- Department of Mechanical Engineering Science, Faculty of Engineering and the Built Environment, University of Johannesburg, Johannesburg, South Africa
This study examined the influence of DES pretreatment using choline chloride and ethyl glycerol with the molar ratio of 1: 1 at different solid:liquid ratios and temperatures on groundnut shells’ microstructural arrangement and biomethane yield. Scanning electron microscopy (SEM), X-ray diffraction (XRD), and Fourier-transform infrared spectroscopy (FTIR) were used to study the effects of pretreatment on microstructural arrangements, and the pretreated substrate was digested at mesophilic temperature to determine its biomethane potential. The result of SEM analysis indicated that DES pretreatment alters the microstructural arrangement of groundnut shells, and XRD analysis showed an optimum crystallinity index of 20.71% when the substrate with a solid:liquid ratio of 1:2 was experimented at 80°C. The highest theoretical biomethane yield of 486.81 mL CH4/gVSadded was recorded when the substrate with a 1:4 solid:liquid ratio was investigated at 100°C, and the highest biodegradability rate (84.87%) was observed from the substrate treated with a 1:2 solid:liquid ratio at 100°C. The optimum biomethane yield of 365.70 mL CH4/gVSadded, representing a 226.05% increase, was observed from 1:2 of solid:liquid ratios at 100°C. Therefore, DES pretreatment using choline chloride and ethyl glycerol is a bright, low-cost pretreatment method for enhancing the biomethane yield of lignocellulose feedstocks.
1 Introduction
Sustainable, affordable, and reliable energy, as well as appropriate waste management systems, support sustainable development (Olatunji and Madyira, 2023a). Energy is crucial to social and economic development; however, the primary source of world energy is fossil fuels that burn with harmful effects on the environment (Yildiz, 2018). Energy resources have improved living and working conditions, boosted economic expansion, allowed comfort and movement, and made necessities accessible. Energy-driven technologies replaced manual, labor-intensive jobs during the Industrial Revolution, when energy supplies were abundant, and there was little worry about their usage and availability; however, this is no longer the case. The difference between the supply and demand of energy has widened significantly. This imbalance may be caused by several factors, including population growth, rising living standards, careless use, and technical breakthroughs (Gautam et al., 2019). Most energy mix projections indicate that the present and predicted future energy sources are unsustainable. Despite projecting serious improvements in energy supply, it has been observed that long projection of energy demand globally will rise drastically, with a major increase to be experienced in developing countries. These trends suggest that a decoupling of economic activity from the use of fossil fuels, the primary source of energy, is important to meet the requirements for the three elements of sustainability (economic, environmental, and social) regarding energy production and consumption (Oyedepo, 2012). Fossil fuels are not renewable and are depleted faster than the rate at which they are being replenished. Another challenge of relying majorly on fossil fuels is the release of carbon dioxide during combustion, which is valued at approximately 21.3 billion tons per annum. It was observed that carbon dioxide released from fossil fuels and industries in 2016 was nearly stable, approximately 0.2%, as against the projected value of 2.2% (2017 UN Climate Change Conference in Bonn, Germany (COP23)—Wednesday, 4 October 2017, n.d.). This is not only because of reductions in fossil fuel consumption but also due to energy efficiency techniques and the production of renewable energy.
Renewable energy will play a significant role in supplying future energy requirements and the remediation of the ecosystem. Renewable energy is the energy source replenished through natural processes with limitless supply and can operate without pollution. This includes energy from the Sun, geothermal sources, biomass, wind, ocean waves and tides, and rivers. Renewable energy technologies (RETs), commonly known as “clean technologies” or “green energy,” are technologies that have been created to utilize these forms of renewable energy. Because of their unlimited supply, their supply is secure compared to that of fossil fuels, which is determined by national and international market situations (Oyedepo, 2012). Developing and applying renewable energy systems needs special attention, particularly in awareness of the harmful effects of fossil fuel usage. Globally, there has been a growing increase in the development of sustainable energy sources in recent years. The general acceptability of renewable energy is crucial to the development of sustainable energy in developing and developed countries. When renewable resources are used wisely for the benefit of current and future generations, they should be used at a reasonable pace that is neither too fast nor too slow. This ensures that the natural wealth they symbolize is transformed into long-term wealth as they are used (Taiwo, 2009). Although there are significant disparities in the objectives and accomplishments of different countries in the energy transition, it is undeniable that they are at a pivotal point in the process. Indeed, the energy transition needs to be established while fossil fuel prices are low and are expected to remain that way for the foreseeable future to prevent the irreversible effects of climate change caused by greenhouse gas emissions through fossil fuel burning. Major advantages and drawbacks are associated with consolidating the transition from fossil fuels to clean energy, and energy policies must be designed to handle these challenges appropriately (Arezki and Matsumoto, 2017).
Solid waste generation and management continue to be major social and political issues, especially in cities where waste generation outpaces available space and there is a rapid rise in population. Several worldwide development agendas, charters, and visions emphasize solid waste management’s role in attaining sustainable development. For instance, appropriate and sustainable waste management can assist in achieving some of the United Nations’ Sustainable Development Goals (SDGs), such as goals 2, 3, 6, 7, 9, 13, and 15 (Chowdhury et al., 2022). Additionally, it supports the development of a circular urban economy that minimizes the use of finite resources, encourages the reuse and recycling of materials to reduce waste, pollution, and costs, and promotes green growth. In a more sustainable waste management approach, techniques such as reduction in production, waste classification, reuse, recycling, and energy recovery are given priority over open dumps, landfilling, and open incineration (Yang et al., 2018; Kabera and Nishimwe, 2019). Energy recovery from waste is getting better attention globally because of its inclusivity, environment-friendly nature, and minimal harmful impact on human health and the environment (Rimi Abubakar and Aina, 2016). Waste-to-energy (WTE) technology not only produces clean energy but also offers an environmentally beneficial waste disposal option. Waste is converted into energy using WTE techniques. The kind of waste, the plant’s performance, and the method or path taken for energy recovery all affect how much energy can be recovered (Okedu et al., 2022). The most promising solution to the energy dilemma appears to be converting waste into energy, which can be accomplished through various technological means. The feasibility of these WTE solutions is determined by several parameters, including the energy content, the desired final energy form, the nature and composition of waste, the chemical and thermodynamic conditions, and the overall energy efficiency. Waste-to-energy technologies include biochemical conversion, thermal conversion, landfill with gas capture, chemical conversion (esterification), microbial fuel cell, and hydrothermal carbonization (Gautam et al., 2019).
Biochemical conversion techniques use microbial processes to transform organic waste into energy. The biodegradable portion of municipal solid wastes, wastewater sludge, agricultural residues, energy grasses, etc., are some of the bright feedstocks for these techniques (Olatunji et al., 2023a). Waste-to-energy biochemical conversion is divided into two processes, which are fermentation and anaerobic digestion. Fermentation is the process of converting organic waste into alcohol or acid (ethanol, lactic acid, etc.) and residue that is rich in nutrients in the absence of oxygen (Cai et al., 2016). Bioethanol is a clean fuel that can be produced from a pure culture of specific yeast strains (Orozco-González et al., 2022). The microbial breakdown of organic waste in the absence of oxygen is known as anaerobic digestion, producing biogas (methane and carbon dioxide) digestate (fertilizer). Biogas released from tightly closed tanks (digester) can be used to generate electricity or heat as renewable energy (Olatunji et al., 2022a). Anaerobic digestion has four stages: hydrolysis, acidogenesis, acetogenesis, and methanogenesis (Raja and Wazir, 2017). Biogas can be generated from different organic wastes, such as corn cob, groundnut shells, wastewater sludge, cow dung, Jatropha cake, macroalgae and microalgae, poultry droppings, and duck waste (Ogunkunle et al., 2018; Venturin et al., 2018; Olatunji and Madyira, 2023b). Utilization of lignocellulose biomass for biogas production has been encouraged because of its environment-friendly nature and role as an economically sustainability source of feedstock. It is regarded as an eco-friendly second-generation technology for energy generation. Methane production from lignocellulose is an efficient technique for energy recovery from biomass compared to other techniques that require a higher energy input/output ratio (Monlau et al., 2013). Lignocellulose feedstocks are identified as an abundant and renewable feedstock for biogas production. The global production is approximately 120 × 109 annually, equivalent to 2.2 × 1021 J, approximately 300 times higher than the present global energy demand (Guo et al., 2015). One of the largest sources of lignocellulose materials is the crop residues from agricultural activities; most of them do not have other uses. The unused agricultural residues on the field can release greenhouse gases or cause serious environmental pollution when burned openly. These residues have been reported to have high biogas production potential that can be annexed as renewable energy sources (Rabemanolontsoa and Saka, 2016; Xu et al., 2019; Podgorbunskikh et al., 2020). However, lignocellulose feedstocks are recalcitrant, inhibiting the anaerobic digestion process and limiting their biogas production potential (Madyira and Olatunji, 2023). Therefore, pretreatment is required to convert lignocellulose feedstocks into biogas and other value-added products efficiently. Pretreatment aimed to break down the strong microstructural arrangement of the lignocellulose by altering the lignin and hemicellulose arrangement, thereby improving the porosity and reducing the cellulose crystallinity and polymerization level (Patowary and Baruah, 2018). Different pretreatment techniques have been investigated on lignocellulose, and recently, several studies have examined these techniques for improving the digestion process and enhancing biogas release from lignocellulose feedstock. These techniques are categorized as biological, thermal, mechanical/physical, chemical, nanoparticle additives, and combined pretreatment (Olatunji et al., 2021). A comprehensive review of the application of pretreatment techniques on lignocellulose feedstock before biogas and methane production has been published (Millati et al., 2011; Zhang et al., 2016; Brémond et al., 2018; Olatunji et al., 2021; 2023b). Different methods have been experimented with on various lignocellulose feedstocks, but their efficiency depends on the microstructural arrangement, treatment type, temperature, etc., making comparing the pretreatment techniques difficult.
