- College of Energy and Electrical Engineering, Hohai University, Nanjing, Jiangsu, China
With the rapid development of photovoltaic power generation, how to improve the photovoltaic grid connection rate is an urgent problem to be solved. This article proposes an optimized scheduling method for the water and photovoltaic complementary system, taking into account the operation strategy of pump stations to improve the photovoltaic grid connection rate. Firstly, a multi-objective optimization scheduling model is constructed to consider both power generation and output fluctuation, and the uncertainty of photovoltaic power generation is analyzed from multiple perspectives. Then, taking the cascade hydropower stations and surrounding photovoltaic power stations in a river basin in Sichuan as an example, the operation strategy of pump stations is introduced into the water and photovoltaic complementary system, considering different weather scenarios, to reduce the photovoltaic curtailment rate. The study verifies that the introduction of pump stations can effectively increase the photovoltaic grid connection rate, and quantitatively analyzes the pump station capacity configuration under different photovoltaic penetration rates.
1 Introduction
Since the 21st century, in response to global climate change and energy crises, 31 major countries, including the United States, China, and the European Union, have established carbon reduction targets (Ding et al., 2014). As a response to climate change and to achieve green and low-carbon development, China has made solemn commitments to the international community that include peak carbon emissions and carbon neutrality goals. These commitments have given strong impetus to the development of a clean and low-carbon energy system in State Council of the People's Republic of China (2021). Vigorously developing and fully utilizing renewable energy such as wind, solar, and water is an important way to fulfill the commitment to reduce carbon emissions (National Energy Administration, 2022; Huang et al., 2021). By the end of 2022, China’s cumulative installed capacity of PV power reached 358 GW, making it the country with the largest installed capacity of PV power globally (2016). However, PV power generation exhibits significant volatility, intermittency, and randomness, posing challenges to the stable operation of the power grid when directly integrated (Pang and Zhang, 2017). Therefore, the coordinated development and operation of hydropower and PV power in a complementary manner has been proposed as an important approach to promote the integration of PV power and enhance its utilization efficiency (Zhu et al., 2021).
The complementary principle between hydropower and PV power generation lies in the fact that when the PV power generation is insufficient to meet the demand, the surplus electric energy can be utilized to elevate the water level in the hydropower station reservoir, thereby storing water energy for future needs (Liu et al., 2011). However, the integration of hydropower and PV power still faces certain challenges. Due to the intermittent and uncertain nature of PV power generation, it presents higher demands for energy scheduling and management when combined with hydropower. Rational arrangements of both power generation levels and operational status are required to achieve balanced and optimized energy supply. Furthermore, the coordinated operation of hydropower and PV power is essential, encompassing coordinated operation of power generation equipment, grid scheduling, and the safety and stability of system operation. Some research has been conducted to overcome the difficulties in integrating and accommodating PV grids. Zhu et al. (2021) proposed a daily coordinated optimization operation strategy for hydropower-PV complementarity in terms of the fluctuating power ratio. They developed an optimization model and proposed a PSO algorithm based on the sigmoid function for model resolution. Ming et al. (2018) focused on short-term dispatch of the Longyangxia hydropower-PV joint operation on the Yellow River, establishing a mathematical model for peak shaving capability and proposing a model resolution method based on simulated optimization goals, considering factors such as reservoir capacity, water level changes, and restrictions in the hydropower-PV complementarity dispatch calculation. However, these research has limited the model’s practical applicability by rigidly defining the PV power uncertainty scenario and failing to fully account for variables like environmental protection requirements, grid planning, and equipment lifespan in the hydropower-PV complementarity dispatch.
In addition, other renewable sources (Zhang et al., 2019; Yang et al., 2021; Shen et al., 2022), their operational scheduling has also received widespread attention (Liu et al., 2021; Song, 2021 constructed a scheduling model for hydropower-PV complementarity systems, employing different control strategies aimed at minimizing total output volatility and optimizing overall stability. However, the consideration of various complex factors in actual operation was insufficient, limiting the universality of the model. Hongyang et al. (2016) proposed three operational strategies based on the different degrees of hydropower regulation for PV fluctuations, while summarizing the strengths and weaknesses of each strategy. Nevertheless, the consideration of reservoir capacity constraints for hydropower stations was omitted. Luo et al., 2021 presented a probabilistic point allocation algorithm based on polynomial chaos theory for operational safety, considering the stochastic nature of PV output. Theoretically, the cascade hydropower-PV complementarity system might operate safely and steadily owing to this method, but it did not fully account for the effects of variables like variations in light intensity on system performance and operation.
