- School of Chemistry and Chemical Engineering, Southwest Petroleum University, Chengdu, Sichuan, China
The study investigated the effects and mechanisms of clay content, emulsion water content, pH, and metal cations on clay-crude oil emulsions. The results indicate the following: 1) At a water content of 50 V/V%, montmorillonite can form emulsions with crude oil at different concentrations, with the highest stability observed at 5 wt% content. In contrast, chlorite, illite, and kaolinite cannot form emulsions at low concentrations. 2) Under acidic conditions, montmorillonite, illite, and chlorite cannot form emulsions with crude oil, or the emulsions are highly unstable. However, kaolinite forms more stable emulsions under acidic conditions. In alkaline environments, emulsions formed by all clay minerals exhibit increased stability. 3) The order of the effectiveness of different metal cations in reducing the stability of montmorillonite-crude oil emulsions is K+ > Na+ > Mg2+ > Ca2+, while for chlorite, illite, and kaolinite, it is Mg2+ > Ca2+ > K+ > Na+. 4) The factors that influence the stability of clay-crude oil emulsions are the arrangement of clay particles in water and the dispersion capability of clay particles in water. The most significant influencing factor is the arrangement of clay particles in water.
1 Introduction
Currently, global energy consumption has reached a historic peak, triggering significant transformations in global energy trade and supply-demand dynamics. The international energy price system exhibits volatility, with the preservation of energy supply security now constituting the foremost objective in nations reliant on energy imports. Currently, the majority of initially developed oil fields have advanced into intermediate and late developmental stages. Effectively extracting the remaining oil from these reservoirs represents the primary technical challenge in the current context. To meet the escalating global demand for oil, enhancing the oil recovery rate is of paramount importance. Primary and secondary oil recovery technologies can recover only 30%–40% of the total crude oil reserves. Consequently, tertiary oil recovery technology, capable of extracting 60%–70% of the remaining crude oil in reservoirs, has garnered global attention (Janssen et al., 2019; Liu et al., 2020).
Numerous factors contribute to the formation of emulsions between crude oil and water, primarily categorized into two aspects: 1. During oil and water extraction and transportation, turbulence and agitation occur in locations like pores, pipelines, and pumps, leading to thorough mixing and emulsion formation. 2. Interfacially active substances are present in the crude oil during extraction, comprising both artificially introduced compounds and naturally occurring reservoir components (He et al., 2022; Zhang et al., 2023). These active substances encompass surfactants, binary systems, and alkalis. The artificially introduced ones consist of surfactants and bases, while the reservoir hosts natural interfacially active substances like waxes, asphaltenes, colloids, clays, and microorganisms. Crude oil emulsion constitutes a thermodynamically unstable multiphase dispersion system. When liquid is dispersed into droplets, it escalates the interfacial area between the two liquids, leading to increased interfacial free energy. This renders the emulsion thermodynamically unstable. However, interfacially active substances can adsorb at the oil-water interface, significantly reducing the interfacial tension between oil and water, thereby stabilizing the entire system (Sztukowski and Yarranton, 2005; Liu et al., 2019).
Pickering discovered that solid particles can effectively stabilize emulsions. Solid particles possessing surface activity adhere to the oil-water interface, forming a dense film that enhances the stability of the oil-water emulsion. These solid particles in Pickering emulsions present an excellent alternative to traditional surfactants, offering superior thermal stability, cost-effectiveness, and environmental friendliness. Presently, Pickering emulsion systems find application across diverse fields. Given their expansive oil-water interface, these systems offer an ideal platform for interfacial catalysis (Crossley et al., 2010). Moreover, due to their non-irritating nature, Pickering emulsions are employed in pharmaceuticals for drug delivery (Oka et al., 2015; Ana Maria Bago Rodriguez, 2020).
Currently, within the petroleum industry, Pickering emulsions have garnered considerable attention from experts (Liu et al., 2021). Their exceptional stability under high-temperature and high-pressure conditions positions Pickering emulsion systems as a promising avenue for enhancing crude oil recovery (Hu et al., 2023). Furthermore, Pickering emulsions have been investigated for their potential in drilling fluids, as the inclusion of solid particles can augment fluid viscosity (Son et al., 2015; Zhang et al., 2015).
"Clay" serves as a collective term encompassing various minerals, predominantly characterized by a 1:1 or 1:2 ratio of silicon dioxide tetrahedra to aluminum oxide octahedra (Uddin, 2008). Reservoirs commonly host clay minerals, including montmorillonite, kaolinite, illite, and chlorite, with particle sizes reaching several tens of micrometers. Clay minerals within the formation undergo expansion upon water absorption, leading to the formation of smaller layers, sometimes as minute as the nanometer scale. During this stage, clay particles exhibit a significantly larger specific surface area, enabling their adsorption at the oil-water interface, thereby contributing to emulsion stabilization (Abend and Lagaly, 2001).