Deep eutectic solvents (DESs) are a chemical pretreatment technique that has been regarded as one of the most acceptable green solvents of the 21st century. DESs are gaining attention in studies due to their biodegradability, low cost, ease of recycling, high solubility, non-flammability, and environment-friendly nature (Smith et al., 2014). DESs are defined as the combination of two or more components that can be liquid or solid, and the specific resulting mixture has a high melting point but becomes liquid at room temperature. Common DESs are choline chloride, succinic acid, citric acid, glycerol, urea, etc. (Paiva et al., 2014). Compared to other chemical and ionic liquids, the application of DESs has been limited to enzyme reactions (Gorke et al., 2008), organic reactions (Gore et al., 2011), electrochemistry (Jhong et al., 2009), and organic extractions (Abbott et al., 2009). Because of their biodegradable characteristics and acceptable toxicity, they have been considered an ideal candidate for feedstock pretreatment (Dai et al., 2013). Pretreatment with DESs improves the surface area and cellulose crystallinity, enhancing digestion. It also eliminates hemicellulose by altering the glycosidic bonds (C–O–C) to produce monosaccharides (Xu et al., 2020). Furthermore, DESs can selectively destroy the higher percentage of lignin by primarily splitting the aryl ether bond (β-O-4) within the feedstock (Alvarez-Vasco et al., 2016; Shen et al., 2020). The efficiency of DES pretreatment depends on the strength of the selected DESs to separate carbon–carbon linkages and aryl ether linkages in the feedstock’s microstructural arrangement (Xu et al., 2020).
Groundnut shells are one of the most abundant lignocellulose materials with biogas production potential (Olatunji et al., 2022c). Being a lignocellulose feedstock, the anaerobic digestion of this feedstock is ineffective without pretreatment. Some studies have examined the influence of different pretreatment techniques to enhance the biogas yield of groundnut shells (Dahunsi et al., 2017; Jekayinfa et al., 2020; Olatunji and Madyira, 2023b). Their findings indicate that pretreatment methods improve the biogas and methane yield of groundnut shells, but their degree of effectiveness differs. It was further reported that there is still a need for more research in the pretreatment of this economical feedstock to establish the most economical means of optimum recovery of renewable energy from groundnut shells. Therefore, this study examines the effects of DES pretreatment on the microstructural arrangement, crystallinity, functional groups, and biomethane yield of groundnut shells. Groundnut shells are mostly left on the farm/processing area after harvesting and are usually burnt off, posing health and fire-related hazards, promoting pathogen growth, and contributing to greenhouse gas emissions. The literature on the DES pretreatment technique is limited, and we aim to establish the potential of DESs on the structural arrangement and biomethane yield of groundnut shells. The study used scanning electron microscopy (SEM), X-ray diffraction (XRD), and Fourier-transform infrared spectroscopy (FTIR) to investigate the effects of DES pretreatment on surface changes and interior arrangement to provide the theoretical interpretation of the method. Finally, the pretreated and untreated feedstocks were subjected to an anaerobic digestion process to ascertain the influence of the method on biomethane yield. The result from this study is expected to provide baseline information for future studies on lignocellulose pretreatment for enhancing biomethane yield.
2 Materials and methods
2.1 Materials
Groundnut shells were sourced locally from a groundnut farm at Mogwase, Rustenburg, North West Province, South Africa (GPS: −25.2621, 27.27336), after harvesting and processing. The sample was then reduced to a particle size of between 2 and 4 mm using a hammer mill. Inoculum was collected from a recently terminated digester, whereby wastewater was digested at mesophilic temperature (35 ± 2). The pH value of the inoculum was observed to be 7.2. The substrate and inoculum were stored at 4°C in the laboratory before pretreatment, laboratory analysis, and anaerobic digestion. Choline chloride and ethyl glycerol were procured from Sigma-Aldrich (Darmstadt, Germany) for pretreatment.
2.2 Deep eutectic solvent preparation
The deep eutectic solvent was prepared from the combination of choline chloride and ethyl glycerol, as reported by Smith et al. (2014), with a pH value of 6.7 (presented in Table 1). The choline chloride and ethyl glycerol ratios were measured using a 1: 1 M ratio. A mixture of 1: 1 was selected because they are both hydrogen-bond donor and acceptors, which is the basis for the complexation of solid-producing liquids with a supramolecular arrangement (Dai et al., 2013). The mixture of choline chloride and ethyl glycerol in the beaker was heated at different temperatures, as shown in Table 1, on a magnetic stirrer at 500 rpm for 1 hour until a clear homogenous solution was achieved (Olugbemide et al., 2021).
2.3 Substrate pretreatment with DES
Groundnut shells were pretreated with the prepared DES using different solid:liquid ratios and temperatures. The calculated quantity of groundnut shells was added to the DES using the solid:liquid ratio presented in Table 1 and heated at temperatures specified in Table 1 for 1 hour as recommended (Olugbemide et al., 2021). Pretreated samples were allowed to cool down to room temperature and washed with running water until a neutral pH (7) was achieved. The cleaned feedstock was oven-dried at 70°C for 1 h and kept in a zip-lock plastic before being stored at 4°C in the laboratory for physicochemical property analysis, structural analysis, and the biomethane potential test.
2.4 Substrate characterization
2.4.1 Physicochemical property analysis
To determine the compositional level of the substrate and inoculum, their physicochemical properties were examined. Total solids (TSs), moisture content, percentage ash, and volatile solids (VSs) were examined using the established procedures of the Association of Official Analytical Chemists (AOAC) (Official Methods of Analysis, 21st Edition (2019)—AOAC INTERNATIONAL, n.d.). Lignin, hemicellulose, and cellulose percentage were analyzed as prescribed by Van Soest et al. (1991). The nitrogen, carbon, and hydrogen percentages were determined using an elemental analyzer (Vario El cube, Germany), and the percentage of oxygen was calculated with the assumption that C + N + H + O = 99.50% (Rincón et al., 2012).
2.4.2 Microstructural characterization
The impacts of different solid:liquid ratios and temperatures of DES pretreatment on the microstructural arrangement of groundnut shells were studied using a scanning electron microscope (VEGA3 TESCAN X-Max, Czech Republic). The analysis was duplicated, and the images were picked at different magnifications before selecting the most explicit image. X-ray diffraction (D-8 Advance, Indiana, USA) was used to study the degree of cellulose crystallinity of groundnut shells before and after DES pretreatment. This investigation was carried out at 5°C–35°C with 5°C/min speed at 2Ө as the diffraction angle. Equation 1 was used to calculate the crystallinity index (Ic) of the pretreated and untreated substrates using the empirical data from the XRD analysis (Atalla and VanderHart, 1999). The influence of DES pretreatment on the functional group of the substrate was investigated under Fourier-transform infrared spectroscopy (SHIMADZU IRAfinity-1, Japan). This was considered under the wavelengths of 500 and 4,000 cm−1, and the changes in the absorbent ratio of the substrates were determined from the FTIR data using Eq. 2 (Dahunsi et al., 2019).
Here, Ic is the crystallinity index, Imax is the highest diffraction at peak position at 2Ө = 22°, and Ix is the intensity at 2Ө = 18°.