The major novelty of this study is:
(1) Considering PV uncertainty from the aspects of changes in light and temperature, comprehensively considering the impact of PV uncertainty in different scenarios on system operation.
(2) Under the premise of considering ecological flow, measures are taken to reduce the impact of PV power generation uncertainty by introducing pump stations in hydropower stations, and the introduction of pump stations increases the absorption capacity of PV power generation.
(3) Through the analysis of different PV output scenarios, it is also demonstrated that the introduction of pump stations has universal applicability, and the capacity configuration of pump stations is also analyzed.
The rest of this paper is arranged as follows. In Section 2, the objective function, constraint conditions, and model resolution method of a hydropower-PV complementarity scheduling model are presented. The fundamentals of the case study are presented in Section 3, which also includes an examination of the case study results for PV output under various conditions and a capacity configuration analysis of the pumping station. Finally, the main conclusions are drawn in Section 4.
2 Complementary hydro-PV model
2.1 Objective function
Maximizing power generation and minimizing fluctuations are two important objectives in the complementary operation of hydro-PV systems (Wang et al., 2019). The multi-objective model for maximizing power generation and minimizing fluctuations enables better utilization of the complementarity of the hydro-PV system, achieving optimal power generation under different weather and lighting conditions. These two objectives are mutually constraining and balanced. These two objectives can be balanced and traded off by creating a multi-objective model, which can assist to identify the most suitable plan for overall system optimization.
1) Objective Function 1: Maximization of Power Generation within the Calculation Period.
In Eq. 1, i represents the station number; j = s, h, representing the PV power station and hydropower station, respectively; nj is the total number of stations for type j; t is the time period variable; T is the total number of time periods in the calculation period; E is the total power generation of hydro and solar, kW·h; Nj,it is the power generation output of the ith station of type j in the tth period, kW; mt is the number of hours in the tth period; η is the efficiency of the hydro turbine generator unit, typically ranging from 0.82 to 0.90 for large hydropower stations, and in this paper, η is selected as 0.82; Qh,it is the power generation flow rate of the ith hydropower station in the tth period, m3/s; H is the net working head of the hydro turbine, m.
2) Objective Function 2: Minimization of the Total Power Generation Fluctuations within the Calculation Period.
In Eq. 2, F represents the total power generation fluctuation of hydro and solar within the calculation period;
2.2 Constraint conditions
2.2.1 Generating station output constraints
where,
2.2.2 Station flow constraints
where,
2.2.3 Reservoir water storage constraints
where,
2.2.4 Output channel constraints
where, q represents sunny weather, while y represents cloudy and rainy weather.
2.2.5 PV uncertainty constraints
where, X represents the random variable of PV power generation, μ represents the expected value of the PV power generation, σ represents the standard deviation of the PV power generation, k is a positive number, taken as 2, and P represents probability. This inequality describes the upper limit of the probability that the PV power generation deviates from its expected value by more than k times the standard deviation.
2.3 Model solution
The solving steps of the complementary system’s model for maximizing power generation and minimizing the fluctuations are as follows to fluctuations are shown in Figure 1,
Step 1: Model establishment.
The scheduling problem of hydropower-PV complementarity is formulated as a standard mathematical optimization problem using MATLAB and the YALMIP framework. This involves defining decision variables, and constraint conditions.
Step 2: Solver invocation.
The Gurobi solver is utilized for problem resolution. In YALMIP, the established mathematical optimization problem can be addressed by the Gurobi solver.
Step 3: Problem resolution.
During the resolution process, the Gurobi is utilized to solve the problem, finding the optimal values for decision variables to maximize or minimize the objective function.
Step 4: Results output.
Output results include the power station outputs, discharge flow, reservoir water levels, and other relevant data under different combined scenarios, considering the multi-objective of maximizing power generation and minimizing fluctuations in the complementary system.
3 Case analysis
3.1 Description of the case
This paper focuses on the cascade power stations in the Xiao Jin River basin and the surrounding PV power stations to validate the model and solution strategy. The Xiao Jin River is formed by the confluence of the Beiyuan Fubian River and the Dongyuan Wori River, which are tributaries of the Dadu River. The basin covers an area of 5,275 square kilometers, with a total length of 60.8 km, a drop of 472.6 m, and an average gradient of 7.8%. The main stem of the Xiao Jin River is planned for 3 levels of development, the Beiyuan Fubian River is planned for 4 levels, and the Dongyuan Wori River is planned for 3 levels of development. Except for the Meiwuo station on the Fubian River, the rest are already in operation. Among them, Mupo and Yangjiawan have daily regulation capabilities, while Menggu Bridge is a runoff power station. The last level of the Wori River, the Chunchangba station, has been put into operation with an installed capacity of 54 MW and has daily regulation capabilities. In this paper, the study focuses on the daily complementary scheduling of the Mupu, Yangjiawan, and Chunchangba hydropower stations (MHS,YHS,CHS) as a case study, with a total installed capacity of 159 MW. The main constraint conditions for the cascade power stations are detailed in Table 1.
