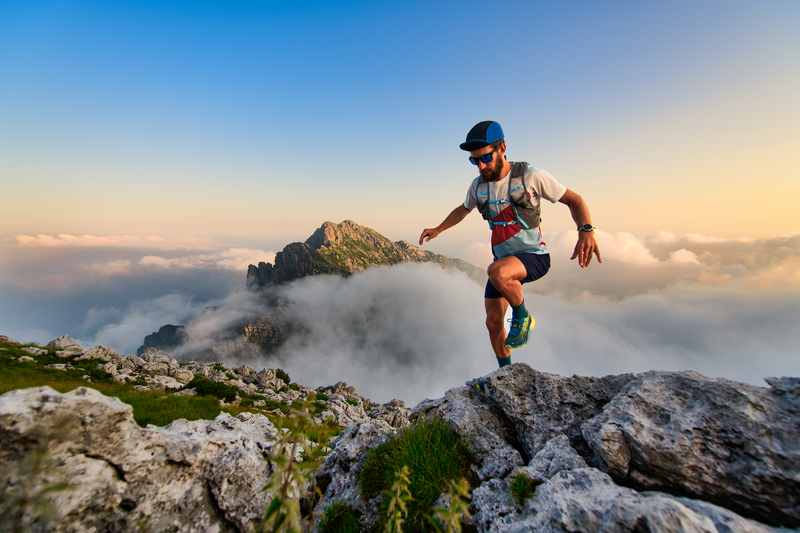
95% of researchers rate our articles as excellent or good
Learn more about the work of our research integrity team to safeguard the quality of each article we publish.
Find out more
MINI REVIEW article
Front. Energy Res. , 15 December 2023
Sec. Electrochemical Energy Storage
Volume 11 - 2023 | https://doi.org/10.3389/fenrg.2023.1325316
This article is part of the Research Topic High-Performance Lithium-metal Batteries View all 4 articles
As the specific energy of traditional lithium-ion batteries (LIBs) approaches theoretical limits, the quest for alternatives intensifies. Lithium metal batteries (LMBs) stand out as a potential solution, promising substantially higher energy densities (∼35% increase in specific energy and ∼50% increase in energy density at the cell level). Historically, challenges with liquid electrolytes (LEs) in LMBs, such as dendrite growth and unstable solid electrolyte interphase (SEI) formation, led to skepticism about their compatibility and most of the focus was on solid-state electrolytes (SSEs) such as polymer electrolytes and recently inorganic electrolytes (oxides, sulphides halides). However, recent strides in LE engineering have repositioned LEs as viable candidates for LMBs, particularly with the strategic use of additives and the careful formulating of solvents. This review delves into the engineering of LEs for LMBs, highlighting their renewed potential and explores the realm of SSEs and report on the recent advancements in both fields. We aim to provide a comprehensive overview of the evolving landscape of LMB research.
Lithium-ion batteries (LIBs) have revolutionized the realms of portable electronics and electric vehicles, offering transformative power storage solutions for a technologically-driven society (Chu et al., 2016). Their widespread adoption was driven by the high energy density as well as efficiency and versatility they introduced, which was notably absent in the battery technologies that preceded them (Liu et al., 2019a). Yet, the insatiable demand for even higher energy density and efficiency reveals that the potential of LIBs is nearing its limit, especially with graphite-based anodes reaching their theoretical energy threshold (Lin et al., 2017). Lithium metal batteries (LMBs) emerged as a promising alternative, offering much higher specific capacities (Liu et al., 2017). They were, in fact, commercialized even before LIBs but were shelved due to notable safety concerns and limited cyclability (Lin et al., 2017). Their potential to usher in an era of batteries with unparalleled specific energy has been acknowledged, given their impressive specific capacity and low reduction potential (Liu et al., 2017). By replacing the graphite electrode with lithium metal, there’s a projected ∼35% increase in specific energy and ∼50% increase in energy density at the cell level, outlining a promising route to meet the US Department of Energy’s (DOE) electric vehicle pack benchmarks of 235 Wh kg–1, 500 Wh L–1, and a cost of 125 US$ kWh–1 (Albertus et al., 2017). However, despite these compelling advantages, LMBs face significant challenges, such as the growth of dendrites, which not only shorten battery life but also pose serious safety threats (Albertus et al., 2017).