2.5 Anaerobic digestion
The experiment to examine the influence of the DES pretreatment method on groundnut shells was conducted in a batch digester in the laboratory following the VDI 4630 standard (organischer Stoffe Substratcharakterisierung, 2016). The experiment was set up at mesophilic temperature using the Automatic Methane Potential Test System II (AMPTS II). AMPTS II reactor bottles of 10–500 mL were preloaded with 400 g of stabled inoculum as recommended by VDI 4630 (organischer Substratcharakterisierung, 2016). The quantity of substrate added to the inoculum was determined using Eq. 2, considering the volatile solids of the substrate and inoculum. Reactors were loaded at a 2:1 ratio of substrate:inoculum, and the AMPTS II water bath was maintained at 37°C ± 2 throughout the digestion process. The calculated quantity of DES-pretreated and untreated substrates was charged in each digester, as given in Table 1, and the experiment was replicated twice as recommended (Caillet et al., 2019). Two reactors with only stabilized inoculum were run as a parallel experiment to ascertain the exact volume of biomethane released. The biomethane yield released from the parallel experiment was used as a head-space correction for the reactors with both substrate and inoculum. In the AMPTS II programming, the mixing time was set as 60 s with 60 s off time. The agitation speed adjustment was maintained at 80%, and the carbon dioxide flush gas had a concentration of 10%. The reactor head-space was kept at 100 mL, and 60% methane content was presumed (Brennan and Owende, 2010). Nitrogen baseline gas was used to flush out the oxygen in the reactors to set an anaerobic condition. Screw cap bottles of 100 mL were filled with NaOH (3M NaOH) at the carbon dioxide removal section to eliminate the carbon dioxide in the gas released. The reactor bottles were linked with the carbon dioxide removal bottles using silicon tubes. The carbon dioxide removal unit was also connected to the third unit with another silicon tube, where the quantity of biomethane generated was rerecorded. Gas chromatography installed with the system was used to analyze the gas released to understand the quality of the gas. When it was observed that the daily biomethane released was below 1% of the cumulative biomethane released by day 35, the experiment was terminated.
Here, Ms is the mass of the substrate (g), Mi is the mass of the inoculum (g), Cs is the concentration of the substrate (%), and Ci is the concentration of the inoculum (%) (organischer Substratcharakterisierung, 2016).
2.6 Theoretical methane yield and biodegradable rate (Bd)
The biomethane potential of the pretreated and untreated substrates was determined through theoretical yield using the result of the elemental analysis with Eqs 3, 4 (Buswell and Mueller, 2002). The biodegradability percentage of the digestion process was calculated using the experimental methane yield (EMY) and theoretical methane yield (TMY), as shown in Eq. 5 and reported by Elbeshbishy et al. (2012).
where a, x, y, and z are the stoichiometry ratios of carbon, hydrogen, oxygen, and nitrogen, respectively.
3 Results and discussion
3.1 Effects of DES pretreatment on the structural arrangements of groundnut shell
3.1.1 Effects of pretreatment on microstructural arrangements
The influence of different DES pretreatment conditions on the microstructural arrangement of groundnut shells was investigated with scanning electron microscopy, and the result is presented with the images in Figure 1. It was observed from the figure that DES pretreatment significantly impacts the microstructural arrangement of the substrate when images in Figures 1A–D are compared to Figure 1E. The images showed that different pretreatment conditions produce varying effects on the substrate. The effects of DES pretreatment on the groundnut shells can be traced to the biocatalysis characteristics of the solvents (Paiva et al., 2014). Figure 1E presents a more intertwined and smoother arrangement with several fiber layers that can inhibit the activities of microorganisms during digestion. Figures 1A–E indicate that the DES alters the lignin arrangement of the substrate with variation in the influence based on solid:liquid ratios and treatment temperatures. It was observed that the cell walls were broken down, and the initial compact and fine surface were loosened and fragmented, as can be observed in the morphological images shown in Figures 1A–E. It was observed from the images that the varying conditions (solid:liquid ratios and temperature) influenced the defibrillation and coarseness of the substrate. This result agrees with the previous report that indicated that pretreatment techniques significantly influence the structural arrangement of lignocellulose feedstocks. Still, the severity level depends on the microstructural arrangement of the feedstock and pretreatment conditions (Olatunji et al., 2021).
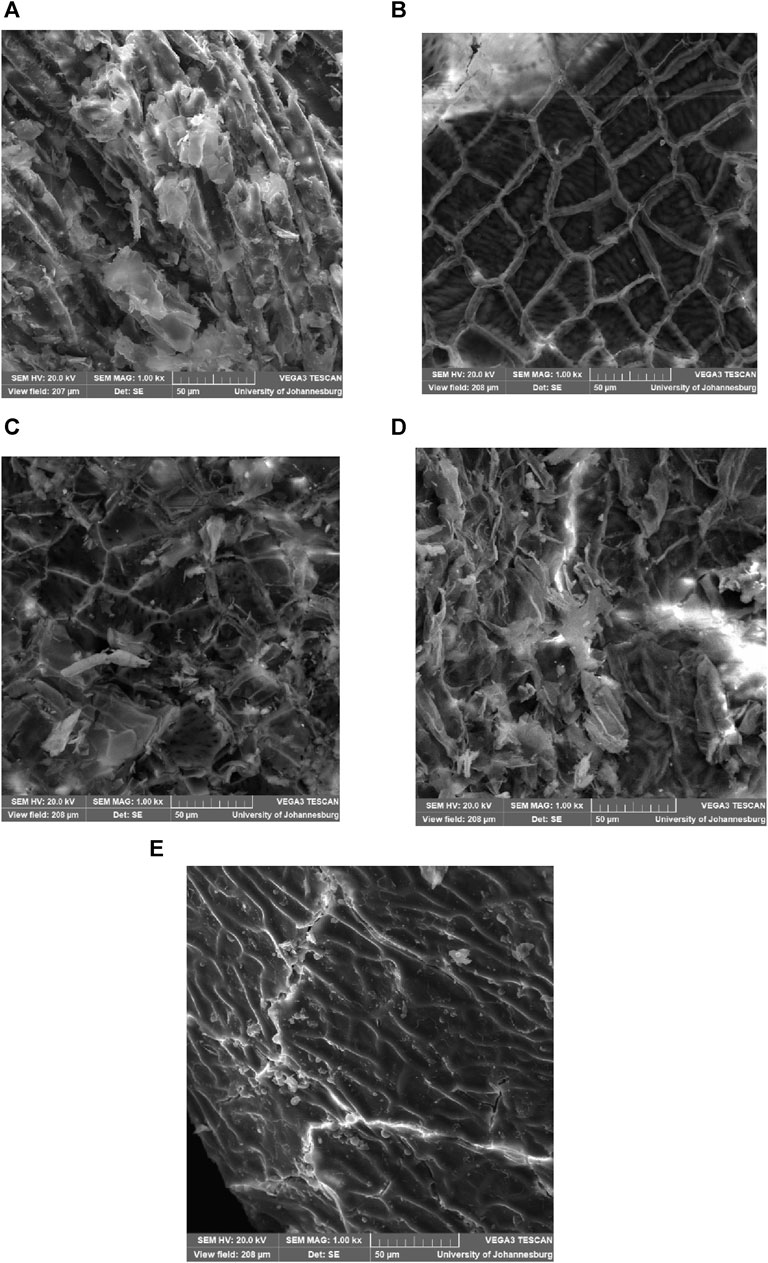
FIGURE 1. SEM images of DES-pretreated (A) 1:2 at 80°C, (B) 1:4 at 80°C, (C) 1:2 at 100°C, 1:4 at 100°C, and untreated groundnut shells.
The image in Figure 1A presents more damage to the lignin portion of the feedstock, which is swollen and has higher degrees of surface fragmentation with internal tissue exposure. It shows some tendency for methanogenic bacteria activities to have more accessible space during anaerobic digestion. On the other hand, the image in Figure 1B shows a collapsed structure with pores and cracks. It was observed that the internal tissue exposure was limited compared to other pretreated substrates, which can minimize the accessibility of anaerobic digestion bacteria during digestion. Comparing images picked for treatments A and B, it is evident that the selected solid:liquid ratio significantly influences the impacts of DES pretreatment on the microstructural arrangements of groundnut shells, which agrees with the previous study (Olugbemide et al., 2021). Figures 1C, D depict a significant effect of DES pretreatment on groundnut shells. There is a sign of lignin removal/redistribution, which enhances the accessibility to cellulose and hemicellulose. However, this is more pronounced in Figure 1D than in Figure 1C, which indicates that the solid:liquid ratio is important to the effect of DES pretreatment. These images indicate better availability for methanogenic bacteria, which is expected to improve the biomethane yield, provided the level of inhibitory compounds generated is minimal. Compared to other chemical pretreatment of groundnut shells, DES pretreatment’s influence on the microstructural arrangements of groundnut shells is not as severe as that of alkali and acidic pretreatment (Madyira and Olatunji, 2023). This could be due to the strength of the other chemicals, although it is presumed that DES will produce fewer inhibitory compounds, which could be advantageous to biomethane release.