3.2 Simualtion results
3.2.1 Scenarios generation
PV uncertainty refers to power and energy fluctuations caused by the influence of elements such as weather, environment, and equipment during the operation of solar power generation systems. Describing PV uncertainty can consider the following aspects: changes in sunlight, temperature, and equipment performance (Mao, 2014). Where changes in sunlight refer to changes in solar radiation and weather conditions, the intensity of sunlight on the PV power generation system will change. According to this situation, we can divide the PV output into three scenarios: sunny, overcast, and rainy days. Figure 2 shows the 24-h output of PV in these three scenarios. From the figure, we can see that the PV output curve shows an overall parabolic shape, with a nonlinear relationship between output power and sunlight intensity. In sunny weather, direct sunlight shines on the PV cells, resulting in high sunlight intensity and providing greater energy input, increasing the output power of PV cells. However, in overcast, rainy weather, or at night, the sunlight intensity is lower, resulting in a decrease in the output power of the PV cells (An et al., 2016). The fluctuation of sunlight intensity in different weather conditions leads to a fluctuating curve characteristic of the output power of PV cells. This is due to the instability and non-uniformity of sunlight intensity, such as factors like cloud cover or tree shadows, which can cause sudden changes in sunlight intensity, thereby affecting the output power of PV cells.
Seasons also are significant impacts on PV output. Seasonal changes can result in variations in sunlight duration and solar elevation angle. In summer, longer sunlight duration and higher solar elevation angle lead to a greater amount of energy being directly incident on the PV cells, thus providing a relatively higher output power. Conversely, in winter, shorter sunlight duration and lower solar elevation angle result in less solar radiation being received by the PV cells, leading to lower output power (Bai et al., 2018b). However, seasonal changes also affect environmental temperatures. In the summer, higher temperatures raise the working temperature of the PV cells. High temperatures can raise internal resistance and reduce electron transfer speed, lowering the conversion efficiency and output power of PV cells. In contrast, colder temperatures in the winter cause the PV cells to work at a lower temperature, increasing their efficiency and output power. The figure below compares total monthly solar radiation to the number of hours of full operation each month at a ground-based solar power station in Inner Mongolia (Li et al., 2018). As shown in Figure 3, the months with the highest total radiation are May and June, but the months with the most hours of complete operation are April and March, followed by September and October. As a result, despite June being the month with the most solar energy resources due to abnormally high temperatures, it may not be the month with the most electricity generation for PV stations all year. In reality, April is frequently the month of peak electricity generation.
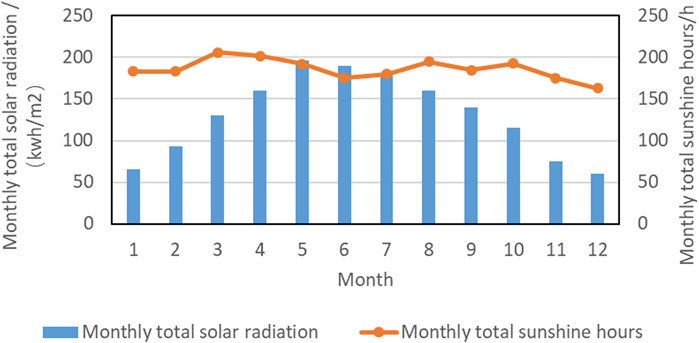
FIGURE 3. Comparison of monthly total solar radiation and monthly full sunshine hours for solar energy resources.
3.2.2 Scheduling results
3.2.2.1 Power output of complementary hydro-PV without pumping station
Figure 4 displays the complementing hydro-PV outputs in three weather scenarios: sunny, cloudy, and rainy. The figure shows that cascade hydropower and PV generating complement one other because of their resource characteristics. While PV generation is impacted by weather and sunshine intensity, hydropower generating is restricted by weather and water supplies. By operating in a complementary manner, it is possible to use PV generation to fill in the gaps when hydropower generation resources are inadequate or unstable, thereby increasing overall electricity generation. The output of PV generation exhibits significant volatility, while hydropower generation output is relatively stable (Bai et al., 2018a). By adjusting the coordinated operation strategy of hydropower and PV generation, it is possible to smooth the volatility of PV generation and reduce the overall system volatility. Cascade hydropower and PV generation systems possess a certain degree of flexibility and adjustability, allowing for the adjustment of generator output and scheduling strategies based on actual conditions. By flexibly adjusting the operation mode and output of hydropower and PV generation, it is possible to maximize the utilization of renewable energy resources and achieve the dual objectives of maximizing electricity generation and minimizing fluctuations (Chen et al., 2019).
