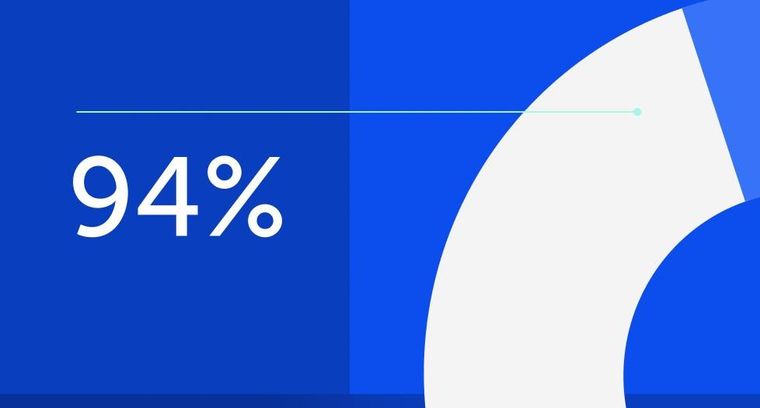
94% of researchers rate our articles as excellent or good
Learn more about the work of our research integrity team to safeguard the quality of each article we publish.
Find out more
ORIGINAL RESEARCH article
Front. Energy Res., 02 August 2024
Sec. Sustainable Energy Systems
Volume 12 - 2024 | https://doi.org/10.3389/fenrg.2024.1336016
This article is part of the Research TopicSupply Chain Transformation for Pursuing Carbon-neutralityView all 10 articles
A new concept based on Power Plant Units, able to deliver renewable energy on demand, for the transition from fossil fuels to renewable energy in Switzerland is presented. The technically realized efficiencies showed that complete electrification leads to the most efficient energy system and cheapest electricity. The electricity demand is expected to almost double, and the overall energy cost will increase by 20% compared to 2019. However, the technical challenges of seasonal electricity storage, without any reserves and redundancy, amounts to 20 TWh. Hydropower and PV without storage produce the cheapest electricity. Future nuclear fission technologies, e.g., molten salt Thorium breading reactor - currently still in an experimental stage–might become the most economical and least environmental impact solution for CO2 neutral continuous electricity production. The opportunities for a massive increase of hydroelectric production are limited, already shifting the use of water (9 TWh) from summer to winter is a great challenge. PV and hydrogen production in Switzerland have the advantage to provide approximately 75% of the electricity without seasonal storage leading to significantly lower electricity cost than from imported hydrogen or synthetic hydrocarbons. The most economical solution for aviation and reserves is imported bio-oil converted to synthetic Kerosene, for which large storages already exist.
GRAPHICAL ABSTRACT | Future supply of Switzerland with renewable energy. Assuming the volume of the hydroelectric storage lakes is doubled, the roof area is covered with photovoltaics, and eight power plant units are able to produce 1 GW on demand and are fueled with hydrogen or bio-oil. The reserves are the existing oil tanks, and the bio-oil is also used for aviation. Bio-oil can be produced in abundant places, e.g., Australia or Africa, where palm oil plantations are installed.
• Renewable energy on demand is essential for replacing fossil fuels and can be realized by combining intermittent energy supplies like photovoltaic and wind with battery and seasonal storage in a power plant unit.
• Importing renewable energy carriers requires a storage capacity similar to the seasonal storage for domestic production of renewable energy.
• Renewable energy production in Switzerland with seasonal storage and importing renewable energy carriers is a technical and economic challenge, respectively.
• The fuel for aviation and the energy reserves for the power plant units can be realized with synthetic oil produced by hydriding bio-oil, avoiding the need for new large and expensive storage systems and CO2 capture from the atmosphere.
• Thermal power plants fueled with renewable energy carriers provide equal amounts of electricity and heat. Both forms of energy are of high value in the wintertime.
Industrialization has led to a global economic trading system and increased global wealth by orders of magnitude. The use of fossil energy to run the engines has two important consequences, i.e., the emission of carbon stored in the earth’s crust to the atmosphere as CO2 (Figure 1) and the consumption of fossil fuel reserves several orders of magnitude faster than their formation, which leads to a depletion of these fuels. Besides the biological origin of the fossil fuels, hydrocarbons can also be formed deep under the surface by other non-plant-based processes (Thomas, 1992). As a consequence, the amount of hydrocarbon reserves may be larger than estimated today. Only around 50% of the emitted CO2 remains in the atmosphere (Sarmiento and Gruber, 2002). The other 50% is absorbed by natural sinks (Friedlingstein et al., 2022), e.g., the ocean and geological and biological processes (Springer, 1992). The CO2 absorbing processes depend on the concentration of CO2 in the atmosphere, and the absorption rate increases with increasing concentration (Canadell et al., 2007). The CO2 concentration in the atmosphere increases linearly with the cumulated CO2 emissions. In any case, fossil fuels have to be substituted by renewable energy in a sustainable manner, i.e., by carbon-neutral renewable energy. To provide energy on demand, the main challenges are the production of electricity and heat from renewable energy as well as local and seasonal storage. In addition, non-fossil aviation fuel needs to be produced in future.
Figure 1. Global average temperature as a function of the cumulated CO2 emissions observed. The fitted curve corresponds to ΔT [°C] = 2.85·ln (c/c0) with c0 = 286.6 ppm CO2 (blue line, extrapolation: blue dotted line) the radiative forcing of the CO2 (Appendix 4). The years are indicated (red markers. The end of the reserves, according to Campbell (Campbell and Laherrere, 1998) (900 Gt C) and proven reserves (Friedlingstein et al., 2022) (1750 Gt C), are indicated with the year assuming a continuous growth of the usage of fossil fuels and CO2 emissions of 1.5%/year.
After the Fukushima Dai-ichi nuclear-plant disaster caused by the Tsunami due to the earthquake on 11. March 2011, the Swiss Federal Council has decided not to rely on nuclear power in the future. The existing nuclear power plants should remain connected to the grid for as long as they are safe. This decision was confirmed by the national parliament on 8. June 2011. On 21. May 2017 the Swiss electorate accepted the revised Federal Energy Act. The aims behind the revision are, according to the Paris climate agreement from 2014, to reduce energy consumption, increase energy efficiency and promote the use of renewable energy. In addition, the revised version prohibits the construction of new nuclear power plants. Switzerland’s Long-Term Climate Strategy (Bafu, 2024) includes the reduction of greenhouse gas emissions as far as possible in every sector–whether through a sufficiently high price for emission-intensive technologies, through technical measures or by promoting alternatives. The buildings and transport sectors can cut their fossil emissions to zero by 2050 and energy-related emissions can also be almost completely eliminated in industry too.
The Swiss railway investigated in 1912 the situation of the railway development based on coal fired steam engines and concluded (Berichthaus, 1912), that the railway will be electrified and the steam locomotives will be replaced by electrical locomotives. Therefore, numerous hydroelectric power plants were built in the following years in order to provide the electricity for the railway and all other applications. The current energy economy based on renewable hydropower and nuclear power for electricity and fossil fuels, is able to deliver power and energy on demand. With the substitution of fossil fuels with renewable energy, i.e., photovoltaics and wind, the energy system becomes more and more production controlled and a significant gap between production and demand is opening. Therefore, renewable energy storage has to be introduced in order to close the gap and provide energy on demand from renewable energy sources.
The potentials, costs and environmental assessment of electricity generation technologies was published in 2017 as a report (PSI, 2023). The maximum renewable generation potentials in Switzerland was estimated to be 18 TWh·y-1 for roof-top photovoltaics (PV), additional to existing (36 TWh·y-1) hydropower 4.5 TWh·y-1, geothermal 4.5 TWh·y-1, additional biomass and wind 4 TWh·y-1, each. These estimates were compared to several other available published data with a close agreement. The PV installations in Switzerland are growing (Swissolar, 2024) and reached 2023 an installed peak power of 1.3 GWp·y-1, with a total PV electricity production of 4.8 TWh·y-1. A detailed analysis (Walcha et al., 2020) of the available roof-top area in Switzerland for PV installations (150 km2) showed a potential PV electricity production of 24 ± 9 TWh·y-1.
Biogenic and nonbiogenic carbon footprints in Switzerland were quantified, and the circular economy associated with a net-zero-emission society was optimized (Xiang et al., 2020). The impacts of an increased substitution of fossil energy carriers with electricity-based technologies in Switzerland was analyzed (Rudisuli et al., 2019). The complete electrification of the mobility and domestic heating with heat pumps leads to an increased electricity demand of 32 TWh·y-1 and an additional electricity power demand of 6 GWp are needed. An annual electricity deficit and surplus of 24.1 TWh·y-1 and 7.5 TWh·y-1, respectively, will result. With (perfect) seasonal-storage, surpluses could be eliminated completely, while deficits would remain at 15.9 TWh·y-1, which is the absolute difference of the annual electricity consumption and production.