3.1.2 Effects of DES pretreatment on crystallinity
Lignocellulose feedstocks have been observed to consist of both crystalline and non-crystalline microstructures, whereby the cellulose portion is identified as a crystalline structure, while hemicellulose and lignin are reported to be amorphous structures (Kim and Holtzapple, 2006; Sarbishei et al., 2021). DES-pretreated and untreated groundnut shells were investigated by XRD; the result is illustrated in Figure 2. It was inferred that untreated samples produce seven different distinctive peaks, which is a characteristic of cellulose materials (Olugbemide et al., 2021). It was observed that the peak of the pretreated substrate begins to flatten out at 18°, indicating crystalline reduction and cellulose transformation. There are no significant differences in the intensity of the pretreated samples, meaning that the cellulose did not undergo structural change during pretreatment. No noticeable difference exists between the peaks of the pretreated feedstocks, indicating cellulose transition during dissolution (Moyer et al., 2018).
Table 2 presents the crystallinity index (Ic) of pretreated and untreated substrates. It was observed that the Ic of the samples is 20.71, 19.18, 20.44, 19.17, and 16.52 for treatments A, B, C, D, and E, respectively. It was observed from the table that DES pretreatment significantly influences the Ic of groundnut shells. Compared to the untreated substrate, the Ic was improved by 25.36, 16.10, 23.55, and 16.04% for treatments A, B, C, and D, respectively. It was observed that the solid:liquid ratio plays a more significant role in the Ic than the temperature. A solid:liquid ratio of 1:2 showed better Ic improvement than 1:4, but temperatures did not have any significant impact. This result agrees with other literature works that pretreatment methods influence the Ic of lignocellulose feedstocks. Compared to other studies, this research produced a lower Ic than other pretreatment techniques on the same substrate (Madyira and Olatunji, 2023). The lower Ic recorded in this study can be traced to the reduction in cellulose content, which implies that the DES molar ratio is considered, the solid:liquid ratio is reduced, and the temperature reduces the Ic of groundnut shells. Furthermore, the lower Ic in this study means an increase in the diffraction intensity observed in the amorphous region, which can be linked to the properties of the DES used to recrystallize the cellulose crystal region (Olugbemide et al., 2021). It was also observed that the DES composition partially reduces the amorphous portion, like hemicellulose and lignin. It has been reported that crystalline cellulose restricts microbial activities more than amorphous cellulose (Zhao et al., 2012). Findings from this study support previous investigations on the influence of DES pretreatment techniques on lignocellulose feedstocks (Procentese and Rehmann, 2018). It is difficult to compare the effects of the pretreatment techniques because the impact of pretreatment depends on the structural arrangement of the feedstocks, which varies from one to another (Olatunji et al., 2021). Different studies have investigated the influence of crystallinity on enzymatic hydrolysis, but there is no concrete agreement because other factors involved in the process are more important or the same. When dilute acidic pretreatment of lignocellulose materials was considered, it was observed that the Ic did not directly relate to enzymatic hydrolysis (Moutta et al., 2014).
On the other hand, a significant relationship between the Ic and enzymatic hydrolysis at high enzyme loading was observed to be negative. The investigation also opined that the influence of the Ic on enzymatic hydrolysis needs to be examined without enzymes being a hindrance parameter (Li et al., 2014). The result of Ic values from this investigation showed an improved crystallinity compared to untreated substrate, and it is expected to enhance biomethane yield provided other factors (inhibitory compounds, pH, temperature, etc.) are within the acceptable range.
3.1.3 Functional group analysis
The impact of DES pretreatment on the functional group characteristics of groundnut shells and untreated feedstock was examined under FTIR spectra. Figure 3 illustrates the detailed characterization and spectra images, while Table 3 presents the spectrum assignment as observed by Shahid et al. (2020). The samples followed the same pattern until 3,329 cm−1 wavenumber was reached, as shown in Figure 3. The O–H straining in the untreated groundnut shells is responsible for the vibration intensity detected at 3,329 cm−1, which decreased with DES treatment. This suggests that both intramolecular and extramolecular hydrogen bonds in the cellulose were broken down, which facilitates better cellulose digestion because the dissolution of hydrogen bonds can modify how groundnut hells are arranged and increase the amount of cellulose available for anaerobic digestion. Similar straining vibrations for –CH and –CH2 were revealed by the vibration detected at 2,921 cm−1. This indicates a partial breakdown of the carbon chain following DES pretreatment, which results in the alteration in the cellulose structure. Carbonyl bands are apparent at approximately 2,624 cm−1 and are associated with the hydroxyl C=O straining vibration. These are the ester functional groups connected to ketones, carboxylic compounds, celluloses, and hemicelluloses (Heredia-Guerrero et al., 2014). This feature is readily discernible in the untreated substrate, and it was observed that it gradually vanished in all the treated specimens. The extinction of the carbonyl might be connected to the removal of a significant amount of lignin. The C=C aromatic structural vibration in lignin and the C=C straining of the aromatic ring are responsible for the strength of vibrations at 1,992 cm−1 and 1,792 cm−1, respectively. The strength of the substrate samples was lowered at different points following the DES pretreatment. As the solid:liquid ratio and temperature increased, the vibration strength at 1,509 cm−1 indicated that the C–O–C stretching vibration was less prominent in the untreated substrate, confirming lignin removal.
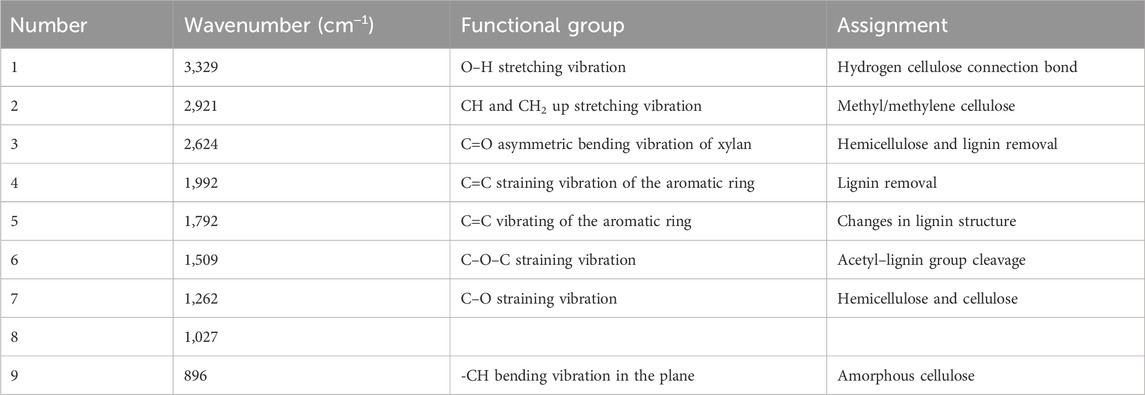
TABLE 3. FTIR spectrum peak assignments of groundnut shells pretreated with DES at varying conditions.
However, the C–O stretching vibration in cellulose and hemicellulose is represented by the vibration strength at 1,262 cm−1. The strength was moderately reduced, suggesting that the DES pretreatment fractured the hemicellulose and cellulose parts. There was no discernible difference in treatments A and C and the peak at 896 cm−1 except for treatments B and D. This section displays the C–H bending vibration in the cellulose’s plane, and the 1:2 solid:liquid ratio has the most impact on it.
3.2 Effect of DES on the physicochemical properties of groundnut shells
The AOAC standard procedure was used to determine the TS and VS of the DES-pretreated and untreated groundnut shells (Official Methods of Analysis, 21st Edition (2019)—AOAC INTERNATIONAL, 2019). It was noticed that the TS value was 85.00, 78.63, 100, 98.64, and 100% for treatments A, B, C, D, and E, respectively. Likewise, the VS values of 97.00, 97.00, 98.50, 99.00, and 94.00 were observed for treatments A, B, C, D, and E, respectively. It was noticed that DES pretreatment influenced the TS and VS of groundnut shells. The VS values of pretreated groundnut shells were improved compared to those of the untreated substrate. This can be linked to the strength of the pretreatment technique to remove/redistribute the lignin portion and expose the cellulose, the major content for biomethane production. This indicates that the pretreated substrate’s biomethane potential is high since the VS represents the percentage of substrate available for biogas production. This result corroborates previous studies on the influence of pretreatment on the VS of lignocellulose materials (Olatunji and Madyira, 2023c). It was noticed that the solid:liquid ratio does not significantly impact the VS of the substrate, but temperature variation does. EDS pretreatment was discovered to influence the elemental composition of groundnut shells. The analysis shows that DES pretreatment increases the percentage of the carbon content but reduces the nitrogen content. The increase in carbon content indicates food availability for methanogenic activities during biomethane production and determines the quantity and quality of biomethane released (Khayum et al., 2020). This may be because some nitrogen content was used or released during pretreatment. This has a significant influence on the C/N ratio of the substrate. It was noticed that the C/N ratios were 48.37, 47.29, 52.95, 66.93, and 27.63 for treatments A, B, C, D, and E, respectively. It has been observed that a C/N ratio of 20–30 is the most productive for biomethane production (Kainthola et al., 2020). This implies that only the untreated substrate falls within the range. An inoculum high in nitrogen content will be ideal for effectively digesting the pretreated substrate. DES pretreatment affected the values of hydrogen and oxygen content, as shown in Table 4. This result agreed with previous studies on the impact of DES pretreatment on the physicochemical characteristics of lignocellulose feedstocks (Dai et al., 2013; Smith et al., 2014; Procentese et al., 2015).