A crucial aspect of lithium batteries, both LIBs and LMBs, is the choice and stability of the electrolyte used. Historically, ether-based electrolytes dominated the landscape due to their reductive stability (Peled, 1979). However, with the advent of high-voltage cathodes, there was a noticeable shift. The changing dynamics required a shift from ether solvents to carbonate-based, moderate-concentration electrolytes to ensure compatibility (Amanchukwu et al., 2020). Although these aligned with high-voltage cathodes, they exhibited instability when paired with lithium metal, leading to issues like dendritic growth and reduced cycle life (Qian et al., 2015).
Considering these developments and challenges, the past decade saw researchers pioneering strategies that enhanced electrolyte concentrations and opened doors to more stable electrolytes that could work seamlessly with lithium metal anodes and high-voltage cathodes. However, the quest for the ideal LMB is ongoing, as the performance of current LMBs still falls short of practical application benchmarks (Louli et al., 2020). Figure 1A demonstrates the increased interest in LMBs in recent literature by showcasing the ratio of publications containing the keyword ‘lithium metal batteries’ to publications containing the more general keyword ‘lithium batteries’ calculated from numbers provided by Manthiram et al. (Su and Manthiram, 2022). This mini review will delve deeper into the forefront of battery research. Firstly, we will discuss recent advances in lithium electrolyte (LE) engineering, shedding light on the innovations that have enhanced battery performance and safety. Subsequently, we will explore the strides made in enabling solid-state lithium metal batteries (SSLMBs). Figure 1C, D show schematics that summarize the challenges within LE enabled LMBs and solid-state electrolytes (SSEs) enabled LMBs. Finally, we gaze into the horizon of electrochemical energy storage, outlining our envisioned future targets and practical considerations for LMBs. We will explore pathways, informed by current research trends and technological gaps, on how we might traverse the journey from present achievements to future aspirations.
FIGURE 1. (A) Ratio of publications with the keyword lithium metal battery to publications with the keyword lithium battery adapted from (Su and Manthiram, 2022). (B) Comparison of capacity retention curves of batteries with different Coulombic efficiencies. (C) Schematics comparing condition of lithium metal batteries cycled with non compatible liquid electrolyte (top) and compatible liquid electrolyte (bottom) reproduced from (Wang et al., 2022). (D) potential challenges in all-solid-state lithium metal batteries reproduced from (Liu et al., 2022).
The pursuit of enhancing electrolyte concentrations for superior battery performance can be traced back to LIB research from the mid-1980s (Yamada and Yamada, 2015a; Wang et al., 2022). McKinnon and Dahn’s 1985 discovery showcased how saturated LiAsF6 in propylene carbonate (PC) successfully circumvented the co-intercalation of PC into ZrS2 electrodes (McKinnon and Dahn, 1985). Subsequent works by Jeong et al. extended these findings, highlighting the efficacy of concentrated solvents like LiClO4, LiPF6, and LiBETI in PC to suppress co-intercalation into graphite anodes. (Jeong et al., 2003; Jeong et al., 2008a). Recently, Yamada et al. successfully extended their application to alternative solvents such as dimethyl sulfoxide (DMSO) and acetonitrile (AN), complemented with 3.2–4 M LiTFSI as the salt (Yamada et al., 2010). These high concentration electrolytes (HCEs) not only curtailed co-intercalation, but also significantly enhanced the electrolyte’s reductive stability (Yamada et al., 2014). Additionally, Suo et al., ‘s 2015 adaptation of this approach to water-based electrolytes marked a pioneering venture into aqueous LIB chemistry (Suo et al., 2015).