The seasonal storage requirement in Switzerland is a technical and economic challenge and contributes significantly to the cost of the energy. However, without the storage the energy consumer has to adapt to the renewable energy supply (Desing and Widmer, 2022) like sunflowers do. Adding energy storage significantly reduces the attainable speed of the energy transition due to its high energy costs. Combining supply side measures, such as sector coupling and smart grids, with aligning demand patterns to solar supply can reduce the storage requirement at least for short term storage.
Replacing the fossil fuels with bio oil or with hydrogen upgraded oxygen free bio oil has the potential to realize the energy transition to a CO2 neutral energy economy on a global level. At present, global forest carbon storage is markedly under the natural potential, with a total deficit of 226 Gt (model range = 151–363 Gt) in areas with low human footprint (Mo et al., 2023). Therefore, all the CO2 in the atmosphere emitted from fossil fuels could be absorbed by increasing the number of trees. Furthermore, with 142 oil palm trunk (OPT) per ha of plantation land and a replanted area of 100′550 ha in 2017, the estimated dry weight of OPT (74.48 t ha-1) generated amounted to a total of 7.49 Mt (Pulingam et al., 2022). 4.0 t ha-1·y-1 palm oil (Sustainablepalmoilchoice, 2024) is produced and the oil palm plants are replanted every 20 years. Therefore, 30 kg oil·y-1 per oil palm tree with 524 kg dry biomass. The 226 Gt C realized with oil palm trees produce 13 Gt oil·y-1 more than the current world demand of fossil fuels!
Based on the energy demand of 2019, the substitution of fossil fuels in Switzerland was analyzed with three energy systems in (Figure 2, Figure 3) view of the energy production and storage requirements (Züttel et al., 2022). The electricity produced by the current nuclear power plants with Uranium fission reactors is replaced with electricity from photovoltaics, the kerosene for aviation is substituted with CO2-neutral kerosene produced from renewable energy and CO2 air capture or biomass, and the remaining energy demand is covered either by electricity, hydrogen, or synthetic hydrocarbons.
Figure 2. Summary of the end energy demand in Switzerland of 232 TWh·y-1 (2019, left hand side)) and after electrification 156 TWh·y-1 (right hand side) of the applications, e.g., mobility and heating. Imported, exported and lost electricity are not shown in the figure but accounted for in the sum. The sectors (clock wise) are Hydroelectricity (blue), nuclear (orange), PV and wind (yellow), heating oil (dark gray), petrol and diesel (light gray), natural gas (white), distance heating (brown), coal (black), kerosene (violet), Biomass (green). Based on only technical energy conversion efficiency increase, the energy demand of the electrified energy economy decreases approximately 33% (ZÜTTEL et al., 2022)
Figure 3. The three energy systems presented for the complete substitution of fossil fuels with renewable energy. The PV surface area is shown as the roof area in Switzerland. The battery represents the local day/night storage and the seasonal storage as compared to Grande Dixence storage lake, the volume of the Gotthard base tunnel, and barrels of oil (ZÜTTEL et al., 2022). The total annual cost of energy per capita is indicated on top; currently the energy cost per capita amounts to 3,000.-/year.
The main technical challenges in substituting fossil fuels are the production of electricity and seasonal storage to shift 20%–30% of the annual energy production from summer to winter. For comparison, an analysis of the hourly energy demand in Germany over the last 35 years, assuming complete coverage with renewable energy, resulted in a storage requirement of 10% of the annual electricity demand (540 TWh·y-1 without substitution of the fossil fuels, 3,000 TWh·y-1 with electricity). The scarcity period defining storage requirements extends over as much as 12 weeks (Ruhnau and Qvist, 2022).
While the electric energy chain is the most efficient, storage is technically demanding and expensive at low energy density. The conversion of electricity to an energy carrier is less efficient due to conversion losses but facilitates the storage, increases the energy storage density, and reduces the cost of storage. Finally, the form of energy that offers the necessary energy density at the lowest possible cost is the most favorable for a future energy system and depends significantly on the application.
The energy transition to a fossil-free energy economy requires the generation of end energy (3.2 kW·capita−1) from renewable energy. Currently, 0.86 kW·capita−1 is produced from renewable generation including biomass, and the electricity production from nuclear power corresponds to 0.24 kW·capita−1. The nonrenewable end energy is 2.02 kW·capita−1.
Approximately 75% of the energy demand can be covered with renewable electricity at a cost comparable to today’s energy cost. The remaining 25%, i.e., the winter months, are technically and economically challenging. Due to the concave shape of the energy demand and the convex shape of the renewable energy production from January to December, the renewable energy has to provide band energy, and the storable forms are used to adapt production to demand.
The PV area for an electricity-based system, including aviation fuels, is around 80 m2·capita−1. For a hydrogen-based energy system, this area becomes twice as large, and for a synthetic hydrocarbon-based energy system, the PV area again almost doubles. However, building 13 hydroelectric power plants like Grande Dixence or a facility 25 times the Gotthard base tunnel for hydrogen storage are constructions beyond everything Switzerland has realized so far.
Feasible technical solutions based on low-carbon technologies, efficiency, and flexibility were analyzed (Panos et al., 2023). Import independency and net-zero emissions by 2050 require an additional cumulative discounted investment of 300 billion CHF2019 in energy efficiency, negative emissions and renewable technologies. The electricity in each net-zero scenario in 2050 is estimated to be between 73 and 102 TWh·y-1, with an electricity storage requirement of only 0.52 TWh and demand-side response. Possible technology paths for Switzerland to respond to the finite availability and to meet international climate goals were assessed (Brethauer and Hans-Peter Studer, 2021), a change to a renewable energy and raw material basis is inevitable. Biomass is the most versatile renewable energy and carbon source, although with a limited availability.
The integration of renewable energies into the electricity sector from social, environmental, and economic view point was reviewed (Osman et al., 2023) with a special focus on hybrid systems. Renewable energy shows a great potential in reducing the overall CO2 emission, however, the various sources of renewable energy exhibit different effects on the CO2 emission (Yuan et al., 2022). The potential of the conversion of biomass to bio fuels including life cycle assessment was reviewed (Osman et al., 2021) with the conclusion that comparing thermochemical and biological processes for the same biomass feedstock and geographical and temporal span, thermochemical processes caused less greenhouse gas emissions compared to biological path-ways. The global transition from fossil to renewable energy was investigated and it was calculated that the world would need to spend around US$62 trillion to build up the wind, solar, and hydro power generating capacity to fully meet demand and completely replace fossil fuels (Jacobson et al., 2022). However, a rapid transition to green energy sources such as wind and solar power could save anywhere between US$5 to US$15 trillion compared to taking no action in 80% of scenarios modelled and could save even more money if green technology continues to improve (Way et al., 2024).
The hydropower plants with storage lakes are the only technically realized and economically competitive large-scale electricity storages available today. However, hydroelectric power plants are only possible in some designated areas, where mountains offer the altitude difference and valleys the storage room. The research work on the assessment of renewable energy focusses mainly on the energy or electricity production and not on delivering energy on demand, with the consequence that these analyses do not allow to determine the consequences of the substitution of fossil fuels with renewable energy but rather assume the availability of some fossil fueled power plants to fill the energy cap. In this work we present a new approach, where we investigate renewable energy not as a production-controlled energy source but offering renewable energy on demand. A sustainable approach is to combine renewable energy conversion with appropriate storage that allows delivery of renewable energy on demand. This includes short term (day/night) storage as well as seasonal storage. In addition, adapting the renewable energy production to the seasonal energy demand pattern and installing storage for imported renewable energy carriers will increase energy security by having redundancy and reserves.
First, we are identifying the increase of electricity demand in the future Swiss energy economy under the assumption that the energy system is going to be electrified. Then a new approach for renewable energy systems is introduced called power plant units (PPUs). The PPU’s decouple the energy production and the energy delivery by an energy storage and provide energy on demand. PPU’s are able to produce 1 GW electricity on demand at any time of the year. The question on how the energy demand can be covered with renewable energy will be addressed. Furthermore, the PPU’s allow to compare renewable energy solutions independent of the local requirements and lead to a general applicable solution. For each possible solution the technical requirements are described and the economic analysis of the energy chain is provided. Therefore, the cost of the renewable energy for each individual solution is estimated in view of capital cost (investment), operational cost and levelized cost of energy.
Each power plant unit consists of a series of components (Ci) transferring the energy from the source to the grid or to an energy carrier, e.g., synthetic hydrocarbon. The components transfer the energy (Wi) with a specific efficiency (ηi) and may also consume auxiliary electricity (Ei). Therefore, the finally available energy at the end of the conversion chain is
The sum of the auxiliary electricity (ΣEi) is added to the last electric component in the conversion chain. The total efficiency is given by
Based on the power and the time or energy and cycle number the total amount of energy transferred through the components is determined during the entire lifetime of the component. The cost for each individual component, the capital cost (CAPEX) is assumed to be amortized during the whole lifetime (n years) and an interest of Z = 2%/year on the capital. The constant annual payback, Pb, can be calculated from the cost series
and the specific cost contribution per energy unit is
The sum of all cost contributions of the components multiplied with the transferred energy corresponds to the cost of the delivered energy of the conversion chain.