3.3 Effect of DES pretreatment on biomethane yield
3.3.1 Daily biomethane yield
The daily biomethane yield generated from the DES-pretreated and untreated groundnut shells is illustrated in Figure 4. It was observed that biomethane release began from all digesters on day 2, although at a different rate. The result indicates that biomethane yield from DES-pretreated samples declines after day 2 before it peaks up later during digestion. The daily biomethane yield was observed to be improved by DES pretreatment when compared to the untreated substrate. The optimum daily biomethane yield of 92.60 mL CH4/gVSadded was recorded on day 11, while the least biomethane yield from the DES-pretreated substrate was observed from treatment A on day 6. The optimum daily biomethane released was recorded between days 6 and 11 for pretreated substrates, whereas the optimum daily biomethane yield from untreated substrates was recorded on day 16. This result further established the previous findings that pretreatment methods influence the daily biomethane yield of lignocellulose feedstocks (Olatunji and Madyira, 2023d). This indicates that DES pretreatment significantly affects the daily biomethane yield and reduces the retention time of the process. Optimum daily biomethane concentrations of 36.40, 92.60, 66.00, 80.90, and 7.12 mL CH4/gVSadded were reported for treatments A, B, C, D, and E at days 6, 11, 6, 11, and 16, respectively. It was noticed that the solid:liquid ratio plays a major role in the daily yield more than the temperature. A solid:liquid ratio of 1:4 produced the highest daily biomethane yield at the temperature ranges considered. This can be linked with the strength of the DES to remove/redistribute the lignin portion of the substrate, thereby making the cellulose accessible for methanogenic bacteria. Treatment B was observed to produce a spike on day 26 after flattening for some days, while all other treatments continued with their flattening curve. It can be inferred from this study that DES pretreatment under all the conditions experimented to improve the daily biomethane yield of groundnut shells. This investigation agreed with previous studies that concluded that pretreatment at appropriate levels could enhance the daily biomethane yield of lignocellulose feedstocks (Almomani et al., 2019; Olatunji et al., 2021).
3.3.2 Cumulative biomethane yield
DES-pretreated and untreated groundnut shells were subjected to anaerobic digestion for 35 days in a laboratory batch reactor at mesophilic conditions, and the biomethane yield is presented in Figure 5. The total biomethane yield of 272.50, 251.00, 365.70, 227.70, and 112.16 mL CH4/gVSadded was recorded for treatments A, B, C, D, and E, respectively. Compared to the untreated substrate, it was observed that the biomethane yield was improved by 142.96, 123.79, 226.05, and 103.01% for treatments A, B, C, and D, respectively. It was deduced from the result that all the pretreatment conditions of DES considered in this study enhance the biomethane yield but at different percentages. This agreed with previous studies that observed pretreatment techniques improve the biomethane yield of lignocellulose feedstocks (Olugbemide et al., 2021; Dasgupta et al., 2022; Olatunji et al., 2023b). It was observed from Figure 5 that there was a slow biomethane enhancement from all the pretreated samples at the beginning of the digestion. Still, a significant increase was experienced within the first 11 days of the retention period before it flattened around the middle of the retention period. It was inferred that the DES pretreatment method reduces the retention period of the digestion process. The influence of the solid:liquid ratio is more pronounced than that of the temperature. The result indicates that a 1:2 ratio of solid:liquid produces better biomethane yield at the different temperatures. The optimum cumulative biomethane yield of 365.70 mL CH4/gVSadded was observed from treatment C (1:2 of solid:liquid at 100°C). The least liquid ratio produced the best result because of fewer inhibitory compounds due to less liquid available for pretreatment. It was inferred from the effect that a higher solid:liquid ratio with a high temperature generates the least yield besides the untreated feedstock. This could be linked to the ability of the chemical and temperature to release inhibitory compounds during the pretreatment process. Another reason for the lower yield could be cellulose loss during pretreatment. When the biomethane yields are compared to the effect of pretreatment on the microstructural arrangement using SEM, it was noticed that the most affected samples release the highest biomethane yield. This indicates a significant correlation between microstructural arrangements and methane yield. This supports the previous studies that considered the influence of pretreatment methods on microstructural arrangements and biomethane yield (Olatunji et al., 2022a). The variation in the percentage of biomethane yield corresponding to the crystallinity index results in a slight difference. It can be established from this study that crystallinity plays a significant role in biomethane yield.
Compared with other chemical pretreatment methods of groundnut shells, it was observed that DES pretreatment produces a better yield. The methane yield of groundnut shells was enhanced by 69.79% when 3% NaOH was used for 15 min at 90°C (Olatunji et al., 2022c). Thermal pretreatment with conventional heating produces a 23.96% increase in biomethane yield, and it was observed that the low improvement could be traced to the release of inhibitory compounds during pretreatment (Olatunji et al., 2022a). A 178% increase in methane yield released was reported when acidic pretreatment of groundnut shells was considered (Olatunji and Madyira, 2023b). It can be observed that among some of the pretreatment techniques experimented with on groundnut shells, DES pretreatment produced the most significant improvement except when combined pretreatment (particle size reduction and Fe3O4 additive) was investigated (Olatunji et al., 2022b). However, it has been reported that combined pretreatment increases the cost of production compared to single pretreatment, which could present DES pretreatment as the most efficient method. When DES pretreatment was investigated on corn stover, it was noticed that biomethane yield was improved by 48% (Olugbemide et al., 2021), which is lower than the improvement achieved in this study. Although there are few studies available on DES pretreatment for biogas production, there are few that focus on other end products. Corn cob was pretreated with DES before saccharification, and an approximately 76% increase in yield was observed (Procentese et al., 2015). Enzymatic hydrolysis of oil palm trunk pretreated with DES for 24 h at 50°C produces an optimum glucose yield of 74% (Zulkefli et al., 2017). Compared with other chemical pretreatment techniques on other lignocellulose materials, it can be observed that DES pretreatment on groundnut shells is more efficient in yield. Sweet sorghum was pretreated with different techniques, and it was noticed that pretreatment with NaOH produced the highest gas, followed by oxidative pretreatment. The result showed a 90.9% rise in hydrolysis with a 19.1% increase in total sugar (Cao et al., 2012). It has been discovered that the oxidative pretreatment of rice straw increases the biogas yield; when the biogas yield and process economy are considered, the efficiency is 3% (Song et al., 2012). Anaerobic digestion of sunflower stalks produced 33% more biogas when applying oxidative pretreatment (Monlau et al., 2012). When the results of this study are compared to those of the previous investigation, it becomes evident that the lignocellulose feedstocks’ efficiency varies. Differences in the DES composition, temperature, time, and the microstructural arrangement of groundnut shells may cause this fluctuation. It can be shown from the findings that none of the lignocellulose feedstocks reviewed generates more efficient outcomes than the one found in this study when treated chemically. It is also more successful than some of the results in the literature. Since the lignocellulosic arrangement and pretreatment circumstances differ, it is difficult to conclude that this chemical pretreatment is superior to other findings.
3.3.3 Biodegradability rate
Equations 4, 5 were used to calculate the theoretical methane yield and biodegradability rate of the pretreated and untreated groundnut shells, and the result is presented in Table 5. The result indicates that DES pretreatment influenced the elemental composition of the substrate, theoretical methane yield, experimental methane yield, and biodegradability rate. The result indicates that DES pretreatment improves the biomethane potential of groundnut shells. Despite DES pretreatment, it was observed that only 67.76, 57.26, 84.87, and 46.77% of the theoretical methane yield was released while only 28.28% of the theoretical methane yield of the untreated substrate was released. It was noticed that a lower solid:liquid ratio (1:2) with a lower temperature (80°C) has a higher biodegradability rate (84.87%). At either temperature considered, it was discovered that a lower temperature with a lower solid:liquid ratio produced the best biodegradability rate. The lower biodegradability of a higher solid:liquid ratio and temperature could be linked to the release of inhibitory compounds produced during pretreatment from both chemical and heat used. This agreed with earlier reports on the ability of thermal and chemical pretreatment to generate inhibitory compounds at higher temperatures and chemical concentrations (Zhang et al., 2011; Bolado-Rodríguez et al., 2016; Dumlu et al., 2017; Şenol, 2021). Considering the percentage of degradation, the addition of another pretreatment method could improve the yield further.