3.2.2.2 Power output of complementary hydro-PV with pumping station
The complementary operation of cascade hydropower and PV generation can be achieved by flexibly adjusting the output of hydropower generator units to reduce curtailment of solar power, whereby the output of the hydropower units is flexibly adjusted based on the situation of PV generation to match its output (Ma et al., 2019). When the PV generation capacity is high, reducing the output of hydropower can help avoid curtailment. Conversely, when the PV generation capacity is low, increasing the output of hydropower can help compensate for the energy shortfall. However, to ensure the ecological water use of rivers and lakes, promote the green development of small hydropower, and maintain the healthy life of rivers, ecological flow releases from hydropower stations must meet certain requirements. In this context, adding a pumping station to a hydropower station allows for the flexible adjustment of the output of hydropower generator units based on the situation of PV generation. When the PV generation capacity is high, the output of hydropower can be reduced, and the excess water energy can be utilized for pumped storage; when the PV generation capacity is low, the output of hydropower can be increased to compensate for the energy shortfall (Liu et al., 2015). Therefore, to meet ecological flow release requirements while reducing curtailment of solar power under the premise of PV uncertainty, this paper studies the hydro-PV diurnal output process under sunny, cloudy, and rainy scenarios. Taking the example of the diurnal output process of hydro-PV under sunny conditions (Figure 4A), assuming the minimum output of the Chunchangba, Mupu, and Yangjiawan hydropower stations is 18, 23, and 20 MW, respectively, the addition of a pumping station to the hydropower station enables coordinated operation between PV and hydropower generation. This approach not only reduces curtailment of PV power but also satisfies the ecological flow requirements of the hydropower station.
Figure 5 shows the output of the Chunchangba, Mupu, and Yangjiawan hydropower stations. The graph illustrates that without the addition of a pumping station, the Mupu hydropower station alone can meet the minimum power generation requirements, whereas the Chunchangba and Yangjiawan hydropower stations cannot. Therefore, this paper proposes the addition of a pumping station based on the cascade hydropower station, ensuring that all three stations (Chunchangba, Mupu, and Yangjiawan) meet the minimum power generation requirements.
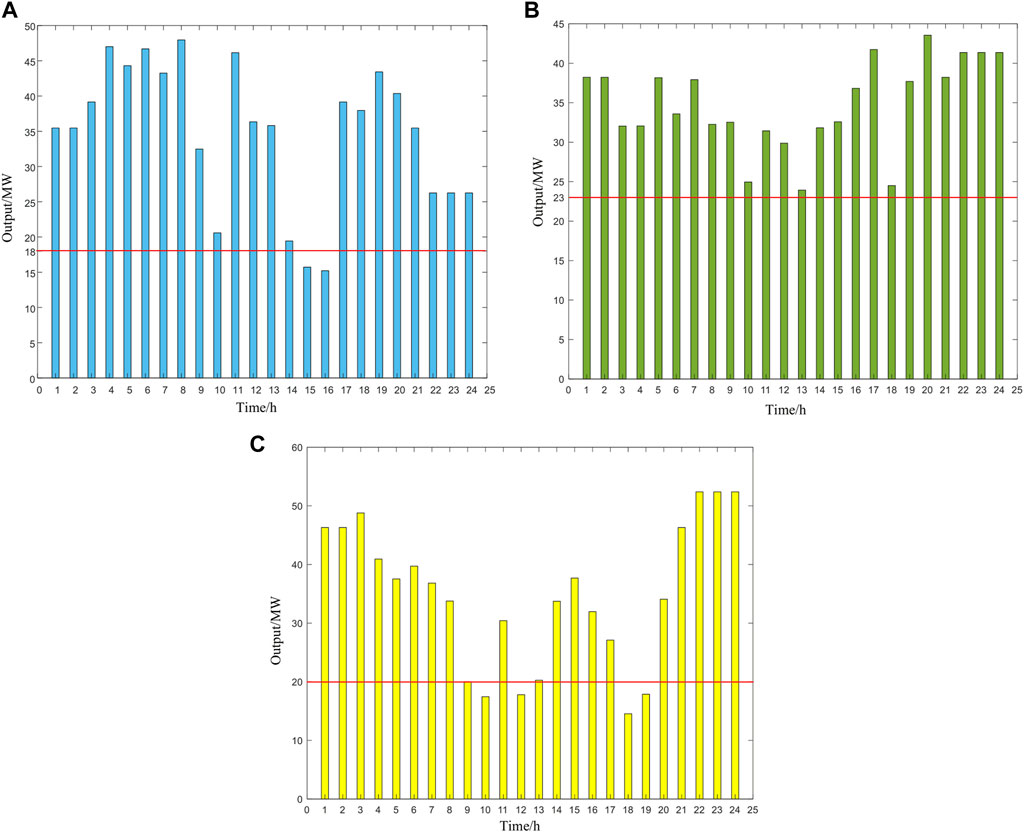
FIGURE 5. Output of Chunchangba, Mupu, and Yangjiawan Hydropower Stations (red line: Minimum Output).