HCE’s potential in enhancing Li metal cycling came to the forefront with Jeong et al., ‘s 2008 exploration, which, while promising, highlighted the necessity for further optimization (Jeong et al., 2008b). A groundbreaking study in 2015 by Qian et al. detailed the success of 4 M LiFSI in DME, achieving impressive Li cycling columbic efficiency (CEs): 99.1% under 0.2 mA/cm2 and 98.4% under 4 mA/cm2 (Qian et al., 2015). Numerous subsequent investigations have further refined HCEs for LMBs, striving for optimal performance and stability (Zeng et al., 2018a; Fan et al., 2018b; Suo et al., 2018; Hagos et al., 2019; Maeyoshi et al., 2019; Chen et al., 2020; Wang et al., 2022). However, despite their merits, HCEs do possess shortcomings, including reduced ionic conductivity and increased viscosity, which necessitate specialized separators (Yamada and Yamada, 2015b). Moreover, the high concentration leads to substantial cost implications due to the predominant usage of expensive Li salts (Zhang et al., 2020).
To circumvent HCEs’ limitations, diluting HCEs with another solvent seemed to be a straightforward solution. However, the challenge lay in selecting a solvent that would not compromise the Li-ion solvation environment intrinsic to HCEs. Early work by Dokko et al., in 2013 highlighted this potential, leveraging the benefits of diluting HCEs with solvents like 1,1,2,2-tetrafluoroethyl-2,2,3,3-tetrafluoropropyl ether (TTE) to enhance Li-S batteries’ cyclability (Dokko et al., 2013). A slew of subsequent research has expanded on these findings, identifying optimal solvent ratios and candidates for dilution that ensure stability and performance (Moon et al., 2015; Ueno et al., 2016; Doi et al., 2017; Wang et al., 2022).
Chen and co-workers’ 2018 introduction of a Li metal CE of 99.3% in a unique electrolyte comprising 5.5 M LiFSI/dimethyl carbonate and bis(2,2,2-trifluoroethyl)ether highlighted the innovation in LHCEs (Chen et al., 2018c). A series of LHCEs followed, each honing in on the optimal molar ratios and solvents to maximize LMB performance (Chen et al., 2018d; Cao et al., 2019; Fan et al., 2019; Ren et al., 2019; Wang et al., 2022). The full potential of these electrolytes has yet to be validated.
Electrolyte additives have etched a reputation for significantly impacting Li-based battery performance (Xu, 2004; Zhang et al., 2018c). Historical research has presented a plethora of additives, from 2-methylfuran (Abraham et al., 1984) and CO2 (Aurbach et al., 1992; Aurbach and Zaban, 1994) to a wide range of inorganic metal ions (Matsuda, 1993; Yoon et al., 2008; Vega et al., 2009; Stark et al., 2011; Wang et al., 2022). Modern advancements, particularly over the past half-decade, have witnessed an influx of new organic/inorganic additives (Wang et al., 2022). Some notable mentions include solvents like vinylene carbonate, (Stark et al., 2011; Ren et al., 2018), fluoroethylene carbonate, (Stark et al., 2011; Zhang et al., 2017), and bis(2,2,2-trifluoroethyl) carbonate (Louli et al., 2019) salts like LiAsF6, (Ren et al., 2018), LiPF6, (Stark et al., 2011), and LiNO3, (Li et al., 2015; Zhang et al., 2018; Yan et al., 2018; Wang et al., 2019; Yan et al., 2019; Wang et al., 2022), and innovative materials such as LiF, (Lu et al., 2014), nanodiamonds, (Cheng et al., 2017), LiPS, (Li et al., 2015), and tris(pentafluorophenyl) borane (Li et al., 2020). Among these, fluorinated chemicals, especially LiF, FEC, and CuF2, have proven to be particularly impactful. LiNO3’s widespread adoption further underscores its effectiveness, greatly enhancing Li metal’s CE (Wang et al., 2019). In tandem, the integration of additives with electrolytes, as demonstrated by Lu and co-workers, (Li et al., 2020; Zhang et al., 2020), showcases the vast potential inherent in additive candidates. These combinations and synergies underline the promise additives hold for enhancing LMBs’ performance.