This general model allows to determine the correct size of all the components in the energy conversion chain and leads to the true cost of the finally delivered energy, i.e., the levelized cost of energy (LCOE).
The future developments are changing the energy demand, e.g., energy saving measures are expected to reduce the energy demand per capita. Also, the efficiency of energy converters is increasing. The efficiency of the PV panels has the potential to increase in the future by up to 50%. The energy demand for mobility can be partially covered by coating the roof area of cars (4–8 m2) with PV producing the energy to drive 4,000–8,000 km y-1; 50% of the average annual driving distance in Switzerland. The climate change leading to increased temperatures lowers energy demand for heating in winter, while air conditioning becomes necessary when high solar intensity provides PV-electricity in summer.
Population growth and increasing wealth will increase energy demand. The general trend of most countries is a linear relationship between energy demand per capita and GDP per capita with a slope of 0.6 CHF/kWh (Figure 4), especially in countries with a GDP <25,000 CHF/capita (>75% of the world population (Wir, 2022)). However, a new trend exhibiting increasing GDP/capita while the energy demand/capita is decreasing is observed in many countries and especially in most industrialized countries. This effect is very positive for the future development of the world. However, it is still questionable whether this trend is going to take place in all countries, especially on the continents of Asia and Africa, which will represent more than 70% of the world population and is expected to reach 10 Billion capita in 2050 (Lutz et al., 2001).
Figure 4. Primary energy demand per capita versus GDP per capita over the last 30 years for selected countries (Ourworldindata, 2024).
The electrification of the applications, i.e., battery electric mobility, heating with heat pumps, air conditioning, information technology, etc., requires stable electricity production on demand. The electricity demand is expected to almost double from the current demand in Switzerland when the electrification is completed in 2050. In order to cover the electricity demand in the electrified energy economy, an additional 53 TWh·y-1 are required. For the decided replacement of the nuclear power plants running on Uranium fission, an additional 21 TWh·y-1 is required. In total, 74 TWh·y-1 more renewable electricity will be requested than is produced today. The electricity demand will increase from 62 TWh·y-1 in 2019 to 113 TWh·y-1. As a consequence, in the coming 30 years, an additional +2.4 TWh·y-1, approximately the production of Grande Dixence storage lake or 4 times Grengiols alpine PV-field in its projected size, will have to be installed every year. Estimations assuming additional energy savings beyond the technical efficiency gains lead to an annual electricity demand (VSE/AES, 2024) between 80 and 90 TWh·y-1 in 2050. This lower electricity demand in 2050 will be due to the import of electricity (7 TWh), the lack of storage, and the assumption that backup power plants (10 TWh) will cover the need in case the renewable energy production is not sufficient.
Renewable energy appears in a variety of qualities. While solar energy (solar thermal, photovoltaics, solar concentrator) and wind are intermittent sources of energy, geothermal power plants deliver heat continuously. The precipitation used by hydroelectric power plants depends on the type of installation and can provide storage in the mountain lakes. Furthermore, biomass is a storable form of renewable energy and even represents a CO2 neutral carbon source. Tidal power goes through predictable oscillations, but it is only available in certain regions of the earth and not in Switzerland. Finally, geothermal energy provides heat continuously (independent of the season). Its extraction is technically challenging in Switzerland due to the low intensity.
Harvesting renewable energy requires more than just converters to provide electricity. Transport, local and seasonal storage is necessary to turn an intermittent renewable source of energy into band energy to deliver on demand.
A power plant unit (PPU) represents an energy system able to deliver 1 GW of electricity on demand or approx. 8.7 TWh·y-1 of electricity per year and with the flexibility to adapt the produced power to meet the electricity demand. In a future energy economy, where most applications are electrified, the grid has to provide reliably electricity on demand (Figure 5). This is only possible if the entire electricity system has the necessary reserves and redundancy. An energy system that works according to the sunshine (Desing and Widmer, 2022) or is dependent on importing electricity (Empa, 2024) is unstable and decreases the productivity of the economy drastically due to the delays and interruptions caused by a lack of power. Only a reliable energy supply allows human beings to develop prosperity.
Figure 5. Renewable electricity production (BFE, 2024) together with the electricity demand in Switzerland and the expected growth of the electricity demand in future due to the electrification of applications. The difference between renewable production and demand is currently filled with nuclear power in Switzerland and imported electricity (from nuclear, coal, and gas fired power plants). Above the electricity are the fossil fuels, heating oil (dark grey), fuel for mobility (middle grey) and natural gas (light grey).
Considering the monthly energy demand in Switzerland (Figure 6) and the transition of the current energy system to an electrified energy system based on renewable energy, electricity production has to be adapted to the increased demand in winter by the following measures:
1) The height of the dams on the storage lakes is increased by 26% in order to double the storage capacity of the lakes and to shift 9 TWh of electricity produced during the summer to winter.
2) Most of the currently used biomass (15 TWh·y-1) and an additional 5 TWh·y-1 of biomass (Burg et al., 2018) is used for heating.
3) All feasible roof areas are covered with photovoltaics producing 24 TWh·y-1, according to Walch et al. (Walcha et al., 2020)
4) The remaining missing band electricity of 53 TWh·y-1 is produced by 6 Power Plant Units (PPUs).
5) At least two additional PPUs are built for redundancy and supply security.
6) Aviation fuel is produced either by 3 Power Plant Units (PPUs) or imported bio-oil based synthetic fuel.
Figure 6. (A) Current Swiss energy system (2019) with contributions from the different sources of energy:
These six measures provide renewable electricity on demand and synthetic fuel for the airplanes without the need for direct air capture of CO2 (Figure 6B). The two backup PPU’s represent a redundancy and reserve of 33% or 4 months of the year. The first three measures can also be replaced by PPU’s, however, they would then only run part time of the year or at a fraction of the nominal power.
A PPU is an energy system able to produce energy on demand rather than intermittent energy given by the natural occurrence of energy flow. Hydroelectric power plants with a storage lake are able to store a large amount of energy and deliver electricity on demand. Fossil fuels and nuclear power plants are operated continuously to provide band energy. Only gas-fired combined cycle power plants burning gas with a large gas storage capacity are able to produce electricity on demand. A PPU has to be able to deliver the band electricity and, in the ideal case, it is also able to follow the electricity demand. Based on our previous energy analysis, the day-night storage and the seasonal storage correspond to 0.25% and 25% of the annual electricity demand, respectively. This storage also covers the fluctuations in demand between day and night as well as the increased demand in winter Table 1.
Table 1. The 10 different PPUs provide >8 TWh·y-1 of electricity all the time and on-demand, except for Bio-SF, Imp.-SF and Imp. PSF these deliver >8 TWh·y-1 as synthetic fuel (synthetic hydrocarbons). PV area is active area, the PV field would be approximately 2–2.5 times larger. The levelized cost of energy (electricity) (LCOE) is given in the last column.
Hydroelectric power plants with a storage lake 500 m above the turbine are able to produce electricity on demand with up to >1 GW of power and an efficiency >80% between the potential energy in the stored water and the electricity delivered. Approximately 1 m3 of stored water with a height difference of 500 m produces one kWh of electricity. Some of the hydroelectric power plants work between two lakes and are able to pump water up and store electricity. The HYD-S PPU, with a height difference of 500 m, has a lake with a volume of 4,000 Mm3 (approximately the volume of the lake of Zürich). The area to collect the precipitation amounts to 5,800 km2.
Hydroelectric power plants in rivers exhibit a small height difference and profit from the large flow of water. Serial installations of river hydropower plants are often found where the height difference allows it. A HYD-R PPU consists of at least 22 hydropower plants in a river with a flow of 500 m3 s-1 leading to a power of 45 MW each.
Modern thermal power plants fueled with oil or gas are combined cycle power plants (CCPP), where the fuel powers a combustion turbine connected to a generator. The exhaust gas is used to produce steam which is then powers a steam turbine connected to another generator. The modern CCPP reaches an efficiency (Kotowicz and Brzęczek, 2018) from 44% to >60% from fuel to electricity and the remaining heat is available for heating. A THERM PPU requires 112 t h-1 of natural gas (CH4) or 146 t h-1 of petrol, oil or kerosene and emits 2.7 Mt CO2·y-1 or 4 Mt CO2·y-1, respectively.