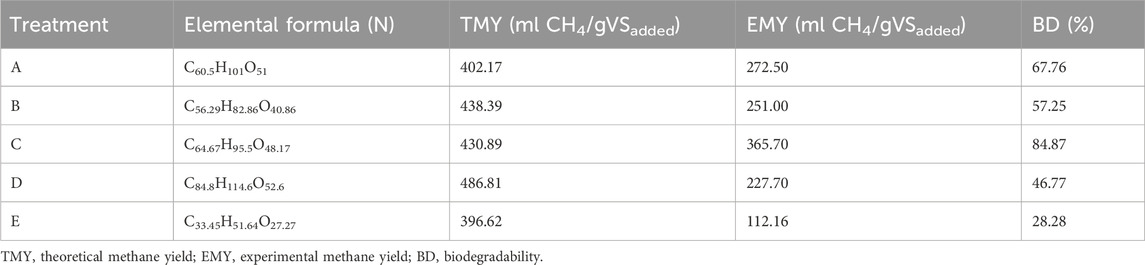
TABLE 5. elemental formula, theoretical and experimental biomethane potential, and biodegradability of groundnut shells.
4 Conclusion
The deep eutectic solvent pretreatment of groundnut shells using choline chloride and ethyl glycerol at different solid:liquid ratios and temperatures was studied. The pretreatment method was observed to be efficient for lignocellulose material in terms of microstructural arrangement and biomethane yield. The microstructural analysis of the pretreated substrate indicates that DES pretreatment alters the substrate’s structural arrangement, improving the enzymatic hydrolysis, biodegradability, and biomethane yield of groundnut shells. Biomethane yield was increased from 112.16 to 365.70 mL CH4/gVSadded, and it was discovered that a solid:liquid ratio of 1:2 at 100°C produced the optimum biomethane yield. Results from this investigation are comparable with existing studies where lignocellulose pretreatment improves the enzymatic hydrolysis and methane yield. This study produced a further step toward establishing baselines for optimum pretreatment conditions for the DES pretreatment of lignocellulose feedstocks.
Data availability statement
The raw data supporting the conclusion of this article will be made available by the authors, without undue reservation.
Author contributions
KO: conceptualization, investigation, methodology, and writing–original draft. DM: conceptualization, investigation, project administration, supervision, and writing–review and editing.
Funding
The authors declare that no financial support was received for the research, authorship, and/or publication of this article.
Conflict of interest
The authors declare that the research was conducted in the absence of any commercial or financial relationships that could be construed as a potential conflict of interest.
Publisher’s note
All claims expressed in this article are solely those of the authors and do not necessarily represent those of their affiliated organizations, or those of the publisher, the editors, and the reviewers. Any product that may be evaluated in this article, or claim that may be made by its manufacturer, is not guaranteed or endorsed by the publisher.
References
Abbott, A. P., Collins, J., Dalrymple, I., Harris, R. C., Mistry, R., Qiu, A. F., et al. (2009). Processing of electric arc furnace dust using deep eutectic solvents. Aust. J. Chem. 62, 341–347. doi:10.1071/CH08476
Almomani, F., Bhosale, R. R., Khraisheh, M. A. M., and Shawaqfah, M. (2019). Enhancement of biogas production from agricultural wastes via pre-treatment with advanced oxidation processes. Fuel 253, 964–974. doi:10.1016/J.FUEL.2019.05.057
Alvarez-Vasco, C., Ma, R., Quintero, M., Guo, M., Geleynse, S., Ramasamy, K. K., et al. (2016). Unique low-molecular-weight lignin with high purity extracted from wood by deep eutectic solvents (DES): a source of lignin for valorization. Green Chem. 18, 5133–5141. doi:10.1039/C6GC01007E
AOAC INTERNATIONAL (2019). Official methods of analysis. 21st Edition. Available at: https://www.aoac.org/official-methods-of-analysis-21st-edition-2019/ (Accessed October 15, 2021).
Arezki, R., and Matsumoto, A. (2017). Chapter 4. The energy transition in an era of low fossil fuel prices. Int. Monet. Fund. Available at: https://www.elibrary.imf.org/display/book/9781484310328/ch004.xml (Accessed November 3, 2023).
Atalla, R. H., and VanderHart, D. L. (1999). The role of solid state NMR spectroscopy in studies of the nature of native celluloses. Solid State Nucl. Magn. Reson 15, 1–19. doi:10.1016/S0926-2040(99)00042-9
Bolado-Rodríguez, S., Toquero, C., Martín-Juárez, J., Travaini, R., and García-Encina, P. A. (2016). Effect of thermal, acid, alkaline and alkaline-peroxide pretreatments on the biochemical methane potential and kinetics of the anaerobic digestion of wheat straw and sugarcane bagasse. Bioresour. Technol. 201, 182–190. doi:10.1016/j.biortech.2015.11.047
Brémond, U., de Buyer, R., Steyer, J. P., Bernet, N., and Carrere, H. (2018). Biological pretreatments of biomass for improving biogas production: an overview from lab scale to full-scale. Renew. Sustain. Energy Rev. 90, 583–604. doi:10.1016/j.rser.2018.03.103
Brennan, L., and Owende, P. (2010). Biofuels from microalgae-A review of technologies for production, processing, and extractions of biofuels and co-products. Renew. Sustain. Energy Rev. 14, 557–577. doi:10.1016/j.rser.2009.10.009
Buswell, A. M., and Mueller, H. F. (2002). Mechanism of methane fermentation. Ind. Eng. Chem. 44, 550–552. doi:10.1021/IE50507A033
Cai, D., Li, P., Chen, C., Wang, Y., Hu, S., Cui, C., et al. (2016). Effect of chemical pretreatments on corn stalk bagasse as immobilizing carrier of Clostridium acetobutylicum in the performance of a fermentation-pervaporation coupled system. Bioresour. Technol. 220, 68–75. doi:10.1016/J.BIORTECH.2016.08.049
Caillet, H., Lebon, E., Akinlabi, E., Madyira, D., and Adelard, L. (2019). Influence of inoculum to substrate ratio on methane production in Biochemical Methane Potential (BMP) tests of sugarcane distillery waste water. Procedia Manuf. 35, 259–264. doi:10.1016/J.PROMFG.2019.05.037
Cao, W., Sun, C., Liu, R., Yin, R., and Wu, X. (2012). Comparison of the effects of five pretreatment methods on enhancing the enzymatic digestibility and ethanol production from sweet sorghum bagasse. Bioresour. Technol. 111, 215–221. doi:10.1016/j.biortech.2012.02.034
Chowdhury, H., Chowdhury, T., Sharifi, A., Corkish, R., and Sait, S. M. (2022). Role of biogas in achieving sustainable development goals in Rohingya refugee camps in Bangladesh. Sustain. Switz. 14, 11842. doi:10.3390/su141911842
Dahunsi, S. O., Adesulu-Dahunsi, A. T., Osueke, C. O., Lawal, A. I., Olayanju, T. M. A., Ojediran, J. O., et al. (2019). Biogas generation from Sorghum bicolor stalk: effect of pretreatment methods and economic feasibility. Energy Rep. 5, 584–593. doi:10.1016/j.egyr.2019.04.002
Dahunsi, S. O., Oranusi, S., and Efeovbokhan, V. E. (2017). Optimization of pretreatment, process performance, mass and energy balance in the anaerobic digestion of Arachis hypogaea (Peanut) hull. Energy Convers. Manag. 139, 260–275. doi:10.1016/J.ENCONMAN.2017.02.063
Dai, Y., van Spronsen, J., Witkamp, G. J., Verpoorte, R., and Choi, Y. H. (2013). Natural deep eutectic solvents as new potential media for green technology. Anal. Chim. Acta 766, 61–68. doi:10.1016/j.aca.2012.12.019
Dasgupta, S., Li, J., Chikani-Cabrera, K. D., Machado, P., Fernandes, B., Tapia-Tussell, R., et al. (2022). Improvement in methane production from pelagic sargassum using combined pretreatments. Life (Basel) 12, 1214. doi:10.3390/LIFE12081214
Dumlu, L., Gunerhan, U., Us, E., Yilmaz, V., Carrère, H., Perendeci, A., et al. (2017). Effects of thermal-HCl pretreatment process on biogas production from greenhouse residues Effects of thermal-HCl pretreatment process on biogas production from greenhouse residues Effects of thermal-HCl pretreatment process on biogas production from greenhouse residues No.IWA-503590 METHODS towards a more sustainable world INTRODUCTION. Available at: http://www.AD15.org.cn.
Elbeshbishy, E., Nakhla, G., and Hafez, H. (2012). Biochemical methane potential (BMP) of food waste and primary sludge: influence of inoculum pre-incubation and inoculum source. Bioresour. Technol. 110, 18–25. doi:10.1016/J.BIORTECH.2012.01.025
European Parliament (2017). Texts adopted - 2017 UN climate change conference in Bonn, Germany (COP23) - Wednesday. Available at: https://www.europarl.europa.eu/doceo/document/TA-8-2017-0380_EN.html (Accessed November 3, 2023).