Most oil reservoirs contain a significant clay content, ranging from 20% to 50% (Kassab et al., 2021; Hua et al., 2022). During the oil recovery process, the increasing clay content in these reservoirs presents substantial challenges for demulsifiers, resulting in elevated oilfield expenses and extended operational periods. Although certain studies have examined the effects of bentonite and kaolinite on crude oil emulsions, no comprehensive investigations have explored the impacts of montmorillonite, kaolinite, chlorite, and illite on emulsion formation and stability (Abend and Lagaly, 2001; Zhu and Wang, 2022). Consequently, this study offers a detailed analysis of the influence of these four distinct clay types on emulsion formation and stability under varying conditions.
2 Materials and methods
2.1 Materials
2.1.1 Chemicals
Crude oil, produced from the Xinjiang oilfield in China, Specific parameters of crude oil are detailed in Table 1. Montmorillonite, illite, kaolinite, and chlorite are produced in Hebei Province, China. Sodium hydroxide (NaOH, AR, ≥97%, Shanghai Aladdin Biochemical. Technology Co., Ltd., China). Hydrochloric acid solution (37%, Shanghai Aladdin Biochemical Technology Co., Ltd., China). Sodium chloride (NaCl, AR, 99.5%, Shanghai Aladdin Biochemical Technology Co., Ltd., China). Potassium chloride (KCl, AR, 99.5%, Shanghai Aladdin Biochemical Technology Co., Ltd., China). Magnesium chloride hexahydrate (MgCl2 · 6H2O, AR, 99%, Shanghai Aladdin Biochemical Technology Co., Ltd., China). Calcium chloride (CaCl2, AR, 99.5%, Shanghai Aladdin Biochemical Technology Co., Ltd., China).
2.1.2 Instruments
52–01005 A high-definition video microscope (Bresser, Germany). SF750 high-speed mixer (Chengdu Lichen Technology Co., Ltd., China). Zetaprobe Zeta Potentiometer (Brookhaven Instruments, United States).
2.2 Methods
2.2.1 Preparation of clay-crude oil emulsions
The clay was introduced into water and agitated vigorously for 5 min at 10,000 rpm using a high-speed mixer to ensure the uniform dispersion of clay particles in the water, resulting in the formation of a clay dispersion liquid. In this clay dispersion, crude oil was added and stirred at 10,000 rpm for 5 min, achieving the homogeneous dispersion of crude oil within the clay dispersion to create an emulsion. This study involved the preparation of clay-crude oil emulsions under various conditions, including different types of clay, varying clay concentrations, water content, pH levels, and distinct metal cations. The specific experimental setups are detailed below:
(1) Clay-crude oil emulsions with varying clay and water contents: The clay minerals used are montmorillonite, illite, kaolinite, and chlorite, with each mineral’s content in the emulsion system set at 0.5 wt%, 1 wt%, 3 wt%, and 5 wt%. The emulsions are prepared with water contents of 50 V/V% and 30 V/V%.
(2) Clay-crude oil emulsion under diverse pH conditions: Deionized water’s pH is adjusted using NaOH and HCl to levels of 2, 4, 7, 9, and 11. A clay dispersion is formed for montmorillonite, illite, chlorite, and kaolinite, each paired with crude oil, resulting in a clay-crude oil emulsion with each clay mineral’s content at 5 wt% and water content set at 50 V/V%."
(3) Clay-crude oil emulsion with various metal cations: Deionized water was supplemented with NaCl, KCl, MgCl2-H2O, and CaCl2, with the brine concentration in all configurations set at 0.5 mol/L. Clay dispersions of montmorillonite, illite, chlorite, and kaolinite were then prepared to create clay-crude oil emulsions in conjunction with crude oil. The clay mineral contents in all emulsions were 5 wt%, and the water contents were 50 V/V%.
2.2.2 Observation of emulsion stability
The stability of the emulsion was assessed using a bottle experiment. The prepared emulsion was placed into a sealed glass bottle with a 50 mL capacity and an 11 cm height. The layering condition of the emulsion was observed at various time intervals. The experimental temperature is 25°C.
2.2.3 Microscopic observation of emulsion morphology
The microscopic morphology of the emulsion was examined using a high-definition microscope. A prepared emulsion was dispensed drop by drop onto a glass slide using a rubber-tipped dropper. To facilitate optimal light transmission through the emulsion, the emulsion was evenly spread on the glass slide with a cell spatula. Subsequently, the glass slide was positioned on the microscope stage for observation. The experimental temperature is 25°C.
2.2.4 Zeta potential test
A zeta potential meter was employed to assess the zeta potential of the clay dispersion under various conditions, with a clay particle concentration of 0.1 kg/L. Each sample underwent five parallel experiments to ensure data accuracy. The experimental temperature is 25°C.
2.2.5 Rheological properties
Using a rotational rheometer, the rheological properties of clay dispersion liquids were measured, including the viscosity of clay dispersion liquids and the variation of shear stress with shear rate. The shear rate range was set from 2 s−1 to 40 s−1, at a temperature of 25°C. In this study, we tested the rheological properties of four types of clay dispersion liquids under different pH conditions and concentrations. The experimental temperature is 25°C.