The uranium fission reactors have some disadvantages in terms of safety, uranium resources, and the long living isotopes as fission products (nuclear waste). Future nuclear power plants may overcome the disadvantages of the current nuclear reactors. A NUC PPU is a nuclear power plant that delivers a constant power of >1 GW and consumes <30 t y-1 of nuclear fuel per year. The conversion from nuclear fuel to electricity exhibits an efficiency of around 25%. Therefore, >3 GW of heat are produced, available for a centralized heating network. The molten salt (MSR) Thorium nuclear breeding reactors (Woodhead Publishing, 2017) are after first tests in the middle of the 20th century in the US again under development and China has commissioned an experimental reactor with a power of 2 MWth. in July 2023 (Sinap, 2023). The advantages of the MSR are the possibility to use the nuclear waste from Uranium fission reactors as a fuel leaving almost only short living isotopes, the general flexibility in nuclear fuel and higher efficiency. The technical and economic estimations are based on the conventional Uranium-fission reactors and may be different for the future Thorium-MSR.
The PV-HYD uses a photovoltaic (PV) field for electricity production. Due to the intermittence of solar energy, a PV installation with an annual average power of 1 GW exhibits a peak power of 8 GW and a daily average power of 3 GW in summer. Therefore, local battery storage for peak saving and day/night storage with a capacity of 0.4% of the annual energy production is required in order to reduce the peak power in the grid and to provide electricity during the night. Furthermore, a hydroelectric storage power plant is used for seasonal storage. The electricity consumed during midday in summer is cheap, while the electricity at midnight in winter becomes most expensive. The PV-HYD PPU consists of a 53 km2 PV active PV area, which corresponds to >100 km2 PV field (greater than the area of the lake of Zürich), a battery capacity of 45 GWh, and an additional 25% of HYD-S PPU with pump storage capability.
The PV-H2 is similar to PV-HYD except for the seasonal storage that is realized with underground hydrogen storage and a combined cycle power plant. Due to the conversion efficiency of the CCPP and the hydrogen production by electrolysis, the PV active area is 86 km2, corresponding to a PV field of >170 km2 (twice the area of the lake of Zürich). The underground storage of 4.4 TWh hydrogen (112′000 t H2) under a pressure of 200 bar is around 7 Mm3, a sphere with a diameter of 240 m. The CCPP with up to 1 GW of electric power delivers electricity and the same amount of energy in form of heat.
The use of biomass, which consists of CO2 absorbed from the atmosphere (KEITH, 2009), as carbon and hydrogen sources is much more efficient and less costly than direct air capture (DAC). According to the overall reaction (CH-OH)n + (n+1)H2 ➝ (CH2)nH2 + nH2O the synthetic hydrocarbons can be produced from reacting biomass with hydrogen, and water is formed as a by-product. The technical process works in two steps: the conversion of biomass to syngas and the Fischer-Tropsch synthesis to produce hydrocarbons. The ideal reaction produces 1 kg of synthetic hydrocarbon from 2.1 kg of biomass (wood) and consumes 0.14 kg of hydrogen (5.6 kWh) produced by PV. The upper heating value of biomass (dry wood) (Demirbaş, 1997; Sheng and Azevedo, 2005) is 4.8 kWh·kg-1 and that of synthetic hydrocarbons (kerosene) is 12.83 kWh·kg-1. Therefore, the energy in biomass and the energy in synthetic hydrocarbons is almost equal in the ideal process. In order to produce 23 TWh of synthetic aviation fuel, the biomass currently used for heating (Bfe, 2024) (16 TWh) plus additional available biomass (Burg et al., 2018) (7 TWh) are needed.
The IMP-H2 PPU is based on imported hydrogen produced in places with a high solar intensity and available space outside of Switzerland. The H2-CCPP consumes 448,000 t y-1 H2 in order to continuously deliver 1 GW of electricity and 1 GW of heat. A transport system delivering 1,200 t H2 per day needs to be built, and for redundancy, a storage of the same size as for PV-H2 is required, which corresponds to a reserve of about 3 months. The imported hydrogen is transported on the sea in liquid form. Storage of liquid hydrogen is 4 times more compact than pressurized hydrogen (200 bar). A liquid hydrogen storage unit of 1.6 Mm3, a sphere with a diameter of 150 m, holds 112,000 t H2.
Hydrogen is produced from PV electricity (s. PV-H2) and CO2 is directly captured from air. The CO2 is reduced to CO with hydrogen and syngas (CO + 2H2) is converted into synthetic oil by the Fischer-Tropsch synthesis (s. Bio-SF). The heat produced in the exothermal reaction of the FT-synthesis is used for the DAC.
Palm oil as starting product for synthetic oil has many advantages over stark based biomass, because of the low oxygen content and the large degree of saturation. The oil palm is a very efficient plant and produces 4.5 t/ha of oil with an energy content of 10.8 kWh·kg-1. The palm oil is catalytically hydrided and cracked with hydrogen under pressure. The complete hydriding of palm oil reduces the mass by 15% but conserves the energy in the oil due to the addition of the energy in the hydrogen, resulting in 12.8 kWh/kg.
The cost of the electricity produced by the PPUs is determined by a model energy chain consisting of all components in the energy conversion from the input energy to the electricity output. Each component has a specific efficiency for the energy transferred, and the size is determined by the energy for storage or energy per time (i.e., power) for transformation. In addition, components that require additional energy or electricity for the storage or transformation add this electricity to the electric part of the energy chain.
For each component, the capital cost (CAPEX) including the interest (Z) on the capital cost with a constant annual payback (Pb: Eq. 3) over the lifetime is added to the operational cost (OPEX) leading to an additional energy cost per component (Cc: Cc = Pb + OPEX). Finally, the amount of energy (Etot) transferred during the lifetime (n) of the component is considered, and the specific cost per energy unit (CE: CE = n·Cc/Etot) is calculated. The specific costs along the energy chain are multiplied with the energy transferred at the component and summed up in order to be divided by the available energy at the output, leading to the specific energy cost of the final energy. The costs of the components are based on current minimum costs, which are expected to decrease in future for most of the components.
Figure 7 shows the cost of the energy (Changeoracle, 2022) (electricity and synthetic fuel) at the production site, i.e., without the grid cost which adds around 0.09 CHF/kWh, for summer and winter, i.e., without and with seasonal storage. The classical renewable energy from hydroelectric power exhibits a constant cost over the year, like the thermal and nuclear power plants. PV together with a hydroelectric storage plant or with hydrogen production and storage lead to an electricity cost increase in summer by a factor of three and in winter by factor of times 6 and 20 higher, respectively, when compared to the electricity cost of the hydroelectric power plant. The cost of electricity from imported hydrogen is independent of the season, it is always 1.02 CHF/kWh. The cost of synthetic fuel produced in Switzerland from wood is 6.2 CHF/L, while the imported synthetic fuel produced from PV with CO2 from DAC and FT-synthesis costs 10.8 CHF/L. Imported synthetic oil from Palm oil cracked with hydrogen produced by PV costs 1.7 CHF/L.
Figure 7. (A) Production cost of the electricity (without grid cost per kWh without
The future cost is estimated based on the assumption that the CAPEX of components will drop to PV 200 CHF/kWp, Batteries 55 CHF/kWh, electrolyzer 500 CHF/kWel. with an efficiency of 90%, electronics 200 CHF/kW, DAC of CO2 200 CHF/kg and liquefaction of hydrogen 2000 CHF/kg. Therefore, the cost of hydrogen will decrease to 5 – 7 CHF/kg and synthetic oil will cost 2.3–2.7 CHF/kg. The main challenge for future cost reduction of hydrogen and synthetic fuels, beside the economy of scale, is the increase of the efficiency of electrolyzers. The cost of hydrogen and synthetic fuels is, therefore, expected to decrease to 50%–25% of the cost today.
The electricity demand of 60 TWh·y-1 in Switzerland (Swissgrid, 2024) corresponds in the annual average to a power of 6.8 GW, with a low of 6.5 ± 2 GW in summer and a high of 8.7 ± 1.5 GW in winter. If the additional electricity demand of 50 TWh·y-1 will be mainly produced by photovoltaics (>400 km2) a peak power of 90 GW is expected, leading to a day/night average power of 34 GW in summer, which is still much larger than the expected electricity demand of 12 GW. Therefore, the local battery storage is inevitable to bring the max. power down from 90 GW to 32 GW and cover the electricity demand during the night. In addition, the seasonal storage needs to absorb >20 GW during the summer months in order to store 20 TWh electricity for the winter.
The high efficiency of an electrified energy distribution is compromised by the requirement to produce at every moment exactly the electricity that is demanded. The resilience of an electric grid is very small compared to fuel-based distributed energy systems. Furthermore, the transport of electricity around the world is not an option for technical and geographical reasons unless a superconducting cable around the world will be realized one day. Local short-term storage in batteries is an option if the battery is cycled frequently, but very expensive for seasonal storage.
The conversion of electricity to hydrogen and further to ammonia or synthetic hydrocarbons (CH4 or oil), i.e., transforming electricity into a chemical energy carrier, opens other options for storage as compressed gas, cryogenic liquid, or liquid at ambient conditions. The conversion of the electricity into an energy carrier and back to electricity is limited by the technically realized efficiencies. The amount of energy carrier produced is determined by the production efficiency and the amount of energy recovered in the conversion back to electricity.