Fluorinated electrolytes have emerged as a promising electrolyte due to their ability to form stable solid electrolyte interphase (SEI) or cathode electrolyte interphase (CEI) layers (Yuan et al., 2023). These electrolytes are derived by substituting hydrogen atoms in solvent molecules with fluorine atoms, which aids in forming LiF-rich SEI or CEI layers on both the cathode and Li metal (Li et al., 2019). This substitution also reduces the flammability of organic solvents (Shadike et al., 2021; Xu et al., 2021). Wang et al. introduced an all-fluorinated electrolyte composed of 1 M LiPF6 in a mixture of FEC, 3,3,3-fluoroethyl-methyl carbonate, and 1,1,2,2-tetrafluoroethyl-2′,2′,2′-trifluoroethyl ether. This electrolyte is nonflammable and forms F-rich SEI layers (Shadike et al., 2021). With added lithium difluorooxalatoborate salt, the interphase of the LiNiO2 cathode is stabilized, enhancing electrochemical performance (Deng et al., 2019). It is also suitable for 5.3 V high-voltage cathodes by forming LiF-rich CEI layers (Chen et al., 2019). Yu et al. designed fluorine-substituted ether solvents, which after fluorine substitution, became highly stable against both Li-metal and high-voltage cathodes (Yu et al., 2020). They further synthesized fluorinated-1,2-diethoxyethanes, revealing that the position and amount of F atoms on 1,2-diethoxyethane significantly influence electrolyte performance (Kim et al., 2022). Fluorinated electrolytes also exhibit extended electrochemical windows, reaching up to 6 V, surpassing conventional carbonate electrolytes (Yang et al., 2021). However, they have higher viscosity and lower ionic conductivity, necessitating the use of co-solvents to optimize these properties.
Phosphate solvents, inherently non-flammable, have been explored as potential electrolyte solvents as well. These solvents, while employed in various applications as flame-retardant additives, unfortunately, exhibit instability towards the Li-metal anode. For instance, Wang et al. highlighted that even with an electrolyte containing 10 wt% tris(2,2,2-trifluoroethyl) phosphite additive, Li–S batteries failed after 700 cycles due to the Li-metal anode’s degradation (Wang et al., 2014). Liu et al. delved into the stability of Li deposition in phosphate electrolytes with varying salt-to-solvent ratios. They found that a 1:1 ratio was essential to achieve an initial CE of 94.8% for Li deposition (Zeng et al., 2018b). Several strategies have been proposed to stabilize the Solid Electrolyte Interphase (SEI) between the Li-metal anode and phosphate solvents. These include designing ion-solvation phosphate-based electrolytes, preparing LHCEs, constructing nitride interphases, and developing inorganic-rich solid-state electrolytes (Shi et al., 2018). In high-concentration phosphate electrolytes, both the phosphate solvent and Li ions are surrounded by lithium salt anions. This configuration leads to the formation of inorganic-rich SEI layers during discharge, enhancing Li deposition’s average CE to over 99% when the salt-to-solvent ratio is 1:2 (Zeng et al., 2018a). Additives have also shown promise in stabilizing the interphase in phosphate electrolytes. Guo et al. introduced LiNO3 into the triethyl phosphate electrolyte, resulting in a nitride-rich interphase that improved the reversibility of Li deposition (Tan et al., 2019). Another effective additive, Li2O, has been shown to enhance the inorganic content in the SEI layer, improving its stability (Zhang et al., 2021; Kim et al., 2022).