2.2.6 Particle size distribution test
In this study, the particle size distribution of clay particles in the clay dispersion solution and the droplets in the emulsion will be tested. The testing methods for the two samples are different. In the clay dispersion solution, only clay particles are present as the dispersed phase, so testing can be directly conducted using a laser particle size analyzer. However, in the emulsion, clay particles coexist with droplets, thus requiring the use of image processing software to statistically analyze the droplet size distribution of the emulsion. The specific procedures are as follows.
2.2.6.1 Particle size distribution test of clay dispersion solution
Using a laser particle size analyzer to determine the particle size of clay dispersion liquids, a certain concentration of clay dispersion liquid was prepared and placed in the sample chamber to measure the particle size distribution of the clay dispersion liquid. We conducted particle size distribution tests for clay minerals at concentrations of 0.5 wt% and 5 wt%. The experimental temperature is 25°C.
2.2.6.2 Particle size distribution test of emulsion
Photograph the prepared emulsion using a high-resolution microscope, process the images using Nano Measurer software, and obtain the droplet size distribution of the emulsion.
3 Results and discussion
3.1 Effect of clay content and water content on clay-crude oil emulsion
3.1.1 Effect of clay content on clay-crude oil emulsion
Under pH = 7 conditions, montmorillonite dispersions of various concentrations exhibited the ability to form emulsions with crude oil. However, when the montmorillonite content was below 3 wt%, emulsions tended to separate rapidly. At montmorillonite contents of 3 wt% and 5 wt%, the emulsions remained highly stable, the system viscosity increased, and no delamination occurred even after 24 h. Conversely, kaolinite, illite, and chlorite only formed emulsions at 5 wt% and experienced relatively rapid delamination (Figure 1). The particle size distribution and viscosity variations of clay dispersion liquids for four types of clay were tested at different clay concentrations. As the clay content increased, the distance between clay particles decreased, making them more prone to aggregation and forming larger particles. Simultaneously, due to the closer arrangement of clay particles, the interaction forces between particles increased, leading to an increase in the viscosity of the clay dispersion liquid. When the clay particle concentration is 0.5 wt%, the particle size of clay particles mainly ranges from 1 to 10 μm. When the concentration increases to 5 wt%, the clay particle size increases to 1–100 μm (Figure 2), the viscosity of the clay dispersion increases with the increase in clay content (Figure 3). The increased viscosity of the clay dispersion liquid hinders the displacement of emulsion droplets in the system, thereby impeding the aggregation and breakup of droplets, resulting in a more stable emulsion.
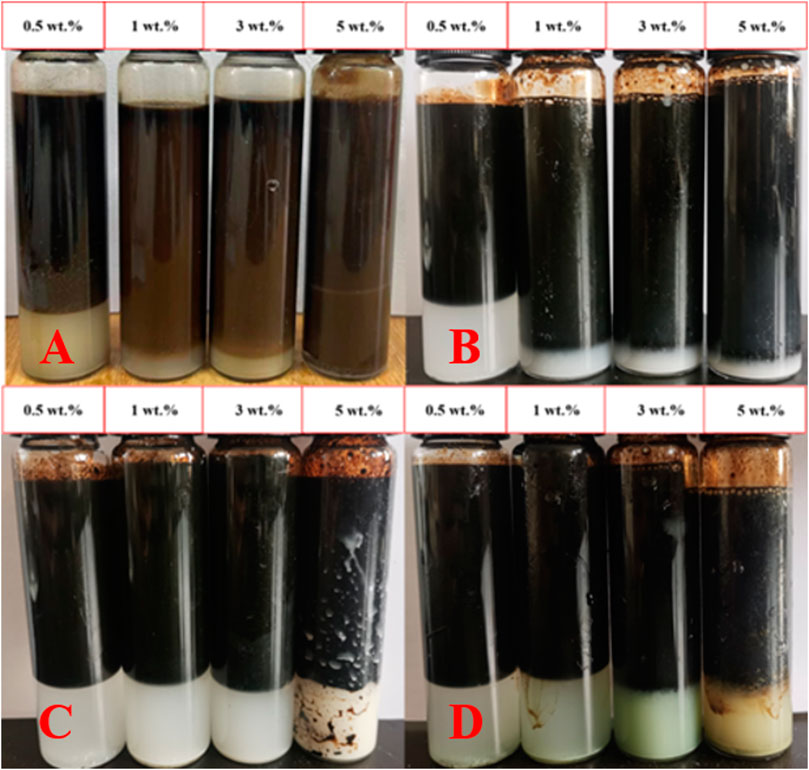
Figure 1. After 24 h, stratification of emulsions at different concentrations of clay. (A) Montmorillonite. (B) Kaolinite. (C) Illite. (D) Chlorite.
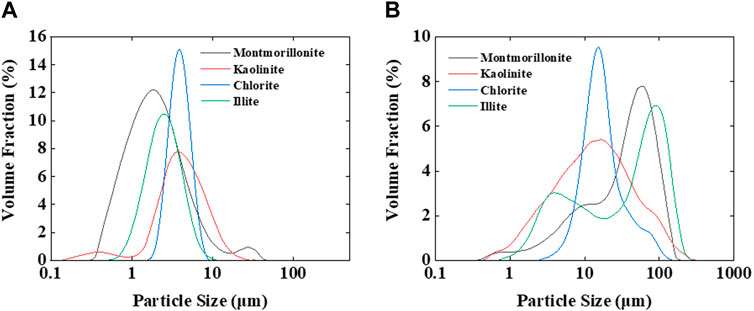
Figure 2. (A) Particle size distribution at a clay concentration of 0.5 wt%. (B) Particle size distribution at a clay concentration of 5 wt%.