Hydroelectric plants with a storage lake exhibit a low electricity storage density of 1–4 kWh/m3 for 500 m and 1800 m of height difference and a roundtrip efficiency >70%. Furthermore, the size of the dam only depends on the hydrostatic pressure and not in the amount of water behind the dam. The volume of the stored water is determined by the shape of the valley. Removing soil and rocks from the valley to increase the volume is only possible for the construction of the dam due to the high energy demand (Bieniawski, 2024) of excavation of around 30 kWh/m3. In storage lakes with a large height difference of 1800 m the energy for excavation corresponds to less than 10 years for seasonal storage.
Alternatively, large underground caverns for pressurized gas or for liquified gas use the surrounding rock to hold the pressure (Wang et al., 2019) (max. 180 bar) or as a thermal insulator (thermal conductivity approximately 1–7 W m-1·K−1).
Figure 8 shows the conversion efficiency from electricity to energy carrier, storage, and back to electricity versus the storage density of electricity. Hydroelectric storage and batteries reach a roundtrip efficiency of more than 70%. However, the storage density is 10−3 TWh/Mm3 and <0.5 TWh/Mm3, respectively. The efficiency of heat storage depends on the temperature of the heat extracted from the storage and can reach 50% for the latent heat of the phase change of LiH at 700 °C and up to 30% for the sensible heat in sand at 600 °C. The efficiency of the chemical energy carriers (hydrogen (H2), ammonia (NH3), and synthetic hydrocarbons (CH4)) are at or below 20%. The electricity density of the chemical energy carriers shows three groups. The first one is located below the electricity density of 200 MWh·m-3 consisting of hydroelectricity, batteries and thermal storages as well as ammonia below 8 bar and hydrogen at 200 bar. Compressed hydrogen at 200 bar (H2 density 15 kg/m3 at 25°C) with 294 kWh/m3 less than the theoretical energy density of 400 kWh/m3 of Li-ion batteries but 3 times more than the realized energy density of 95 kWh/m3 in the Tesla Megapack2 (Wikipedia, 2023). The second group consists of liquid hydrogen (H2 density 70.8 kg/m3 at −252.87 °C and 1.013 bar), compressed methane at 200 bar (CH4 density 180 kg/m3 at 25 °C), liquid ammonia (NH3 density 682 kg/m3 at −33°C and 1.013 bar) and is 1.2–1.5 TWh/Mm3 and metal hydrides with 1 mass% of hydrogen and 67 kg H2·m-3 and 1.6 MWh·m-3. The third group represents the maximum storage density and consists of liquid methane (CH4 density 422.8 kg/m3 at −161.5°C and 1.013 bar) and liquid hydrocarbons (oil density 850 kg/m3 at 25 °C and 1.013 bar) and is around >3 TWh/Mm3.
Figure 8. Conversion efficiency from electricity to energy carrier, storage, and back to electricity versus the storage density of electricity in kWh·m-3 (1 MWh·m-3 = 1 TWh/ Mm-3). The efficiencies are based on technical efficiencies(
The production of hydrogen from renewable energy in regions with high solar intensity and available land area, e.g., Australia has several advantages: (1) The solar intensity is twice as high as in Switzerland, consequently size of the photovoltaic field is only half of what it would be in Switzerland. (2) Space for the PV fields is available and not difficult to reach. (3) The seasons in Australia (southern hemisphere) are opposite those in Europe. Consequently, the production and demand patterns match each other much better. (4) Global transport in liquid form by ship with propulsion of the ship with the boil-off is efficient and CO2 neutral. (5) Global trading of renewable energy carriers produces economic growth and benefits in regions where no economy currently exists. If Switzerland imports most of the renewable energy to produce 53 TWh of electricity in 6 - 8 thermal power plants with central district heating, 2.7 Mt y-1 (7,400 t d-1) of hydrogen has to be imported. In 2022, Switzerland imported 8.85 Mt y-1 of crude oil and oil products, and the mandatory reserves of oil and products are equivalent to 4.5 months, therefore, around 3 Mt.
Importing liquid hydrogen or liquid synthetic fuel (hydrocarbons) allows to produce electricity in combined cycle thermal power plants which consume a small space as compared to local PV fields. However, with the currently available technology and the cost of the components this approach leads to very high costs of the electricity produced from hydrogen and from synthetic fuel of >1 CHF/kWh and >2 CHF/kWh, respectively. In order to be competitive with the local PPU’s the liquid hydrogen and the synthetic fuel cost has to be <7 CHF·kg-1H2 and <1.75 CHF·L synfuel, respectively, and the electricity produced costs around 0.35 CHF·kWh-1.
Importing renewable liquid energy carriers and building the necessary storage for redundancy and reserves is mainly an economic challenge for Switzerland and requires that enough producers of renewable fuels worldwide will be available.
The production of synthetic hydrocarbons from renewable energy via PV is expensive because of the components: Electrolysis, CO2 capture and the Fischer-Tropsch synthesis. These three components determine 90% of the cost of the synthetic fuel. Today, the energy cost (PV electricity) contributes only <5% to the final cost of the synfuel. Bio mass with the general chemical composition of HCOH already contains the carbon as well as the hydrogen for a synthetic hydrocarbon. An additional hydrogen molecule is needed to reduce the oxygen in the compound. In case of palm oil, the energy in the palm oil is conserved in the hydriding process by adding 11wt% of hydrogen to remove the oxygen and to saturate the unsaturated carbon, leading to a mass loss of 15wt% and an increase of the energy content from 10.8 kWh·kg-1 to 12.8 kWh·kg-1. The synthetic fuel is similar to diesel or kerosene and can be stored in the already existing fuel storage tanks. Palm oil plantations are very efficient in harvesting solar energy and producing bio-oil. For 1 t y-1 of bio-oil an area of only 2,600 m2 is needed, close to the equator, approximately 5 times less area than for rapeseed oil (Rahman et al., 2024). In Switzerland, the reserves for redundancy of the power plant units and the fuel for aviation can be covered by imported bio-oil produced on approximately 7,000 km2 close to the equator and shipped to Switzerland with a rate of 2.4 Mt y-1.
The substitution of fossil fuels with renewable energy in Switzerland is possible if sufficient renewable energy is produced (+72 TWh·y-1) or renewable energy carriers are imported, and sufficient storage capacity (+20 TWh) is built up to provide electricity on demand. Six power plant units cover a large amount of the electricity demand, in addition to double the volume of the storage lakes of the hydroelectric power plants (+9 TWh·y-1), covering all feasible roof areas with photovoltaics (+21 TWh·y-1), and using a large fraction (20 TWh·y-1) of the available biomass for heating.
The most economic PPUs (Table 2) are the hydroelectric power plants (storage HYD-S and river HYD-R), the thermal power plants (THERM) using biogas, and the nuclear power plants (NUC). The construction of an additional hydroelectric power plant of the size of a PPU (HYD-S), i.e., 50% of all hydroelectric power plants with a storage lake existing in Switzerland, is very difficult to realize. Already, doubling the volumes of existing storage lakes is technically possible by increasing the height of the dam by 26% or by excavation of the lake, but this is a huge technical challenge. Adding a hydroelectric river plant PPU (HYD-R), i.e., 50% of all hydroelectric river plants existing in Switzerland, is technically impossible.
Table 2. Comparison of the parameters of the different PPUs, i.e., estimated resulting electricity cost per kWh, initial capital investment (CAPEX) for the PPU, the PV area, and the plantation area. The areas and CAPEX outside of Switzerland are indicated in parenthesis. Future developments of the renewable energy components may reduce the CAPEX more than 50%.
Construction of 6 + 2 thermal power plants or nuclear power plants is feasible. However, the new nuclear powerplants with a higher flexibility in the fuel supply and that are able to breed fuel are still in an early stage of development. China’s Ministry of Ecology and Environment has approved the commissioning of an experimental molten salt thorium nuclear reactor in Wuwei City, Gansu Province, China (Interestingengineering, 2024). The technology has the potential to contribute significantly to energy security in the future with a minimum of negative impacts on the environment. Furthermore, the breeding technology is able to reuse current nuclear waste and convert it to short-living isotopes and solve the problem of finding deep long-term storage cavities for nuclear waste.
Production of renewable electricity by photovoltaics is a feasible option for the future. The PV-field area, which is roughly twice the active PV-area, for one PPU corresponds approximately to the surface area of the lake of Zürich (100 km2). The advantage of the local production of renewable electricity is the high efficiency and low cost of the directly used electricity. The technical challenge is the seasonal storage of 25% of the produced electricity by a hydroelectric storage power plant.