Ionic liquids (IL), by virtue of their distinct physicochemical properties, have marked a significant advance in the electrolyte landscape for LMBs Renowned for their nonflammability and negligible volatility, these liquids are poised to replace conventional carbonate-based solvents, enhancing the safety metrics of battery systems (Sun et al., 2020; Dong et al., 2022). The ionic liquids featuring FSI-/TFSI- anions have garnered attention due to their efficient ion transfer, with the reduced interaction between cations and anions boosting Li+ fluidity (Wang et al., 2019). One such prominent example is the EM–5Li–Na IL electrolyte, composed of 1-ethyl-3-methylimidazolium bis(fluorosulfonyl)imide ([EMIm]FSI) mixed with 5 M lithium bis(fluorosulfonyl)imide (LiFSI) and 0.16 M sodium bis(trifluoromethanesulfonyl)imide (NaTFSI) additive. This electrolyte showcases a low viscosity of 125 mPa s at ambient temperature, significantly lower than earlier ionic liquid formulations (Sun et al., 2020). Its ion conductivity stands at ∼2.6 mS cm-1 at 25°C, appreciably higher than other ionic liquids used in LMBs, and increases further with temperature (Sun et al., 2020). The LiCoO2 (LCO)||Li cell employing this electrolyte demonstrates superior capacity retention and CE at moderate rates, in contrast to the rapid performance degradation seen with conventional electrolytes (Sun et al., 2020). Furthermore, the high concentration of LiFSI salts in the EM–5Li–Na IL electrolyte is linked to its enhanced battery performance, and the strategic inclusion of NaTFSI as an additive is a novel approach to LMB improvement (Sun et al., 2020). In essence, ionic-liquid electrolytes, with their broad electrochemical windows and superior safety profiles, could also offer a potential path forward for the development of high-voltage, high-safety LMBs.
Solid-state electrolytes (SSEs) are also a promising option as an electrolyte in LMBs. Monroe’s report highlighted that a solid electrolyte with a shear modulus greater than 6.8 GPa effectively suppresses lithium dendrites (Monroe and Newman, 2005). It was also established that, aside from the modulus, other properties like Li+ conductivity of the solid electrolyte have a role in dendrite suppression (Khurana et al., 2014). Optimal solid electrolytes share certain desired characteristics, (Wang et al., 2021), namely,.
• High Li+ ion conductivity at ambient temperature.
• Adequate mechanical strength to prevent dendrite growth.
• Compatibility at the interface with both electrodes.
• Chemical and electrochemical stability.
Among the various solid electrolytes researched, they can be broadly classified into ceramic electrolytes, polymer electrolytes, and hybrid inorganic/polymer solid electrolytes.
These are notable for their good Li+ conductivity (>10–3 Scm−1) and high elastic modulus, ranging from tens to hundreds of gigapascals (Xiong et al., 2020). Oxides (Li et al., 2019; Guo et al., 2019; Jiang et al., 2020) and sulfides (Zhao et al., 2020) have been explored as potential electrolytes in LMBs. However, their intrinsic brittleness and rigidity, combined with compatibility issues at the solid electrolyte/lithium interface, pose significant challenges for real-world applications. Interlayers between the solid electrolyte and lithium, like a membrane consisting of lithium perchlorate, poly (ethylene oxide) (PEO), and garnet particles, have been introduced to mitigate these problems (Pervez et al., 2019; Wen et al., 2019).
Comprising of a blend of lithium salts and a polymer matrix, polymer solid electrolytes benefit from enhanced interface compatibility over their inorganic counterparts, thanks to their inherent flexibility (Li et al., 2019). PEO stands out as the primary material in this category. However, challenges arise due to its low modulus (below 400 MPa) and insufficient ion conductivity at ambient temperature (Balo et al., 2018; Ding et al., 2019). Strategies to enhance Li+ transport dynamics include the introduction of plasticizers such as IL, (Balo et al., 2018), incorporation of inorganic fillers, (Zhao et al., 2020), and fabricating cross-linked polymer electrolytes (Khurana et al., 2014). Recent innovations in this space include ILE@MOF electrolyte, which offers enhanced ionic conductivity by anchoring anions of the electrolyte salt (Chen et al., 2019).