In this case, a 3 MW pumping station was introduced to the hydropower station. Figure 6 displays the optimized complementary hydro-PV output and the output of the Chunchangba and Yangjiawan hydropower stations after the addition of a 3 MW pumping station. The daily PV generation utilization rate was calculated using Eq. 8. Following this procedure, the diurnal hydro-PV output processes under cloudy and rainy scenarios were analyzed, as depicted in Figures 4B, C. Subsequently, the PV generation utilization rates before and after the introduction of the pumping station were determined for sunny, cloudy, and rainy weather conditions (Table 2). The results demonstrate that the introduction of a 3 MW pumping station in the hydropower station system effectively reduces curtailment of solar power under any weather scenario, indicating that the application of pumping stations is not limited by weather conditions (Zhang and Yang, 2015). The primary role of the pumping station is to convert excess electrical energy generated by PV systems into mechanical energy and store it as potential energy in the form of water or other energy forms, thereby enhancing the overall efficiency of the PV generation system. During sunny weather, the electrical energy generated by the PV system may exceed the demand of the load, and the pumping station can transform the surplus electrical energy into stored potential energy (Zhang et al., 2018). On cloudy or rainy days, the introduction of the pumping station can compensate for the insufficient electrical energy by releasing stored potential energy, thus reducing curtailment of solar power (Wang et al., 2018). As a result, regardless of weather conditions, whether sunny, cloudy, or rainy, a 3 MW pumping station may efficiently reduce solar power curtailment while increasing overall PV generation utilization. This demonstrates the usability of pumping stations in various weather circumstances.
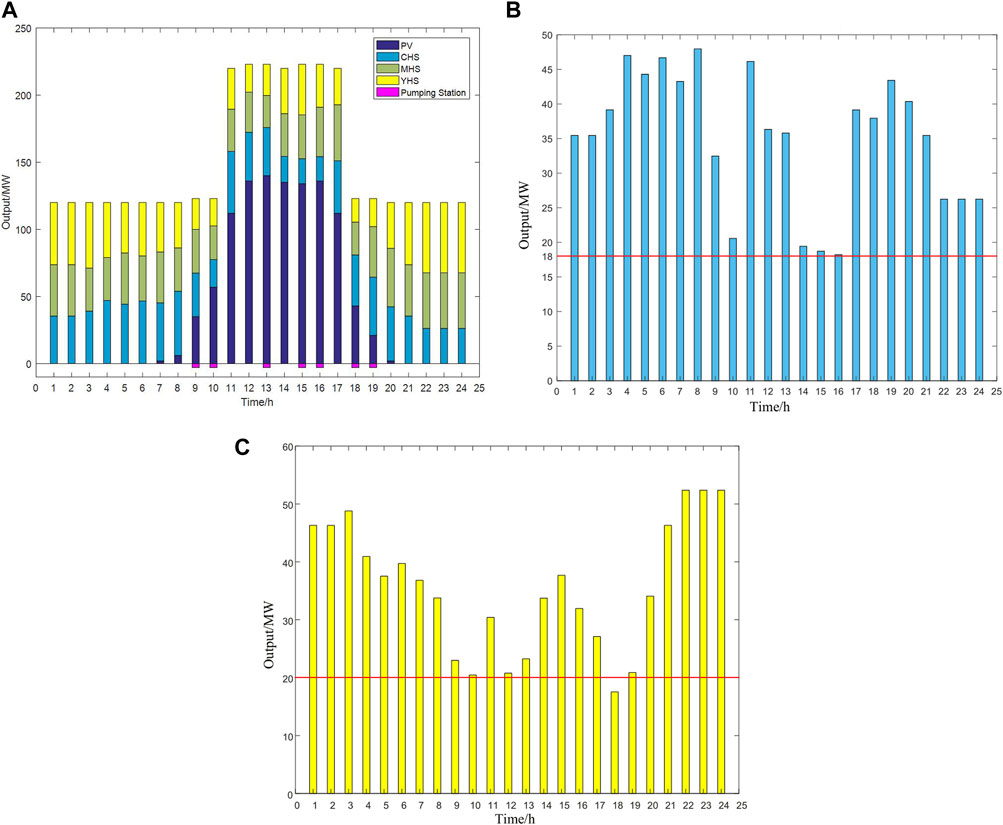
FIGURE 6. Complementary Hydro-PV Output after Introducing Pumping Stations and the Output of Chunchangba and Yangjiawan Hydropower Stations (red line: Minimum Output).