Alternatively, hydrogen can be produced from PV electricity and stored. Due to the energy losses in conversion, the PV area needs to be large as for the hydroelectric storage, and the cost of electricity produced from hydrogen after storage increases significantly. Building large hydrogen storage units in Switzerland is feasible and necessary, also if the hydrogen would be produced outside of the country and imported. The necessary reserves are of a similar size as the seasonal storage, in the order of 20 TWh. The storage has to be built no matter where the hydrogen is produced. In addition, for redundancy and energy security, at least two more power plant units would have to be installed. Producing electricity from hydrogen in a combined cycle power plant (THERM) would deliver an equal amount of heat and electricity, the heat can be used for district heating in winter, cover most of the heating demand of Switzerland, and reduce the demand of local biomass for heating. The biomass is then available for synthetic fuels, i.e., biomass is pyrolyzed to syngas with hydrogen and converted to synthetic fuel and thus prevent capturing CO2 from a point source or even directly from air. The synthetic fuel is stored in the already installed oil storage tanks (mandatory reserves of 4.5 months). Furthermore, the synthetic fuel can be used for aviation as well as backup fuel for the thermal power plants. The cost of the synthetic fuel is estimated 4 CHF/L and, therefore, the electricity produced 1 CHF/kWh. This rather high cost is mitigated by the heat produced at the same time and by the mixture with lower cost electricity directly from PV and from the hydroelectric power plants resulting in an average cost of <0.33 CHF/kWh of energy.
As an alternative to the production of synthetic oil from biomass (wood) in Switzerland, bio-oil could be imported. The bio-oil has a high energy density (10.8 kWh/kg) and a synthetic oil similar to kerosene can be produced by a heterogeneously catalyzed hydriding reaction. The main advantage of bio-oil, especially palm oil, is its much lower cost compared to synthetic oil produced in Switzerland. Furthermore, the palm oil plant is efficient and requires 5 times less surface area compared to other bio-oils, e.g., oil from rapeseed. Switzerland could import up to 23 TWh·y-1 of bio-oil for aviation and hold a reserve of 20 TWh of bio-oil for the THERM PPUs, which are fueled either with hydrogen or bio-oil. Currently the synthetic bio-oil is estimated to cost around 1.7 CHF/L and, therefore, the electricity would cost around 0.35 CHF/kWh. The plantation area for Palm oil is about 5 times larger as compared to the field area of PV for the same amount of synthetic oil from hydrogen and CO2.
Table 3 shows an overview of the expected annual energy cost, the capital investment, the PV-area in Switzerland, and the area of the oil palm plantation outside of Switzerland for the different PPUs, as compared to the current (2019) energy economy. In any case, the energy reserves and the aviation fuel are assumed to come from imported bio-oil or bio-oil-based synthetic kerosene. The 6 PPUs would deliver 54 TWh·y-1 of electricity per year. This, combined with the 24 TWh·y-1 produced by photovoltaics on the roofs would provide 72 TWh·y-1 of renewable energy. However, a smart combination of PPUs, e.g., nuclear and combined cycle thermal plants including two thermal plants as reserves, allow to optimize the cost for energy. The use of the combined cycle thermal power plants as well as the nuclear power plants for central heating, further reduces the energy demand in winter below the demand estimated in this work (s. Figure 6B).
Table 3. Comparison of the parameters of the different PPUs, i.e., estimated resulting annual energy cost per capita, initial capital investment (CAPEX) for 6 PPU’s, the PV area, and the plantation area. The areas and CAPEX outside of Switzerland are indicated in parenthesis. The cost of doubling of the hydroelectric storage lake volume is not considered. Future developments of the renewable energy components may reduce the CAPEX more than 50%.
If we do not consider importing hydrogen or importing synthetic fuel until the cost decreases by a factor of 3 and 4, respectively, the annual cost for energy increases by a maximum of 54% to 42 BCHF. In 2022, Switzerland spent 12.4 BCHF for fossil energy carriers (Bazg, 2023) and approximately 27 BCHF in total for energy.
Energy security requires the availability of energy on demand at all times and in addition, reserves of at least 25% of the annual energy demand (>3 months). The future electricity demand in 2050 in Switzerland will increase to 80–130 TWh, depending on the development of the population and the future reduction of energy demand per capita due to improved efficiency and change of behavior. The analysis in the current work is based on an estimated electricity demand of 113 TWh in 2050. The replacement of nuclear power with renewable energy requires 3 PPUs. Depending on the development, 3 – 8 PPUs would cover the increase in electricity demand, and at least 2 PPUs are needed for reserve and energy security. District heating will make an important contribution to the increased energy demand in winter but is a challenge for redundancy since the heat also has to be produced on demand in a secure manner.
Trading of electricity across the border in Europe may still be economically interesting in the future, but this will not replace the reserves nor support energy security since all countries have the same seasons. Every country will have to build up their reserves. Storing energy in the form of synthetic oil is by far the easiest and most economical solution.
Expanding or reinforcing the grid does not replace storage. The local battery storage increases the cost of electricity production but reduces the maximum power. The peak power of PV in Switzerland is equal to close to 8 times the average power. Therefore, the local battery storage allows increasing the local consumption (night) without using the grid and reduces the maximum grid power by almost an order of magnitude (Cárdenas et al., 2021).
The annual solar irradiation determines the size of the photovoltaic field as well as the ratio of peak power to average power and, therefore, the size of the local battery storage and the power in the grid. Australia has twice the solar irradiation of Switzerland, plenty of abundant land area, and the seasons are shifted by half a year. A PV field of approximately 1′600 km2 (40 km × 40 km) in Australia is sufficient to deliver the energy for Switzerland. A PV field of 1.5 Mkm2 in Australia can replace all fossil fuels for the world with renewable energy. This is only about 20% of the land area of Australia or just slightly more than the Gobi Desert. More regions, e.g., in the Sahara or in North and South America, are available. In addition, a large wind power installation in Patagonia or tidal power in the Bristol channel (United Kingdom), yellow sea (Korea), and Penzias Bay (Russia) can contribute to the renewable energy production. Today, Africa produces 4 Mt y-1 of palm oil (Weforum, 2022), approximately twice as much as the Swiss aviation would consume. The global palm oil production has to increase significantly to make a very important contribution to the transformation from fossil to renewable energy. The price for palm oil on the world market is 720 CHF/t, that corresponds to 130 CHF per barrel (August 2023), and it has doubled in the last 20 years (Tradingeconomics, 2024). Palm oil plantations are biologically sustainable because they do not drain essential elements from the soil, are not toxic and highly efficient (385 t of palm oil per year on 1 km2) (WUR, 2024). It is a unique opportunity for the world’s development, because there are many regions that are abundant today and could be transformed in oil palm plantations creating an income for the local population and providing work and wealth especially in the less wealthy societies. Furthermore, the combination of the palm plantation with the upgrading of the palm oil with hydrogen will create a developing economy for the future. The price for the upgraded oil may be as high as 2.5 CHF/L and provide more benefit to the producer while it is still a factor of four cheaper than synthetic oil produced via Fischer-Tropsch synthesis from CO2 air capture and hydrogen from PV.
Finally, the oil plant plantations increase the amount of biomass on earth and bind a significant amount of CO2. If the current oil production of 90·106 barrels per day (4.4 Gt y-1) would be replaced with oil from the palm oil a plantation area of 12·109 km2 has to be created additionally to the current agricultural land of 44·109 km2. This is theoretically possible especially if some of the deserts are converted into palm oil plantations equipped with a solar sea water desalination.
The transition of Switzerland from fossil fuels to renewable energy involves an investment of approximately the energy cost of the coming 30 years. If we assume the energy cost of today (3′000 CHF·y-1 per capita), the investments are on the order of 50% of the annual GDP. In order to complete the transition in 27 years, Switzerland would have to invest 1.5% of the GDP every year. Such large investments over a period of 27 years are risky and difficult to realize. Currently, the costs for photovoltaic installations are covered by private persons and by industry, besides the subsidies of the government. As soon as the energy market is successfully transformed to renewable energy and stable, the free market will be able to take control. However, redundancy and stability are not provided by the free market because of the lack of benefits. The cost of nuclear power plants increased significantly in the last 20 years and is estimated today to range from 6 to 9 BCHF for a 1.1 GW plant (Schlissel and Biewald, 2008). With the technical improvement and the scale effects some of the components for the renewable energy conversion will decrease in cost which will lower the CAPEX as well as the cost of renewable electricity in future by approximately 50% as compared to today.
This paper addresses the question how renewable energy can substitute fossil energy and deliver energy on demand rather than on production on the example of Switzerland. A new concept of power plant units (PPUs) based on photovoltaic or wind is introduced. A PPU consists of the energy conversion and storage units and delivers a constant power of 1 GW, similar to a nuclear power plant. This makes a direct comparison of a renewable energy supply with a fossil energy supply possible. The capital cost (investment) and the levelized cost of energy are determined for the various PPUs. Furthermore, the amount of renewable energy is estimated and the substitution pathway is shown in order for Switzerland to become CO2 neutral in 2050.