After 24 h of observation, the emulsion displayed layering, segregating into four parts from top to bottom:
(1) Oil sludge layer: This layer, devoid of emulsion droplets, forms due to the mixture of crude oil and clay particles. It possesses high viscosity and limited fluidity. The formation of this part is due to the ascent of oil droplets and the carrying of clay particles when the emulsion breaks.
(2) Oil droplet layer: Primarily consisting of unbroken oil droplets that ascend and aggregate under buoyancy, signifying that even in a stratified emulsion system, clay particles effectively stabilize the oil-water interface, resulting in numerous unbroken oil droplets.
(3) Clay dispersion layer: This layer is devoid of crude oil and contains only a small number of dispersed clay particles.
(4) Clay accumulation layer: A substantial accumulation of clay particles precipitates and gathers at the bottom.
The microstructure of the montmorillonite-crude oil emulsion system at various montmorillonite contents is illustrated in Fig. With increasing montmorillonite content, the clay particles in the aqueous phase between oil droplets progressively rise. Ultimately, when montmorillonite content reaches 5 wt%, the aqueous phase between the oil droplets is almost entirely filled with montmorillonite particles (Figure 4). Due to the increase in clay particle content, it becomes difficult for the emulsion droplets to aggregate with each other, resulting in a decrease in the diameter of the formed emulsion droplets (Figure 5). At the same concentration, the water phase between oil drops in the kaolinite, illite, and chlorite dispersions is also filled with a large number of clay particles.
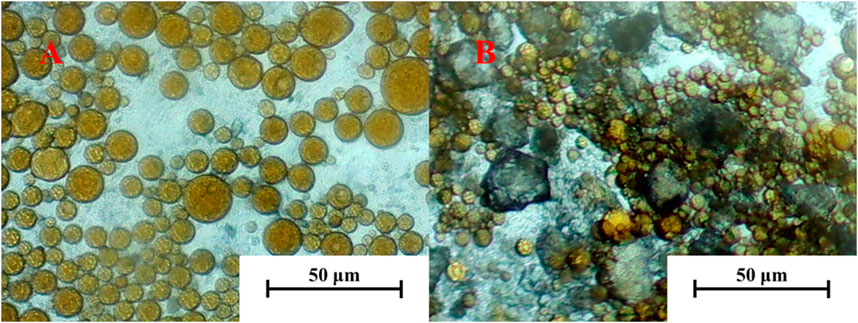
Figure 4. Microscopic morphology of emulsion under different montmorillonite contents. (A) Microscopic morphology of emulsion with a clay content of 0.5 wt%, no obvious clay particles between oil droplets. (B) Microscopic morphology of emulsion with a clay content of 5 wt%, the oil droplets are filled with clay particles between them.
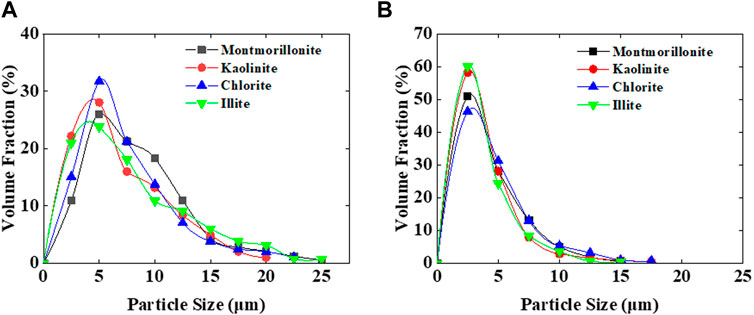
Figure 5. Microscopic morphology of emulsion under different montmorillonite contents. (A) The droplet diameter distribution of the emulsion at clay content of 0.5 wt%. (B) The droplet diameter distribution of the emulsion at clay content of 5 wt%.
Montmorillonite exhibits a higher propensity to form emulsions compared to the other three clays due to two primary factors:
(1) Its superior water absorption and swelling properties;
(2) The unique arrangement of montmorillonite particles in water (Johnston et al., 1992).
Among the four clay minerals, only montmorillonite qualifies as an expansive clay, while the other three are non-expansive clays. Weak interlayer bonding in montmorillonite crystals facilitates extensive hydration, allowing water molecules to penetrate the crystal interlayer. Consequently, the particles disperse more evenly in water, maintain a smaller size, and are less prone to aggregation. In contrast, illite interlayers contain K+ ions with strong bonding, chlorite interlayers are rich in hydroxides with hydrogen bonding, and kaolinite lacks exchangeable cations in its interlayer, preventing water molecules from penetrating. Therefore, only montmorillonite among the four clay minerals undergoes interlayer hydration and exhibits superior swelling properties.
