletto, S., and Boretti, A. (2023). Luminescence solar concentrators: a technology update. Nano Energy 109, 108269. doi:10.1016/j.nanoen.2023.108269
Chauhan, V. K., Shukla, S. K., Tirkey, J. V., and Rathore, P. K. S. (2021). A comprehensive review of direct solar desalination techniques and its advancements. J. Clean. Prod. 284, 124719. doi:10.1016/j.jclepro.2020.124719
Chen, H., Cheng, J., Zhenhua, Li, Abu-Siada, A., and Hongbin, Li (2023). Distributed photovoltaic power fluctuation flattening strategy based on hybrid energy storage. Front. Energy Res. 11. doi:10.3389/fenrg.2023.1303522
Davis, A. (2011) “Fresnel lens solar concentrator derivations and simulations,” in Novel optical systems design and optimization XIV, 8129. Bellingham, WA, USA: International Society for Optics and Photonics, 81290.
El-Bashir, S. M. (2018). Enhanced fluorescence polarization of fluorescent polycarbonate/zirconia nanocomposites for second generation luminescent solar concentrators. Renew. Energy 115, 269–275. doi:10.1016/j.renene.2017.08.016
El Himer, S., El Ayane, S., El Yahyaoui, S., Salvestrini, J. P., and Ahaitouf, A. (2020). Photovoltaic concentration: research and development. Energies 13, 5721. doi:10.3390/en13215721
Esen, V., Saglam, S., and Oral, B. (2017). Light sources of solar simulators for photovoltaic devices: a review. Renew. Sustain. Energy Rev. 77, 1240–1250. doi:10.1016/j.rser.2017.03.062
Fadakar Masouleh, F., Das, N., and Rozati, S. M. (2016). Nano-structured gratings for improved light absorption efficiency in solar cells. Energies 9, 756. doi:10.3390/en9090756
Frias, A. R., Cardoso, M. A., Bastos, A. R. N., Correia, S. F. H., André, P. S., Carlos, L. D., et al. (2019). Transparent luminescent solar concentrators using Ln3+-based ionosilicas towards photovoltaic windows. Energies 12, 451. doi:10.3390/en12030451
Goetzberger, A., and Hebling, C. (2000). Photovoltaic materials, past, present, future. Sol. Energy Mater. Sol. Cells 62, 1–19. doi:10.1016/s0927-0248(99)00131-2
Green, R., and Staffel, I. (2016). Electricity in Europe: existing fossil fuels. Oxf. Rev. Econ. Policy 32, 282–303. doi:10.1093/oxrep/grw003
Hasan, A., McCornack, S. J., Huang, M. J., and Norton, B. (2010). Evaluation of phase change materials for thermal regulation enhancement of building integrated photovoltaics. Sol. Energy 84, 1601–1612. doi:10.1016/j.solener.2010.06.010
Hernández-Rodríguez, M. A., Correia, S. F. H., Ferreira, R. A. S., and Carlos, L. D. (2022). A perspective on sustainable luminescent solar concentrators. J. Appl. Phys. 131, 140901. doi:10.1063/5.0084182
Kenny, R. P., Viganó, D., Salis, E., Bardizza, G., Norton, M., Müllejans, H., et al. (2013). Power rating of photovoltaic modules including validation of procedures to implement IEC 61853-1 on solar simulators and under natural sunlight. Prog. Photovolt. Res. Appl. 21, 1384–1399. doi:10.1002/pip.2365
Korzeniowska, E., Tomczyk, M., Pietrzak, Ł., Hadziselimovic, M., Stumberger, B., Sredensek, K., et al. (2020). Efficiency of laser-shaped photovoltaic cells. Energies 13, 4747. doi:10.3390/en13184747
Madala, S., and Boehm, R. F. (2017). A review of nonimaging solar concentrators for stationary and passive tracking applications. Renew. Sustain. Energy Rev. 71, 309–322. doi:10.1016/j.rser.2016.12.058
McKenna, B., and Evans, R. C. (2017). Towards efficient spectral converters through materials design for luminescent solar devices. Adv. Mater. 29, 1606491. doi:10.1002/adma.201606491
Moraitis, P., Schropp, R. E. I., and van Sark, W. G. (2018). Nanoparticles for luminescent solar concentrators—a review. Opt. Mater. 84, 636–645. doi:10.1016/j.optmat.2018.07.034