The hydroelectric power plants with a storage lake, the river hydroelectric power plants, thermal combined cycle power plants (CCPP) and nuclear power plants are compared with photovoltaics (PV) and wind turbines (WT) with a local battery storage and either hydroelectric, hydrogen or synthetic hydrocarbon seasonal storage. Furthermore, import of hydrogen, synthetic hydrocarbon and bio oil for electricity production in CCPP were analyzed.
Beside the hydroelectric power plants and the nuclear power plants, the most economic and efficient PPU is based on PV or WT with a local battery storage capacity corresponding to >0.5% of the annual energy production in order to balance short term fluctuations in production and reduce the maximum power in the grid. The most efficient seasonal storage is pumped hydroelectricity, but the number of feasible hydroelectric power plants is limited. The local production of renewable electricity provides 75% of the electricity for a lower cost, only 25% are more expensive due to the seasonal storage. Importing energy carriers produced far away do not offer this opportunity and the electricity is always more expensive compared to local production.
The large potential for bio-oil production worldwide allows to substitute fossil oil with a CO2 neutral bio oil and to use the already existing transport, distribution and storage infrastructure. Furthermore, producing bio oil in regions, where currently no plants are growing, opens up new economic opportunities for less developed areas.
About 25% of the electricity on demand, mainly during winter time, is produced by CCPP providing also heat for district heating and hot water supply, which further reduces the electricity demand and energy cost.
The future ideal renewable energy economy is a compromise between the possible local production and storage and the more expensive import of CO2 neutral energy carriers, i.e., hydrogen or synthetic oil. Furthermore, the local production of renewable energy requires investments in building up the PV fields or WT and the short term and seasonal storage, while importing energy carriers only requires to build the CCPP. However, also imported energy carriers need to be stored just like the current mandatory storage for fossil oil, which corresponds to approximately 25% of the annual demand.
The energy cost per capita, which is currently around CHF 3000 per year remains almost the same if the electricity would be produced by nuclear power plants followed by PV in combination with hydroelectric seasonal storage increases the energy cost per capita by almost 50%. PV with seasonal storage with hydrogen, PV using the biomass for seasonal storage and importing Palm oil approximately double the energy cost per capita. Finally importing hydrogen or importing synthetic fuel increases the energy cost by more than a factor of 3.
The most economic energy system for Switzerland is installing PV and WT to cover 75% of the electricity demand in future and combine it with 6 + 2 CCPPs fueled with hydrogen or palm oil during the winter time and when reserves and redundancy is needed, corresponding to 25% of the electricity demand. This also provides a maximum flexibility and independency and allows to produce the required power at any time of the year. The aviation uses imported palm oil or with hydrogen upgraded palm oil to synthetic kerosene that can be produced today for less than 2 CHF/L.
Switzerland is in a favorable situation, because of the CO2 free electricity production. However, electrifying the energy economy and producing all the electricity from renewable energy is a technical and economic challenge, but feasible. On a global level, bio oil has the potential to enable the energy transition to a CO2 neutral and sustainable energy economy, allowing the seasonal (reserves) storage for a low cost and developing currently not used land areas.
The power plant units allow to compare renewable energy plants providing energy on demand rather than supply controlled. This approach can be applied to other countries or even on a global level in order to determine the technological challenges, the capital cost and the levelized cost of energy.
The original contributions presented in the study are included in the article/Supplementary Material, further inquiries can be directed to the corresponding author.
AZ: Conceptualization, Formal Analysis, Investigation, Methodology, Writing–original draft. CN: Investigation, Validation, Visualization, Writing–review and editing. LS: Resources, Validation, Visualization, Writing–review and editing. PG: Formal Analysis, Resources, Validation, Visualization, Writing–review and editing.
The author(s) declare that no financial support was received for the research, authorship, and/or publication of this article.
Critical review by Heinrich Fischer, Robert Lombardi, Lino Guzzella, René Bautz, Gilles Verdan is greatly acknowledged.
Author CN was employed by Christoph Nutzenadel AG.
The remaining authors declare that the research was conducted in the absence of any commercial or financial relationships that could be construed as a potential conflict of interest.
All claims expressed in this article are solely those of the authors and do not necessarily represent those of their affiliated organizations, or those of the publisher, the editors and the reviewers. Any product that may be evaluated in this article, or claim that may be made by its manufacturer, is not guaranteed or endorsed by the publisher.
The Supplementary Material for this article can be found online at: https://www.frontiersin.org/articles/10.3389/fenrg.2024.1336016/full#supplementary-material
Bafu (2024). Switzerland's long-term climate Strategy" from the federal Council. Available at: https://www.bafu.admin.ch/dam/bafu/en/dokumente/klima/fachinfo-daten/langfristige-klimastrategie-der-schweiz.pdf.download.pdf/Switzerland's%20Long-Term%20Climate%20Strategy.pdf.
Bauer, C., Hirschberg, S., Bauerle, Y., Biollaz, S., Calbry-Muzyka, A., Cox, B., et al. (2017) Potentials, costs and environmental assessment of electricity generation technologies. Villigen PSI, Switzerland: EPFL. Paul Scherrer Institut.
Bazg (2023). Bazg. Available at: https://www.bazg.admin.ch/bazg/de/home/aktuell/medieninformationen/medienmitteilungen/aussenhandelsstatistik.msg-id-92611.html.
Berichthaus (1912) Die Elektrifizierung der Schweizerischen Bahnen mit besonderer Berücksichtigung der ehemaligen Gotthardbahn. Germany: Berichthaus.
BFE (2024). BFE. Available at: https://www.bfe.admin.ch/bfe/de/home/versorgung/statistik-und-geodaten/energiestatistiken/teilstatistiken.html.
Bieniawski, R. Z. T. (2024). Specific energy of excavation in detecting tunnelling conditions ahead of TBMs. Available at: https://geocontrol.es/images/pdf/03EB-specific_energy.pdf.
Brethauer, S., and Hans-Peter Studer, M. (2021). Towards net zero greenhouse gas emissions in the energy and chemical sectors in Switzerland and beyond-a review. Chimia 75 (9), 788. doi:10.2533/chimia.2021.788
Burg, V., Bowman, G., Erni, M., Lemm, R., and Oliver, T. (2018). Analyzing the potential of domestic biomass resources for the energy transition in Switzerland. Biomass Bioenergy 111, 60–69. doi:10.1016/j.biombioe.2018.02.007
Campbell, C. J., and Laherrere, J. H. (1998). The end of cheap oil. Sci. Am. 278, 78–83. doi:10.1038/scientificamerican0398-78
Canadell, J. G., Le Quere, C., Raupach, M. R., Field, C. B., Buitenhuis, E. T., Ciais, P., et al. (2007). Contributions to accelerating atmospheric CO2 growth from economic activity, carbon intensity, and efficiency of natural sinks. PNAS 104 (47), 18866–18870. doi:10.1073/pnas.0702737104
Cárdenas, B., Swinfen-Styles, L., Rouse, J., and Garvey, S. D. (2021). Short-medium-and long-duration energy storage in a 100% renewable electricity grid: a UK case study. Energies 14 (24), 8524. doi:10.3390/en14248524
Changeoracle (2022). changeoracle. Available at: https://changeoracle.com/2022/07/20/nuclear-power-versus-renewable-energy/.
Colman, D. R., Poudela, S., Stamps, B. W., Boyda, E. S., and Spear, J. R. (2017). The deep, hot biosphere: twenty-five years of retrospection. PNAS 114 (27), 6895–6903. doi:10.1073/pnas.1701266114
Demirbaş, A. (1997). Calculation of higher heating values of biomass fuels. Fuel 76 (5), 431–434. doi:10.1016/s0016-2361(97)85520-2
Desing, H., and Widmer, R. (2022). How much energy storage can we afford? On the need for a sunflower society, aligning demand with renewable supply. Biophysical Econ. Sustain. 7, 3. doi:10.1007/s41247-022-00097-y
Empa (2024). EMPA. Available at: https://www.empa.ch/de/web/s604/energiezukunft.
Friedlingstein, P., Jones, M. W., O'Sullivan, M., Andrew, R. M., Bakker, D. C. E., Hauck, J., et al. (2022). Global carbon budget 2021. Earth Syst. Sci. Data 14, 1917–2005. doi:10.5194/essd-14-1917-2022
Interestingengineering (2024). Interestingengineering. Available at: https://interestingengineering.com/innovation/thorium-molten-salt-nuclear-reactor-china.