Clay particles in water can be categorized into three arrangements: face-face (F-F), face-edge (F-E), and edge-edge (E-E). Montmorillonite particles in water are arranged in a F-E configuration, while particles of kaolinite, illite, and chlorite adopt a F-F arrangement (Figure 6). Oil droplets in montmorillonite-based emulsions within the F-E arrangement are sandwiched between oil droplets in the aqueous phase, providing a stable structure that minimizes emulsion disruption.
It should provide a concise and precise description of the experimental results, their interpretation, as well as the experimental conclusions that can be drawn.
3.1.2 Effect of water content on clay-crude oil emulsion
Montmorillonite can create emulsions at various concentrations when subjected to 30 V/V% water content. However, stratification was notably swifter when the montmorillonite content was 0.5 wt%, 1 wt%, and 5 wt%. No stratification was observed after 24 h with a 3 wt% montmorillonite content. The most rapid stratification occurred in emulsions containing 5 wt% montmorillonite, leading to the rapid settlement of a significant quantity of white montmorillonite particles at the bottom. Conversely, kaolinite, illite, and chlorite did not yield emulsions when combined with crude oil at varying concentrations.
A reduction in water content, while keeping clay content constant, leads to a decrease in the available water molecules for expanding clay minerals. Consequently, clay minerals become less prone to expansion. During this phase, the aqueous phase predominantly contains large clay particles that cannot be adsorbed at the oil-water interface. This results in an accelerated settling rate, making it impossible to establish a stable emulsion (Abbas, 2021).
3.2 Effect of pH on clay-crude oil emulsion
Under alkaline conditions, all four clay minerals can disperse and create emulsions with crude oil. However, the emulsion system formed by kaolinite, chlorite, and illite dispersions exhibits a rapid delamination rate, in contrast to the highly stable montmorillonite-crude oil emulsion, which shows no delamination even after 24 h. In acidic conditions, an anomaly arises: montmorillonite dispersion can indeed form an emulsion with crude oil, but delamination occurs at a faster pace. Illite and chlorite dispersions fail to establish stable emulsions with crude oil under acidic conditions. Surprisingly, kaolinite can form a stable emulsion with crude oil under acidic conditions, and the system viscosity gradually increases (Figure 7).
The hydroxyl groups exposed on the clay surface tend to decompose. At higher pH levels, there is a greater influx of H+ ions into the water, leading to an increase in the negative charge on the clay surface. This results in an increased absolute value of ζ-potential, strengthening the electrostatic repulsion between clay particles. As a result, it becomes challenging for clay dispersions to aggregate under alkaline conditions. When the pH falls below 7, the water’s H+ concentration rises, leading to more H+ ions interacting with the clay particle surfaces for potential neutralization. During this phase, the absolute value of the clay particles' zeta-potential decreases, weakening the electrostatic repulsion between particles and making them more prone to aggregation and settling (Figure 8) (Arroyo and Olicares, 2000; Fabrice et al., 2013).
Rheological tests were conducted on kaolinite dispersion liquids at different pH levels. When pH > 7, kaolinite dispersion liquids exhibited shear-thinning behavior, with viscosity increasing as pH rose (Figure 9), accompanied by the development of yield strength. In contrast, the viscosity of dispersion liquids for the other three clay minerals did not vary with changes in pH (Figure 10). The charge at the edges of kaolinite primarily arises from the dissociation of hydroxyl groups. In neutral and alkaline settings, both the bottom surfaces and edges of kaolinite mineral particles carry a negative charge, resulting in an F-F arrangement between particles. However, in an acidic environment, the edge surface of kaolinite becomes positively charged while the bottom surface retains a negative charge. Consequently, the arrangement between kaolinite particles shifts to an F-E arrangement, akin to that of montmorillonite, leading to an increase in slurry viscosity. As a result, the kaolinite’s arrangement structure in the aqueous phase between oil droplets under acidic conditions plays a stabilizing role, enhancing the overall stability of the emulsion system (Figure 6) (Chorover and Sposito, 1995; Huertas et al., 1999).
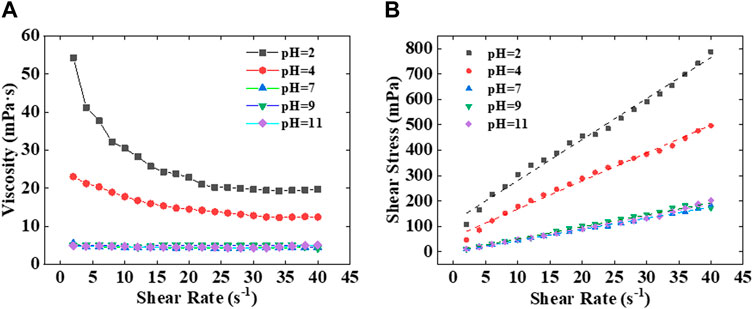
Figure 9. (A) Variation of viscosity of kaolinite dispersion liquids with shear rate at different pH levels. (B) Variation of shear stress with shear rate in kaolinite dispersion liquids at different pH levels.