Where,
3.2.2.3 Discussion
Based on the results in Section 3.2, the following conclusions can be drawn.
(1) In the complementary operation of cascade hydropower and PV power generation systems, the introduction of a 3 MW pump station can significantly improve the utilization rate of PV power generation and effectively reduce the occurrence of curtailment.
(2) Regardless of different weather scenarios such as sunny, cloudy, or rainy days, the addition of a 3 MW pump station can increase the utilization rate of PV power generation. It can effectively mitigate the impact of PV uncertainty in any scenario, demonstrating its universal applicability.
3.2.3 Pump station capacity configuration
In a hydropower station, the capacity configuration of the pumping station must be considered to ensure the stability and efficiency of the hydraulic power generation system, and to meet the demand under different water level conditions, while maximizing operational cost savings. This study calculates the utilization rate of PV power generation for different capacity pumping stations in sunny weather scenarios and analyzes to determine the most suitable pumping station capacity.
Figure 7 shows the optimized PV power generation utilization rate after introducing different capacity pumping stations. It can be observed that introducing pumping stations of different capacities can enhance the power generation utilization rate. However, when the pumping station capacity exceeds 3 MW, the upward trend of the PV power generation utilization rate becomes more moderate. The choice of introducing a 3 MW pumping station is based on comprehensive consideration of other factors, such as economic feasibility, technical viability, and environmental impact. A 3 MW pumping station may have lower construction and operational costs compared to a 6 MW pumping station. Moreover, the implementation of a 3 MW pumping station may be more mature and stable, with easier access to relevant equipment and support systems. Additionally, from an environmental perspective, the relatively smaller scale of a 3 MW pumping station may have a smaller impact on the environment, including aspects such as land use, water resource consumption, noise, and ecological impact. By selecting a smaller-scale 3 MW pumping station, potential impacts on the local environment may be mitigated. Although introducing different pumping stations can enhance the utilization rate of PV power generation, a 3 MW pumping station is enough based on comprehensive considerations of economic, technical, and environmental factors.
4 Conclusion
In order to investigate the complementary operation of cascade hydropower and PV power generation, a multi-objective model for maximizing power generation and minimizing fluctuations was established in this paper. This model was constructed in Matlab focusing on the cascade power stations in the Xiao Jin River basin and its surrounding PV power stations. According to case analysis, the PV output was categorized into three scenarios: sunny, cloudy, and rainy days, and the following conclusions can be drawn.
(1) By considering multiple factors such as weather conditions and the characteristics of PV systems, it is possible to better predict and manage the output fluctuations of PV generation, thereby enhancing system stability and reliability. From a seasonal perspective, the months with the highest solar energy resources may not necessarily coincide with the months of highest PV generation.
(2) This study proposes the introduction of pump stations in hydropower plants to enhance the synergistic effects between hydropower and PV generation, thereby increasing the consumption capacity of PV generation and meeting the ecological flow requirements of hydropower plants.
(3) Analyzing the introduction of pump stations under different meteorological scenarios, the results demonstrate that the comprehensive utilization of PV generation can be effectively improved in all scenarios, indicating the universal applicability of pump stations.
(4) A capacity configuration analysis of pump stations was conducted. Considering factors such as economic feasibility, technical feasibility, and environmental impact, a 3 MW pump station was selected as the optimal solution. This provides important reference for the planning and construction of practical projects.