Mortadi, M., and El Fadar, A. (2023) “Chapter 1 - solar thermal systems: applications, techno-economic assessment, and challenges,” in Advances in renewable energy technologies, renewable energy production and distribution, 2. Academic Press, 3–62. doi:10.1016/B978-0-443-18439-0.00005-7
Ortega-Izquierdo, M., and Del Rio, P. (2016). Benefits and costs of renewable electricity in Europe. Renew. Sustain. Energy Rev. 61, 372–383. doi:10.1016/j.rser.2016.03.044
Poranek, N., Łaźniewska-Piekarczyk, B., Lombardi, L., Czajkowski, A., Bogacka, M., and Pikoń, K. (2022). Green deal and circular economy of bottom ash waste management in building industry—alkali (NaOH) pre-treatment. Materials 15, 3487. doi:10.3390/ma15103487
Roberson, J., Riggs, B., Islam, K., Vera Ji, Y., Spitler, C. M., Gupra, N., et al. (2019). Field Testing of spectrum-splitting transmissive concentrator photovoltaic module. Renew. Energy 139. doi:10.1016/j.renene.2019.02.117
Singh, R., Kumar, S., Gehlot, A., and Pachauri, R. (2018). An imperative role of sun trackers in photovoltaic technology: a review. Renew. Sustain. Energy Rev. 82, 3263–3278. doi:10.1016/j.rser.2017.10.018
Tan, M.-H., Wang, T.-K., Wong, C.-W., Chong, K.-K., Lim, B.-H., Yew, T.-K., et al. (2021). Comprehensive methodology to evaluate parasitic energy consumption for different types of dual-Axis sun tracking systems. Int. J. Photoenergy 2021, 1–12. doi:10.1155/2021/2870386
van Sark., W. G. J. H. M. (2012). “Luminescent solar concentrators—a low cost photovoltaics alternative,” in EPJ Web of Conferences, 2nd European Energy Conference, London, UK, November 11-12, 2024, 02003.
Zetti, M., Mayer, O., Klampaftis, E., and Richards, B. S. (2016). Investigation of host polymers for luminescent solar concentrators. Energy Technol. 5 (7), 1037–1044. doi:10.1002/ente.201600498
Zhou, W., Wang, M.-C., and Zhao, X. (2015). Poly(methyl methacrylate) (PMMA) doped with DCJTB for luminescent solar concentrator applications. Sol. Energy 115, 569–576. doi:10.1016/j.solener.2015.03.012
Keywords: photovoltaic cells, solar concentrator, silicon cells, solar energy, dyes, renewable energy
Citation: Brągoszewska E, Bogacka M, Wajda A and Milewicz B (2024) Enhancing the efficiency of photovoltaic cells through the usage of dye concentrators. Front. Energy Res. 12:1399020. doi: 10.3389/fenrg.2024.1399020
Received: 11 March 2024; Accepted: 14 June 2024;
Published: 03 July 2024.
Edited by:
Eduardo F. Fernández, University of Jaén, SpainReviewed by:
Narottam Das, Central Queensland University, AustraliaCopyright © 2024 Brągoszewska, Bogacka, Wajda and Milewicz. This is an open-access article distributed under the terms of the Creative Commons Attribution License (CC BY). The use, distribution or reproduction in other forums is permitted, provided the original author(s) and the copyright owner(s) are credited and that the original publication in this journal is cited, in accordance with accepted academic practice. No use, distribution or reproduction is permitted which does not comply with these terms.
*Correspondence: Ewa Brągoszewska, ZXdhLmJyYWdvc3pld3NrYUBwb2xzbC5wbA==
Disclaimer: All claims expressed in this article are solely those of the authors and do not necessarily represent those of their affiliated organizations, or those of the publisher, the editors and the reviewers. Any product that may be evaluated in this article or claim that may be made by its manufacturer is not guaranteed or endorsed by the publisher.
Research integrity at Frontiers
Learn more about the work of our research integrity team to safeguard the quality of each article we publish.