Gautam, P., Kumar, S., and Lokhandwala, S. (2019). “Energy-aware intelligence in megacities,” in Current developments in biotechnology and bioengineering: waste treatment processes for energy generation, 211–238. doi:10.1016/B978-0-444-64083-3.00011-7
Gore, S., Baskaran, S., and Koenig, B. (2011). Efficient synthesis of 3,4-dihydropyrimidin-2-ones in low melting tartaric acid–urea mixtures. Green Chem. 13, 1009–1013. doi:10.1039/C1GC00009H
Gorke, J. T., Srienc, F., and Kazlauskas, R. J. (2008). Hydrolase-catalyzed biotransformations in deep eutectic solvents. Chem. Commun., 1235–1237. doi:10.1039/B716317G
Guo, M., Song, W., and Buhain, J. (2015). Bioenergy and biofuels: history, status, and perspective. Renew. Sustain. Energy Rev. 42, 712–725. doi:10.1016/J.RSER.2014.10.013
Heredia-Guerrero, J. A., Benítez, J. J., Domínguez, E., Bayer, I. S., Cingolani, R., Athanassiou, A., et al. (2014). Infrared and Raman spectroscopic features of plant cuticles: a review. Front. Plant Sci. 5, 305. doi:10.3389/FPLS.2014.00305
Jekayinfa, S. O., Adebayo, A. O., Oniya, O. O., and Olatunji, K. O. (2020). Comparative analysis of biogas and methane yields from different sizes of groundnut shell in a batch reactor at mesophilic temperature. J. Energy Res. Rev. 5, 34–44. doi:10.9734/jenrr/2020/v5i130140
Jhong, H. R., Wong, D. S. H., Wan, C. C., Wang, Y. Y., and Wei, T. C. (2009). A novel deep eutectic solvent-based ionic liquid used as electrolyte for dye-sensitized solar cells. Electrochem Commun. 11, 209–211. doi:10.1016/J.ELECOM.2008.11.001
Kabera, T., and Nishimwe, H. (2019). Systems analysis of municipal solid waste management and recycling system in east Africa: benchmarking performance in Kigali city, Rwanda. E3S Web Conf. 80, 03004. doi:10.1051/E3SCONF/20198003004
Kainthola, J., Kalamdhad, A. S., and Goud, V. V. (2020). Optimization of process parameters for accelerated methane yield from anaerobic co-digestion of rice straw and food waste. Renew. Energy 149, 1352–1359. doi:10.1016/J.RENENE.2019.10.124
Khayum, N., Rout, A., Deepak, B. B. V. L., Anbarasu, S., and Murugan, S. (2020). Application of fuzzy regression analysis in predicting the performance of the anaerobic reactor Co-digesting spent tea waste with cow manure. Waste Biomass Valorization 11, 5665–5678. doi:10.1007/s12649-019-00874-9
Kim, S., and Holtzapple, M. T. (2006). Effect of structural features on enzyme digestibility of corn stover. Bioresour. Technol. 97, 583–591. doi:10.1016/J.BIORTECH.2005.03.040
Li, L., Zhou, W., Wu, H., Yu, Y., Liu, F., and Zhu, D. (2014). Relationship between crystallinity index and enzymatic hydrolysis performance of celluloses separated from aquatic and terrestrial plant materials. Bioresources 9, 3993–4005. doi:10.15376/BIORES.9.3.3993-4005
Madyira, D. M., and Olatunji, K. O. (2023). Investigating the influence of different pretreatment methods on the morphological structure of Arachis hypogea shells. Mater Today Proc. doi:10.1016/J.MATPR.2023.07.289
Millati, R., Syamsiah, S., Niklasson, C., Nur Cahyanto, M., Lundquist, K., and Taherzadeh, M. J. (2011). Biological pretreatment: review.
Monlau, F., Barakat, A., Steyer, J. P., and Carrere, H. (2012). Comparison of seven types of thermo-chemical pretreatments on the structural features and anaerobic digestion of sunflower stalks. Bioresour. Technol. 120, 241–247. doi:10.1016/J.BIORTECH.2012.06.040
Monlau, F., Barakat, A., Trably, E., Dumas, C., Steyer, J. P., and Carrère, H. (2013). Lignocellulosic materials into biohydrogen and biomethane: impact of structural features and pretreatment. Crit. Rev. Environ. Sci. Technol. 43, 260–322. doi:10.1080/10643389.2011.604258
Moutta, R. D. O., Ferreira-Leitão, V. S., and Bon, E. P. D. S. (2014). Enzymatic hydrolysis of sugarcane bagasse and straw mixtures pretreated with diluted acid. Biocatal. Biotransformation 32, 93–100. doi:10.3109/10242422.2013.873795
Moyer, P., Kim, K., Abdoulmoumine, N., Chmely, S. C., Long, B. K., Carrier, D. J., et al. (2018). Structural changes in lignocellulosic biomass during activation with ionic liquids comprising 3-methylimidazolium cations and carboxylate anions 03 Chemical Sciences 0306 Physical Chemistry (incl. Structural). Biotechnol. Biofuels 11, 1–13. doi:10.1186/S13068-018-1263-0/FIGURES/7
Ogunkunle, O., Olatunji, K. O., and Jo, A. (2018). Comparative analysis of Co-digestion of cow dung and jatropha cake at ambient temperature. Res. Article. doi:10.4172/2090-4541.1000271
Okedu, K. E., Barghash, H. F., and Al Nadabi, H. A. (2022). Sustainable waste management strategies for effective energy utilization in Oman: a review. Front. Bioeng. Biotechnol. 10, 825728. doi:10.3389/fbioe.2022.825728
Olatunji, K. O., Ahmed, N. A., and Ogunkunle, O. (2021). Optimization of biogas yield from lignocellulosic materials with different pretreatment methods: a review. Biotechnol. Biofuels 14, 159. doi:10.1186/s13068-021-02012-x
Olatunji, K. O., and Madyira, D. M. (2023a). Anaerobic co-digestion of alkali-pretreated groundnut shells and duck waste for methane yield optimization and sustainable environment. E3S Web Conf. 433, 02005. doi:10.1051/E3SCONF/202343302005
Olatunji, K. O., and Madyira, D. M. (2023b). Effect of acidic pretreatment on the microstructural arrangement and anaerobic digestion of Arachis hypogea shells; and process parameters optimization using response surface methodology. Heliyon 9, e15145. doi:10.1016/j.heliyon.2023.e15145
Olatunji, K. O., and Madyira, D. M. (2023c). Effect of acidic pretreatment on the microstructural arrangement and anaerobic digestion of Arachis hypogea shells; and process parameters optimization using response surface methodology. Heliyon 9, e15145. doi:10.1016/J.HELIYON.2023.E15145
Olatunji, K. O., and Madyira, D. M. (2023d). Optimization of biomethane yield of xyris capensis grass using oxidative pretreatment. Energies 2023 16, 3977. Page 3977 16. doi:10.3390/EN16103977
Olatunji, K. O., Madyira, D. M., and Adeleke, O. (2023a). Optimizing anaerobic co-digestion of Xyris capensis and duck waste using neuro-fuzzy model and response surface methodology. Fuel 354, 129334. doi:10.1016/J.FUEL.2023.129334
Olatunji, K. O., Madyira, D. M., Ahmed, N. A., and Ogunkunle, O. (2022a). Biomethane production from Arachis hypogea shells: effect of thermal pretreatment on substrate structure and yield. Biomass Convers. Biorefin. doi:10.1007/s13399-022-02731-7
Olatunji, K. O., Madyira, D. M., Ahmed, N. A., and Ogunkunle, O. (2022b). Effect of combined particle size reduction and Fe3O4 additives on biogas and methane yields of Arachis hypogea shells at mesophilic temperature. Energies (Basel) 15, 3983. doi:10.3390/en15113983
Olatunji, K. O., Madyira, D. M., Ahmed, N. A., and Ogunkunle, O. (2022c). Influence of alkali pretreatment on morphological structure and methane yield of Arachis hypogea shells. Biomass Convers. Biorefin. doi:10.1007/s13399-022-03271-w
Olatunji, K. O., Madyira, D. M., and Amos, J. O. (2023b). Sustainable enhancement of biogas and methane yield of macroalgae biomass using different pretreatment techniques: a mini-review. Energy Environ. doi:10.1177/0958305X231193869
Olugbemide, A. D., Oberlintner, A., Novak, U., and Likozar, B. (2021). Lignocellulosic corn stover biomass pre-treatment by deep eutectic solvents (DES) for biomethane production process by bioresource anaerobic digestion. Sustain. 2021 13 10504 13, 10504. doi:10.3390/su131910504