The proposed complementary operation strategy of hydro and solar power is applicable in regions with both hydroelectric and PV resources, particularly in areas with significant demand for stable energy supply, environmental sustainability, and renewable energy promotion. However, there are limitations to this approach. Firstly, this scheme is not applicable to regions that lack water and PV resources. Therefore, when selecting the implementation of this scheme, it is necessary to fully consider the resource conditions and feasibility of the specific region. Secondly, the introduction of pump stations requires significant financial investment and can have certain impacts on the local ecological environment. The construction and operation of pump stations may have effects on the local ecological environment, such as variations in reservoir water levels that could disrupt the balance of surrounding ecosystems. Therefore, a comprehensive evaluation should be conducted when introducing pump stations, along with the implementation of appropriate environmental protection measures, to minimize adverse impacts on the ecological environment. Furthermore, the feasibility of this scheme also requires specific research and evaluations in different scenarios. Due to variations in resource conditions, climatic characteristics, and energy demands across different regions, the feasibility and effectiveness of this scheme may vary. In summary, further research and specific on-site evaluations are necessary to determine the applicability and potential limitations of this scheme in different environments. This will contribute to a better understanding of the feasibility of this scheme and provide scientific foundations and reasonable guidance for its implementation.
Data availability statement
The original contributions presented in the study are included in the article/Supplementary material, further inquiries can be directed to the corresponding author.
Author contributions
HL: Conceptualization, Data curation, Formal Analysis, Writing–original draft, Writing–review and editing. SL: Supervision, Writing–review and editing.
Funding
The author(s) declare financial support was received for the research, authorship, and/or publication of this article. This research was funded by the authors gratefully acknowledge the financial support of the project National Natural Science Foundation of China (No. U23B20140), National Natural Science Foundation of China (No. U22B20112) and Supported by the Fundamental Research Funds for the Central Universities (No. 423168).
Acknowledgments
First and foremost, I would like to express my gratitude to every author who has worked with me on this paper. Everyone plays to their strengths and makes useful suggestions.
Conflict of interest
The authors declare that the research was conducted in the absence of any commercial or financial relationships that could be construed as a potential conflict of interest.
Publisher’s note
All claims expressed in this article are solely those of the authors and do not necessarily represent those of their affiliated organizations, or those of the publisher, the editors and the reviewers. Any product that may be evaluated in this article, or claim that may be made by its manufacturer, is not guaranteed or endorsed by the publisher.
References
An, Y., Fang, W., and Huang, Q. (2016). Preliminary research of theory and method of hydro/solar complementary operation. Acta Energiae Solaris Sin. 37 (8), 1985–1992.
Bai, K., Gu, J., and Peng, H. (2018a). Optimal allocation for multi-energy complementary microgrid based on scenario generation of wind power and photovoltaic output. Automation Electr. Power Syst. 42 (15), 133–141.
Bai, K., Gu, J., and Peng, H. (2018b). Optimal configuration of multi-energy complementary microgrid system generated by wind and solar output scenarios. Automation Electr. Power Syst. 42 (15), 133–141.
Central Committee of the Communist Party of China and the State Council (2021). Opinions on fully, accurately, and comprehensively implementing the new development concept and effectively carrying out the work of peaking carbon emissions and achieving carbon neutrality [EB/OL].
Chen, P., Wei, F., and Sun, K. (2019). Multi-energy storage system and islanded optimal dispatch method of CCHP. Trans. China Electrotech. Soc. 34 (15), 3231–3243.
Ding, M., Wang, W., Wang, X., Song, Y., Chen, D., Sun, M., et al. (2014). Overview of the impact of large-scale photovoltaic power generation on power system. Chin. J. Electr. Eng. 01, 1–14.
Hongyang, W., Yao, L., and Zhang, K. (2016). Research on operation strategy of hydro-solar complementary power generation. Shandong Electr. Power Technol. 43 (4), 43–45.
Huang, X., Wu, H., and Li, M. (2021). Study on key factors affecting power supply performance of outdoor photovoltaic power. High. Volt. Appar. 57 (5), 36–42.
Li, J., Guo, B., and Niu, M. (2018). Optimal configuration strategy of energy storage capacity in wind/PV/storage hybrid system. Trans. China Electrotech. Soc. 33 (6), 1189–1196.
Liu, D., Chen, S., Ma, M., Wang, H., Hou, J., Ma, S., et al. (2011). Power Syst. Technol. 08, 47–52.
Liu, J., Wang, S., and Wang, M. (2015). Influence analysis of hydro-photovoltaic power complementary system of Longyangxia hydro-power station. Adv. Power Syst. Hydroelectr. Eng. 31 (9), 83–87.
Liu, S., Wang, J., and Li, Q. (2021). Optimal configuration of multi-energy complementary composite power station and its application in system power supply planning. Power Syst. Technol. 45 (8), 3006–3015.