3.3 Effect of metal cations on clay-crude oil emulsion
The introduction of metal cations will reduce the stability of different clay crude oil emulsion systems. The order of decreasing degree of stability of kaolinite, illite and chlorite emulsion system by different cations is: Mg2+>Ca2+>K+>Na+. In contrast, for montmorillonite, the order is K+ > Na+ > Mg2+ > Ca2+. In the presence of K+, montmorillonite dispersion struggles to form emulsions with crude oil, while in the presence of Na+, the emulsion formed by montmorillonite dispersion becomes exceedingly unstable, leading to rapid emulsion breakdown and the emergence of visible, large-sized oil droplets (Jiang et al., 2014).
The impact of these four metal ions on kaolinite, illite, and chlorite results from the adsorption of metal ions onto clay particle surfaces. This is a consequence of the negatively charged surfaces of clay minerals, which attract positively charged metal ions. As a result, metal ions compress the diffuse bilayer on clay particle surfaces, reducing electrostatic repulsion between particles. The hydration capacity of clay particle surfaces greatly exceeds that of metal ions. Consequently, when metal cations adsorb to clay particle surfaces, it leads to a decrease in the thickness of the hydration film on clay particle surfaces and, consequently, a reduction in repulsive forces between particles. Moreover, the higher the valence number of the metal cation, the more significant the decrease in the hydration capacity of clay particles. The mechanism for the impact of Mg2+ and Ca2+ on montmorillonite is analogous (Gui et al., 2016).
However, the influence of Na+ and K+ on montmorillonite extends beyond the inhibition of surface hydration. Both Na+ and K+ have the capacity to permeate the montmorillonite crystal layers, displacing interlayer water molecules. This process results in a reduction in the distance between crystal layers, intensifying their effects. Notably, K+ exhibits a more potent inhibitory impact on montmorillonite hydration compared to Na+. This discrepancy arises from the lower hydration energy of K+ (393 kJ/mol) compared to the other three metal cations, rendering it more prone to adsorption by montmorillonite (Ren et al., 2021). Once inside the interlayer, K+ remains unhydrated, and its smaller radius in relation to the crystal layer spacing prompts it to generate binding forces with both sides of the crystal layers, causing the accumulation of more layers and, ultimately, agglomeration and precipitation (Figure 11).
While chlorite, illite, and montmorillonite share the classification of 2:1 minerals, chlorite possesses a unique feature with a layer of hydroxide (either magnesium hydroxide or aluminum hydroxide) nestled between its crystal layers. This hydroxide replaces the interlayer cations of chlorite, leading to hydrogen bonding between the chlorite crystal layer and the hydroxide. In illite, a substantial quantity of K+ naturally resides within the crystal layer itself. Consequently, it is challenging for Na+ and K+ to penetrate the interlayer between chlorite and illite, limiting their actions to the particle surfaces. In contrast, kaolinite exhibits a smaller surface charge density and thinner surface hydration layer compared to the other three clay minerals, resulting in a more compact interlayer structure (Xuan et al., 2013; Jiang et al., 2014). Consequently, Na+ and K+ face difficulty entering the kaolinite crystal layer. Hence, the four metal ions, Na+, K+, Mg2+, and Ca2+, primarily exert their influence on the surfaces of kaolinite, illite, and chlorite particles, with the degree of hydration inhibition becoming more pronounced as the valence of the metal cation increases.
4 Conclusion
(1) Among the four clay minerals, montmorillonite, illite, chlorite, and kaolinite, only montmorillonite dispersion yields the most stable emulsion. This is attributed to montmorillonite’s superior water dispersion properties and the F-E arrangement between its particles, which enhances emulsion stability.
(2) The absolute zeta potential of montmorillonite, illite, chlorite, and kaolinite exhibits an increase with rising pH levels, resulting in enhanced dispersion in water. In acidic conditions, kaolinite-crude oil emulsions gain increased stability due to the alteration of kaolinite’s particle arrangement from F-F to F-E. Conversely, montmorillonite, illite, and chlorite become more prone to agglomeration and sedimentation under acidic conditions, stemming from a reduction in the absolute value of the zeta potential and the ensuing decrease in electrostatic repulsion between particles. Consequently, the emulsions disintegrate swiftly, and in some cases, fail to form emulsions at all.
(3) The order of magnitude concerning the influence of different metal cations on the reduction of stability in montmorillonite-crude oil emulsion is as follows: K+ > Na+ > Mg2+ > Ca2+. In contrast, for chlorite, illite, and kaolinite, the order is Mg2+ > Ca2+ > K+ > Na+. This discrepancy arises from the capacity of Na+ and K+ to not only inhibit montmorillonite hydration on its particle surfaces and compress the bilayer but also their ability to penetrate the montmorillonite crystal interlayer, enhancing interlayer forces and promoting montmorillonite agglomeration in the presence of these two ions. However, due to the distinct structures of clay minerals, Na+ and K+ are unable to enter the interlayers of chlorite, kaolinite, and illite. Consequently, these metal ions exclusively interact with the surfaces of these three clay minerals, with the degree of their effects being solely dependent on the valence of the metal ions
(4) It was observed that the configuration of clay particles in the aqueous phase exerted the most significant impact on the stability of the clay-crude oil emulsion system. Among the three evaluated particle arrangements F-F, F-E, and E-E, the F-E arrangement proved to be the most stable. The particle dispersion size played a secondary role.