Jacobson, M. Z., von Krauland, A.-K., Coughlin, S. J., Dukas, E., Nelson, A. J. H., Palmer, F. C., et al. (2022). Low-cost solutions to global warming, air pollution, and energy insecurity for 145 countries. Energy Environ. Sci. 15, 3343–3359. doi:10.1039/d2ee00722c
Keith, D. W. (2009). Why capture CO2 from the atmosphere? Science 325 (5948), 1654–1655. doi:10.1126/science.1175680
Kotowicz, J., and Brzęczek, M. (2018). Analysis of increasing efficiency of modern combined cycle power plant: a case study. Energy 153 (15), 90–99. doi:10.1016/j.energy.2018.04.030
Lutz, W., Sanderson, W., and Scherbov, S. (2001). The end of world population growth. Nature 412, 543–545. doi:10.1038/35087589
Mo, L., Zohner, C. M., Reich, P. B., Liang, J., de Miguel, S., Nabuurs, G. J., et al. (2023). Integrated global assessment of the natural forest carbon potential. Nature 624, 92–101. doi:10.1038/s41586-023-06723-z
Murphy, D. J., Goggin, K., and Paterson, R. R. M. (2021). Oil palm in the 2020s and beyond: challenges and solutions. CABI Agric. Biosci. 2, 39. doi:10.1186/s43170-021-00058-3
Osman, A. I., Mehta, N., Elgarahy, A. M., Hinai, A.Al., Al-Muhtaseb, A. ’a H., and Rooney, D. W. (2021). Conversion of biomass to biofuels and life cycle assessment: a review. Environ. Chem. Lett. 19, 4075–4118. doi:10.1007/s10311-021-01273-0
Osman, M. F. A. I., Chen, Z., Abdelhaleem, A., Ihara, I., Mohamed, I. M. A., Seng Yap, P., et al. (2023). Social, environmental, and economic consequences of integrating renewable energies in the electricity sector: a review. Environ. Chem. Lett. 21, 1381–1418. doi:10.1007/s10311-023-01587-1
Ourworldindata (2024). ourworldindata. Available at: https://ourworldindata.org/grapher/energy-use-per-capita-vs-gdp-per-capita.
Panos, E., Kannan, R., Hirschberg, S., and Kober, T. (2023). An assessment of energy system transformation pathways to achieve net-zero carbon dioxide emissions in Switzerland. Earth Environ. 4 (1), 157. doi:10.1038/s43247-023-00813-6
PSI (2023). Final-report-BFE-project. Available at: https://www.psi.ch/sites/default/files/import/ta/PublicationTab/Final-Report-BFE-Project.pdf.
Pulingam, T., Lakshmanan, M., Chuah, J.-A., Surendran, A., Zainab-La, I., Foroozandeh, P., et al. (2022). Oil palm trunk waste: environmental impacts and management strategies. Industrial Crops Prod. 189, 115827. doi:10.1016/j.indcrop.2022.115827
Rahman, M. M., Ahiduzzaman, M., Sadrul Islam, A. K. M., and Blanchard, R. (2024). Karoch Pongamia pinnata- an alternaKve source of biofuel in Bangladesh. Available at: https://www.researchgate.net/publicaKon/355735059.
Ruhnau, O., and Qvist, S. (2022). Storage requirements in a 100% renewable electricity system: extreme events and inter-annual variability. Environ. Res. Lett. 17, 044018. doi:10.1088/1748-9326/ac4dc8
Rudisuli, M., Teske, S. L., and Elber, U. (2019). Impacts of an increased substitution of fossil energy carriers with electricity-based technologies on the Swiss electricity system. Energies. doi:10.3390/en12122399
Sarmiento, J. L., and Gruber, N. (2002). SINKS FOR ANTHROPOGENIC CARBON. Phys. TODAY 55, 30–36. doi:10.1063/1.1510279
Schlissel, D., and Biewald, B. (2008). Nuclear power plant construction costs. Available at: www.synapse-energy.com.
Sheng, C., and Azevedo, J. L. T. (2005). Estimating the higher heating value of biomass fuels from basic analysis data. Biomass Bioenergy 28 (5), 499–507. doi:10.1016/j.biombioe.2004.11.008
Sinap (2023). Sinap. Available at: https://www.sinap.cas.cn/xwzx/tzgg/202212/P020221207808949039651.pdf.
Sustainablepalmoilchoice (2024). Sustainablepalmoilchoice. Available at: https://www.sustainablepalmoilchoice.eu/more-facts/#:∼:text=The%20average%20yield%20of%20oil,1%20ton%20of%20palm%20oil.
Swissgrid (2024). Swissgrid. Available at: https://www.swissgrid.ch/de/home/operation/grid-data/load.html#vertikale-netzlast.
Swissolar (2024). Swissolar. Available at: https://www.swissolar.ch/de/angebot/news-und-medien/fakten-und-zahlen/infografiken.
Thomas, T. (1992). The deep, hot biosphere. Proc. Natl. Acad. Sci. U. S. A. 89, 6045–6049. doi:10.1073/pnas.89.13.6045
Tradingeconomics (2024). Tradingeconomics. Available at: https://tradingeconomics.com/commodity/palm-oil.
VSE/AES (2024). Energiezukunft 2050. Available at: https://www.google.com/url?sa=t&rct=j&q=&esrc=s&source=web&cd=&ved=2ahUKEwiXjfuoh6L-AhV0i_0HHZzwD9sQFnoECAwQAQ&url=https%3A%2F%2Fwww.strom.ch%2Fde%2Fmedia%2F13957%2Fdownload&usg=AOvVaw0Lb3anrIngE5FnLYdCEE6X(11.4.2023.
Walcha, A., Castello, R., Mohajeri, N., and Scartezzini, J.-L. (2020). Big data mining for the estimation of hourly rooftop photovoltaic potential and its uncertainty. Appl. Energy 262, 114404. doi:10.1016/j.apenergy.2019.114404
Wang, T., Li, J., Jing, G., Zhang, Q., Yang, C., and Daemen, J. J. K. (2019). Determination of the maximum allowable gas pressure for an underground gas storage salt cavern: a case study of Jintan, China. J. Rock Mech. Geotechnical Eng. 11, 251–262. doi:10.1016/j.jrmge.2018.10.004
Way, R., Ives, M. C., Mealy, P., and Doyne Farmer, J. (2024). Empirically grounded technology forecasts and the energy transition. Joule 6, 2057–2082. doi:10.1016/j.joule.2022.08.009
Weforum (2022). Weform. Available at: https://www.weforum.org/agenda/2022/11/how-african-palm-oil-can-boost-livelihoods-and-protects-forests/.
Wikipedia (2023). Tesla. Available at: https://en.wikipedia.org/wiki/Tesla_Megapack.
Wir (2022). Wir. Available at: https://wir2022.wid.world/insights/.
Woodhead Publishing (2017) Molten salt reactors and thorium energy. Ansari Road, Darya Ganj: Woodhead Publishing.
WUR (2024). WUR. Available at: https://www.wur.nl/en/newsarticle/new-light-on-the-sustainability-of-palm-oil.htm.
Xiang, Li, Damartzis, T., Stadler, Z., Moret, S., Meier, B., Friedl, M., et al. (2020). Decarbonization in complex energy systems: a study on the feasibility of carbon neutrality for Switzerland in 2050. Front. Energy Res. 8, 549615. doi:10.3389/fenrg.2020.549615
Yuan, Xi, Su, C.-W., Umar, M., Shao, X., and Lobont, O.-R. (2022). The race to zero emissions: can renewable energy be the path to carbon neutrality? J. Environ. Manag. 308, 114648. doi:10.1016/j.jenvman.2022.114648
Züttel, A., Gallandat, N., Dyson, P. J., Schlapbach, L., Gilgen, P. W., and Shin-Ichi, ORIMO (2022). Future Swiss Energy Economy: the challenge of storing renewable energy. Front. Energy Res. Process Energy Syst. Eng. 9. doi:10.3389/fenrg.2021.785908
Keywords: renewable energy, energy storage, cost of energy, power plant units, CO2 free, nuclear
Citation: Züttel A, Nützenadel C, Schlapbach L and Gilgen PW (2024) Power plant units for CO2 neutral energy security in Switzerland. Front. Energy Res. 12:1336016. doi: 10.3389/fenrg.2024.1336016
Received: 09 November 2023; Accepted: 22 May 2024;
Published: 02 August 2024.
Edited by:
Pourya Pourhejazy, UiT The Arctic University of Norway, NorwayReviewed by:
Ghobad Bagheri, Technip Energies, NetherlandsCopyright © 2024 Züttel, Nützenadel, Schlapbach and Gilgen. This is an open-access article distributed under the terms of the Creative Commons Attribution License (CC BY). The use, distribution or reproduction in other forums is permitted, provided the original author(s) and the copyright owner(s) are credited and that the original publication in this journal is cited, in accordance with accepted academic practice. No use, distribution or reproduction is permitted which does not comply with these terms.
*Correspondence: Andreas Züttel, YW5kcmVhcy56dWV0dGVsQGVwZmwuY2g=
Disclaimer: All claims expressed in this article are solely those of the authors and do not necessarily represent those of their affiliated organizations, or those of the publisher, the editors and the reviewers. Any product that may be evaluated in this article or claim that may be made by its manufacturer is not guaranteed or endorsed by the publisher.
Research integrity at Frontiers
Learn more about the work of our research integrity team to safeguard the quality of each article we publish.