Ma, W., Wang, W., and Wu, X. (2019). Optimal dispatching strategy of hybrid energy storage system for smoothing power fluctuation caused by grid-connected photovoltaic. Automation Electr. Power Syst. 43 (3), 58–69.
Mao, W. (2014). Analysis of active power characteristics of photovoltaic power station and related software development. Wuhan: Huazhong University of Science and Technology.
Ming, B., Liu, P., Cheng, L., Zhou, Y., and Wang, X. (2018). Optimal daily generation scheduling of large hydro–photovoltaic hybrid power plants. Energy Convers. Manag. 171, 528–540. doi:10.1016/j.enconman.2018.06.001
National Energy Administration (2022). Action plan for peaking energy carbon and promoting carbon neutrality and standardization. Popular Util. Electr. 37 (11), 8–10.
Pang, X., and Zhang, W. (2017). Research and application of hydroporwer-PV complementary technology. J. Hydroelectr. Eng. 36 (7), 1–13.
Shen, J., Wang, Y., and Cheng, C. (2022). Research status and prospect of power generation dispatching of hydropower-wind-solar hybrid system. Proc. CSEE 42 (11), 3871–3885.
Solar (2015). In 2015, China's total installed capacity of photovoltaic power generation ranked first in the world [EB/OL]. Available at: http://solar.ofweek.com/2016-02/ART-260009-8420-29064342.html.
Song, Ke (2021). Optimal scheduling of hydro-photovoltaic complementary generation system based on scene feature clustering. Hydropower Energy Sci. 39 (6), 202–205.
State Council of the People's Republic of China (2021). Xi jinping chairs the ninth meeting of the central financial and economic commission[EB/OL]. Available at: http://www.gov.cn/xinwen/2021-03/15/content_5593154.html.
Wang, X., Chang, J., Meng, X., and Wang, Y. (2018). Short-term hydro-thermal-wind-photovoltaic complementary operation of interconnected power systems. Appl. Energy 229, 945–962. doi:10.1016/j.apenergy.2018.08.034
Wang, X., Edgar, V., Jordan, K., Chen, L., Mei, Y., Patiño-Echeverri, D., et al. (2019). Integrating wind, photovoltaic, and large hydropower during the reservoir refilling period. Energy Convers. Manag. 198, 111778. doi:10.1016/j.enconman.2019.111778
Yang, Z., Liu, P., Cheng, L., Liu, D., Ming, B., Li, H., et al. (2021). Sizing utility-scale photovoltaic power generation for integration into a hydropower plant considering the effects of climate change: a case study in the Longyangxia of China. Energy 236, 121519. doi:10.1016/j.energy.2021.121519
Zhang, P., and Yang, T. (2015). Research on Longyangxia hydro-photovoltaic complementary operation mechanism. J. North China Univ. Water Resour. Electr. Power Nat. Sci. 36 (3), 76–81.
Zhang, X., Ma, G., Huang, W., Chen, S., and Zhang, S. (2018). Short-term optimal operation of a wind-PV-hydro complementary installation: yalong River, Sichuan Province, China. Energies 11 (4), 868. doi:10.3390/en11040868
Zhang, Y., Chun, M., Lian, J., Pang, X., Qiao, Y., and Chaima, E. (2019). Optimal photovoltaic capacity of large-scale hydro-photovoltaic complementary systems considering electricity delivery demand and reservoir characteristics. Energy Convers. Manag. 195, 597–608. doi:10.1016/j.enconman.2019.05.036
Keywords: hydroporwer-PV complementary, multi-target, pumping stations, short-term dispatching, PV utilization
Citation: Hanxuan L, Linjun S, Hao F and Feng W (2024) Short-term optimization scheduling method of cascade hydropower and photovoltaic complementary system based on pumping station operation strategy. Front. Energy Res. 12:1367135. doi: 10.3389/fenrg.2024.1367135
Received: 08 January 2024; Accepted: 01 February 2024;
Published: 28 February 2024.
Edited by:
Haifeng Qiu, Nanyang Technological University, SingaporeReviewed by:
Jicheng Fang, State Grid Jiangsu Electric Power Co., LTD., ChinaYongli Ji, Nanjing Institute of Technology (NJIT), China
Copyright © 2024 Hanxuan, Linjun, Hao and Feng. This is an open-access article distributed under the terms of the Creative Commons Attribution License (CC BY). The use, distribution or reproduction in other forums is permitted, provided the original author(s) and the copyright owner(s) are credited and that the original publication in this journal is cited, in accordance with accepted academic practice. No use, distribution or reproduction is permitted which does not comply with these terms.
*Correspondence: Shi Linjun, ZWVjQGhodS5lZHUuY24=