Data availability statement
The original contributions presented in the study are included in the article/Supplementary material, further inquiries can be directed to the corresponding authors.
Author contributions
SSZ: Writing–original draft. TL: Writing–original draft. QC: Writing–review and editing. BX: Writing–review and editing. XX: Writing–review and editing. ZY: Writing–review and editing.
Funding
The author(s) declare that no financial support was received for the research, authorship, and/or publication of this article.
Conflict of interest
The authors declare that the research was conducted in the absence of any commercial or financial relationships that could be construed as a potential conflict of interest.
The reviewer RL declared a shared affiliation with the authors to the handling editor at the time of review.
Publisher’s note
All claims expressed in this article are solely those of the authors and do not necessarily represent those of their affiliated organizations, or those of the publisher, the editors and the reviewers. Any product that may be evaluated in this article, or claim that may be made by its manufacturer, is not guaranteed or endorsed by the publisher.
References
Abbas, A. M. A.-M. M. A. A.-S. M. F., Al-Shamrani, M. A., and Abbas, M. F. (2021). Hydromechanical behavior of unsaturated expansive clay under repetitive loading. J. Rock Mech. Geotechnical Eng. 13, 1136–1146. doi:10.1016/j.jrmge.2021.05.002
Abend, S., and Lagaly, G. (2001). Bentonite and double hydroxides as emulsifying agents. Clay Miner. 36, 557–570. doi:10.1180/0009855013640009
Ana Maria Bago Rodriguez, B. P. B., and Binks, B. P. (2020). Catalysis in pickering emulsions. Soft matter 16, 10221–10243. doi:10.1039/d0sm01636e
Arroyo, CARRIQUE, Olivares, J. I. M. N. E. Z., Jiménez-Olivares, M., and Delgado, A. (2000). Rheological and electrokinetic properties of sodium montmorillonite suspensions. J. colloid interface Sci. 229, 118–122. doi:10.1006/jcis.2000.6957
Chorover, J., and Sposito, G. (1995). Surface charge characteristics of kaolinitic tropical soils. Geochimica Cosmochimica Acta 59, 875–884. doi:10.1016/00167-0379(40)03572-
Crossley, S., Faria, J., Shen, M., and Resasco, D. E. (2010). Solid nanoparticles that catalyze biofuel upgrade reactions at the water/oil interface. SCIENCE 327, 68–72. doi:10.1126/science.1180769
Fabrice, S., Jean-Marc, D., Olivier, B., Gaudin, C., Prelot, B., Zajac, J., et al. (2013). Driving force for the hydration of the swelling clays: case of montmorillonites saturated with alkaline-earth cations. J. Colloid Interface Sci. 395, 269–276. doi:10.1016/j.jcis.2012.12.050
Gui, X., Xing, Y., Rong, G., Cao, Y., and Liu, J. (2016). Interaction forces between coal and kaolinite particles measured by atomic force microscopy. Powder Technol. 301, 349–355. doi:10.1016/j.powtec.2016.06.026
He, J., Jia, H., Wang, Q. X., Xu, Y. B., Zhang, L. Y., Jia, H. D., et al. (2022). Investigation on pH and redox-trigged emulsions stabilized by ferrocenyl surfactants in combination with Al2O3 nanoparticles and their application for enhanced oil recovery. Colloids Surfaces a-Physicochemical Eng. Aspects 655, 130303. doi:10.1016/j.colsurfa.2022.130303
Hu, X. J., Long, Y. Q., Xuan, G., Wang, Y. Y., Huang, X. H., Xu, Y. P., et al. (2023). Optimized hydrophobic magnetic nanoparticles stabilized pickering emulsion for enhanced oil recovery in complex porous media of reservoir. Front. Energy Res. 11, 14. doi:10.3389/fenrg.2023.1212664
Hua, G., Wu, S., Zhang, J., Liu, R., Guan, M., Cai, Y., et al. (2022). Laminar structure and reservoir quality of shales with high clay mineral content in the qingshankou formation, songliao basin. Energies 15, 6132. doi:10.3390/en15176132
Huertas, F. J. E. A., Chou, L., and Wollast, R. (1999). Mechanism of kaolinite dissolution at room temperature and pressure Part II: kinetic study. Geochimica Cosmochimica Acta 63, 3261–3275. doi:10.1016/s0016-7037(99)00249-5
Janssen, M. T. G., Mutawa, A. S., Pilus, R. M., and Zitha, P. L. J. (2019). Foam-assisted chemical flooding for enhanced oil recovery: effects of slug salinity and drive foam strength. Energy & Fuels 33, 4951–4963. doi:10.1021/acs.energyfuels.9b00645