Orozco-González, J. G., Amador-Castro, F., Gordillo-Sierra, A. R., García-Cayuela, T., Alper, H. S., and Carrillo-Nieves, D. (2022). Opportunities surrounding the use of sargassum biomass as precursor of biogas, bioethanol, and biodiesel production. Front. Mar. Sci. 8, 2066. doi:10.3389/fmars.2021.791054
Oyedepo, S. O. (2012). On energy for sustainable development in Nigeria. Renew. Sustain. Energy Rev. 16, 2583–2598. doi:10.1016/J.RSER.2012.02.010
Paiva, A., Craveiro, R., Aroso, I., Martins, M., Reis, R. L., and Duarte, A. R. C. (2014). Natural deep eutectic solvents - solvents for the 21st century. ACS Sustain Chem. Eng. 2, 1063–1071. doi:10.1021/sc500096j
Patowary, D., and Baruah, D. C. (2018). Effect of combined chemical and thermal pretreatments on biogas production from lignocellulosic biomasses. Ind. Crops Prod. 124, 735–746. doi:10.1016/J.INDCROP.2018.08.055
Podgorbunskikh, E. M., Bychkov, A. L., Ryabchikova, E. I., and Lomovsky, O. I. (2020). The effect of thermomechanical pretreatment on the structure and properties of lignin-rich plant biomass. Molecules 25, 995. doi:10.3390/MOLECULES25040995
Procentese, A., Johnson, E., Orr, V., Garruto Campanile, A., Wood, J. A., Marzocchella, A., et al. (2015). Deep eutectic solvent pretreatment and subsequent saccharification of corncob. Bioresour. Technol. 192, 31–36. doi:10.1016/J.BIORTECH.2015.05.053
Procentese, A., and Rehmann, L. (2018). Fermentable sugar production from a coffee processing by-product after deep eutectic solvent pretreatment. Bioresour. Technol. Rep. 4, 174–180. doi:10.1016/J.BITEB.2018.10.012
Rabemanolontsoa, H., and Saka, S. (2016). Various pretreatments of lignocellulosics. Bioresour. Technol. 199, 83–91. doi:10.1016/j.biortech.2015.08.029
Raja, I. A., and Wazir, S. (2017). Biogas production: the fundamental processes. Univers. J. Eng. Sci. 5, 29–37. doi:10.13189/ujes.2017.050202
Rimi Abubakar, I., and Aina, Y. (2016). “Achieving sustainable cities in Saudi arabia: juggling the competing urbanization challenges,” in Population growth and rapid urbanization in the developing world, 43–64. doi:10.4018/978-1-5225-0187-9.ch003
Rincón, B., Heaven, S., Banks, C. J., and Zhang, Y. (2012). Anaerobic digestion of whole-crop winter wheat silage for renewable energy production. Energy Fuels 26, 2357–2364. doi:10.1021/EF201985X
Sarbishei, S., Goshadrou, A., and Hatamipour, M. S. (2021). Mild sodium hydroxide pretreatment of tobacco product waste to enable efficient bioethanol production by separate hydrolysis and fermentation. Biomass Convers. Biorefin 11, 2963–2973. doi:10.1007/S13399-020-00644-X
Şenol, H. (2021). Effects of NaOH, thermal, and combined NaOH-thermal pretreatments on the biomethane yields from the anaerobic digestion of walnut shells. Environ. Sci. Pollut. Res. 2021 28, 21661–21673. 1728. doi:10.1007/S11356-020-11984-6
Shahid, M. K., Kashif, A., Rout, P. R., Aslam, M., Fuwad, A., Choi, Y., et al. (2020). A brief review of anaerobic membrane bioreactors emphasizing recent advancements, fouling issues and future perspectives. J. Environ. Manage 270, 110909. doi:10.1016/J.JENVMAN.2020.110909
Shen, X. J., Chen, T., Wang, H. M., Mei, Q., Yue, F., Sun, S., et al. (2020). Structural and morphological transformations of lignin macromolecules during bio-based deep eutectic solvent (DES) pretreatment. ACS Sustain Chem. Eng. 8, 2130–2137. doi:10.1021/acssuschemeng.9b05106
Smith, E. L., Abbott, A. P., and Ryder, K. S. (2014). Deep eutectic solvents (DESs) and their applications. Chem. Rev. 114, 11060–11082. doi:10.1021/cr300162p
Song, Z., Yang, G., Guo, Y., and Zhang, T. (2012). Comparison of two chemical pretreatments of rice straw for biogas production by anaerobic digestion. Bioresources 7, 3223–3236. doi:10.15376/biores.7.3.3223-3236
Substratcharakterisierung, V. (2016). Verein deutscher ingenieure characterisation of the substrate, sampling, collection of material data, fermentation tests vdi 4630 vdi-richtlinien. Available at: www.vdi.de/richtlinien.
Taiwo, A. (2009). Waste management towards sustainable development in Nigeria: a case study of Lagos state. Int. NGO J. 4, 173–179. Available at: http://www.academicjournals.org/INGOJ (Accessed November 3, 2023).
Van Soest, P. J., Robertson, J. B., and Lewis, B. A. (1991). Methods for dietary fiber, neutral detergent fiber, and nonstarch polysaccharides in relation to animal nutrition. J. Dairy Sci. 74, 3583–3597. doi:10.3168/JDS.S0022-0302(91)78551-2
Venturin, B., Frumi Camargo, A., Scapini, T., Mulinari, J., Bonatto, C., Bazoti, S., et al. (2018). Effect of pretreatments on corn stalk chemical properties for biogas production purposes. Bioresour. Technol. 266, 116–124. doi:10.1016/j.biortech.2018.06.069
Xu, H., Peng, J., Kong, Y., Liu, Y., Su, Z., Li, B., et al. (2020). Key process parameters for deep eutectic solvents pretreatment of lignocellulosic biomass materials: a review. Bioresour. Technol. 310, 123416. doi:10.1016/J.BIORTECH.2020.123416
Xu, N., Liu, S., Xin, F., Zhou, J., Jia, H., Xu, J., et al. (2019). Biomethane production from lignocellulose: biomass recalcitrance and its impacts on anaerobic digestion. Front. Bioeng. Biotechnol. 7, 191. doi:10.3389/fbioe.2019.00191
Yang, Q., Fu, L., Liu, X., and Cheng, M. (2018). Evaluating the efficiency of municipal solid waste management in China. Int. J. Environ. Res. Public Health 15, 2448. doi:10.3390/IJERPH15112448
Yildiz, L. (2018). 1.12 fossil fuels. Compr. Energy Syst. 1–5, 521–567. doi:10.1016/B978-0-12-809597-3.00111-5
Zhang, H., Zhang, P., Ye, J., Wu, Y., Fang, W., Gou, X., et al. (2016). Improvement of methane production from rice straw with rumen fluid pretreatment: a feasibility study. Int. Biodeterior. Biodegrad. 113, 9–16. doi:10.1016/j.ibiod.2016.03.022
Zhang, Q., Tang, L., Zhang, J., Mao, Z., and Jiang, L. (2011). Optimization of thermal-dilute sulfuric acid pretreatment for enhancement of methane production from cassava residues. Bioresour. Technol. 102, 3958–3965. doi:10.1016/J.BIORTECH.2010.12.031
Zhao, X., Zhang, L., and Liu, D. (2012). Biomass recalcitrance. Part I: the chemical compositions and physical structures affecting the enzymatic hydrolysis of lignocellulose. Biofuels, Bioprod. Biorefining 6, 465–482. doi:10.1002/BBB.1331
Keywords: lignocellulose feedstocks, groundnut shells, pretreatment, deep eutectic solvents, microstructural arrangements, biomethane
Citation: Olatunji KO and Madyira DM (2024) Enhancing the biomethane yield of groundnut shells using deep eutectic solvents for sustainable energy production. Front. Energy Res. 12:1346764. doi: 10.3389/fenrg.2024.1346764
Received: 29 November 2023; Accepted: 29 January 2024;
Published: 19 February 2024.
Edited by:
Sunday Olayinka Oyedepo, Bells University of Technology, NigeriaReviewed by:
Joseph Oyebanji, Bells University of Technology, NigeriaAugustine Omoniyi Ayeni, Covenant University, Nigeria
Copyright © 2024 Olatunji and Madyira. This is an open-access article distributed under the terms of the Creative Commons Attribution License (CC BY). The use, distribution or reproduction in other forums is permitted, provided the original author(s) and the copyright owner(s) are credited and that the original publication in this journal is cited, in accordance with accepted academic practice. No use, distribution or reproduction is permitted which does not comply with these terms.
*Correspondence: Kehinde O. Olatunji, b2xhb2xhZG9rZTI5M0BnbWFpbC5jb20=