Jiang, G., Xuan, Y., Li, Y., and Wang, J. (2014). Inhibitive effect of potassium methylsiliconate on hydration swelling of montmorillonite. Colloid J. 76, 408–415. doi:10.1134/s1061933x14040061
Johnston, C. T., Sposito, G., and Erickson, C. (1992). Vibrational probe studies of water interactions with montmorillonite. Clays Clay Minerals 40, 722–730. doi:10.1346/ccmn.1992.0400611
Kassab, M., Gebaly, A. E., Abass, A., and Kady, E. E. (2021). Integrated petrophysical and mineral composition of Miocene reservoirs (Kareem and Belayim Formations), Belayim onshore oil field, Gulf of Suez, Egypt: a case study. Arabian J. Geosciences 14, 1654. doi:10.1007/s12517-021-07862-1
Liu, J. X., Zhu, H. J., Wang, P., and Pan, J. M. (2021). Recent studies of Pickering emulsion system in petroleum treatment: the role of particles. Petroleum Sci. 18, 1551–1563. doi:10.1016/j.petsci.2021.10.001
Liu, Z.-X., Liang, Y., Wang, Q., Guo, Y.-J., Gao, M., Wang, Z.-B., et al. (2020). Status and progress of worldwide EOR field applications. J. Petroleum Sci. Eng. 193, 107449. doi:10.1016/j.petrol.2020.107449
Liu, Z., Li, Y., Luan, H., Gao, W., Guo, Y., and Chen, Y. (2019). Pore scale and macroscopic visual displacement of oil-in-water emulsions for enhanced oil recovery. Chem. Eng. Sci. 197, 404–414. doi:10.1016/j.ces.2019.01.001
Oka, C., Ushimaru, K., Horiishi, N., Tsuge, T., and Kitamoto, Y. (2015). Core–shell composite particles composed of biodegradable polymer particles and magnetic iron oxide nanoparticles for targeted drug delivery. J. MAGNETISM MAGNETIC Mater. 381, 278–284. doi:10.1016/j.jmmm.2015.01.005
Ren, J., Deshun, Y., and Zhai, R. (2021). Rheological behavior of bentonite-water suspension at various temperatures: effect of solution salinity. Eng. Geol. 295, 106435. doi:10.1016/j.enggeo.2021.106435
Son, H. A., Yoon, K. Y., Lee, G. J., Cho, J. W., Choi, S. K., Kim, J. W., et al. (2015). The potential applications in oil recovery with silica nanoparticle and polyvinyl alcohol stabilized emulsion. J. Petroleum Sci. Eng. 126, 152–161. doi:10.1016/j.petrol.2014.11.001
Sztukowski, D. M., and Yarranton, H. W. (2005). Oilfield solids and water-in-oil emulsion stability. J. Colloid Interface Sci. 285, 821–833. doi:10.1016/j.jcis.2004.12.029
Uddin, F. (2008). Clays, nanoclays, and montmorillonite minerals. Metallurgical Mater. Trans. A 39, 2804–2814. doi:10.1007/s11661-008-9603-5
Xuan, Y., Jiang, G., Li, Y., Wang, J., and Geng, H. (2013). Inhibiting effect of dopamine adsorption and polymerization on hydrated swelling of montmorillonite. COLLOIDS SURFACES A-PHYSICOCHEMICAL Eng. ASPECTS 422, 50–60. doi:10.1016/j.colsurfa.2013.01.038
Zhang, F. F., Liang, W., Dong, Z. X., Niu, Q. Q., Yang, Z. H., Lin, M. Q., et al. (2023). Dispersion stability and interfacial properties of modified MoS2 nanosheets for enhanced oil recovery. Colloids Surfaces a-Physicochemical Eng. Aspects 675, 132013. doi:10.1016/j.colsurfa.2023.132013
Zhang, N., Zhang, L., and Sun, D. (2015). Influence of emulsification process on the properties of pickering emulsions stabilized by layered double hydroxide particles. Langmuir 31, 4619–4626. doi:10.1021/la505003w
Keywords: pickering emulsion, crude oil extraction, clay minerals, interfacial chemistry, emulsion breaking
Citation: Zhang S, Liu T, Chen Q, Xiao B, Xian X and Ye Z (2024) The influence and mechanism exploration of hydration environment on the stability of natural clay crude oil emulsion. Front. Energy Res. 12:1362462. doi: 10.3389/fenrg.2024.1362462
Received: 28 December 2023; Accepted: 06 May 2024;
Published: 11 June 2024.
Edited by:
Fei Wang, Qingdao University of Science and Technology, ChinaReviewed by:
Rui Liu, Southwest Petroleum University, ChinaYang Yang, Chengdu University of Technology, China
Shaohua Gou, Consultant, Yibing, China
Copyright © 2024 Zhang, Liu, Chen, Xiao, Xian and Ye. This is an open-access article distributed under the terms of the Creative Commons Attribution License (CC BY). The use, distribution or reproduction in other forums is permitted, provided the original author(s) and the copyright owner(s) are credited and that the original publication in this journal is cited, in accordance with accepted academic practice. No use, distribution or reproduction is permitted which does not comply with these terms.
*Correspondence: Zhongbin Ye, eWV6aG9uZ2JpbjE5NjZAMTYzLmNvbQ==