Merging the benefits of both inorganic and polymer solid electrolytes, this category has garnered significant attention. Various polymer matrices (like PEO, (Li et al., 2020), polyethylene glycol (PEG), (Pan et al., 2020), and polycaprolactone (Zhang et al., 2019)) and inorganic fillers (e.g., lithium aluminium germanium phosphate (Zhang et al., 2019), lithium germanium phosphorus sulfide (Liu et al., 2019b; Pan et al., 2020), and lithium lanthanum zirconium oxide (Li et al., 2019)) have been combined to strike a balance between flexibility, conductivity, and rigidity. For instance, an LAGP-PEO hybrid solid electrolyte demonstrated commendable stability attributed to its impressive Li + conductivity and flexural modulus, reinforced by LAGP particles in the PEO matrix (Li et al., 2020).
Despite the advancements in solid electrolyte technologies, there remain substantial challenges. The interfacial resistances documented in several studies are notably high, resulting in relatively low current densities when used in batteries (Al-Salih et al., 2022; Al-Salih et al., 2024). One of the paramount issues in solid-state lithium batteries is the uncontrollable dendrite growth at high current densities, which can result in short circuits or diminished capacity. Dendrite formation might be influenced by the nonuniform contact between the solid electrolyte and lithium metal, but the underlying mechanisms in solid-state batteries remain underexplored (Tsai et al., 2016). Some studies, like that by Aguesse et al., have shed light on potential reasons, such as lithium clusters in cavities and pores of the garnet solid electrolyte or lithium metal accumulation in the pores leading to electrolyte failure (Aguesse et al., 2017). Yet, a comprehensive understanding of dendrite formation in solid electrolytes is still elusive. Considering the rapid advancements and evident challenges of SSEs, continuous research is crucial. While we’ve made progress, the onus is on the scientific community to further unravel the intricacies of solid-state lithium batteries, ensuring that we harness their full potential in the evolving landscape of energy storage solutions.
Table 1 lists examples of impressive high performing lithium metal batteries reported in the literature in the past few years.
TABLE 1. Recently reported lithium metal batteries employing novel liquid and solid electrolytes along with their reported performance. Note: N/P is the ratio of negative to positive electrode capacity.
Drawing upon the extensive analysis of practical considerations for LMB electrolytes, several aspects emerge that warrant critical discussion that takes into account industry, consumer, and market perspectives. The future of LMB technology is undoubtedly reliant on the interplay between battery materials’ chemistry, their structural/performance relationship and their validation in industrial cells under real life application conditions. Let’s delve into some of these aspects more deeply.
The commercial feasibility of LMBs is inextricably linked to the cost-effectiveness of the electrolyte. However, emphasizing cost-effectiveness should not be at the expense of environmental considerations. In fact, the integration of green chemistry and atom economy must be central to future endeavors. Leveraging techniques such as organic-solvent-free methods for salt synthesis, like ball milling, or employing organic synthetic procedures that yield fewer byproducts can help bridge the gap between commercial viability and eco-responsibility.
The spotlight on the cycle life of LMB electrolytes has inadvertently dimmed the attention towards the calendar life of these batteries. Even though recent electrolytes have made strides in cycling efficiency, there is still much ground to cover in terms of calendar life, with factors like SEI thickening playing a dominant role in capacity loss. Drawing parallels with the solid electrolytes for all-solid-state lithium batteries (ASSLBs), it becomes evident that while much progress has been made, the understanding of the nuanced mechanisms at play, like ion solvation and SEI formation pathways, is still in its infancy. The promise of anode-free LMBs heralds a transformative phase in energy density optimization. However, this promise is deeply intertwined with achieving exceptionally high CE. If a future electric vehicle (EV) is designed to journey 1,000 km per charge cycle, it necessitates a CE of at least 99.95% (see Figure 1B). This would ensure the battery’s capability to power the vehicle for a staggering 400,000 km, harmonizing with the typical lifespan of many other car components. Such a CE threshold, while ambitious, positions the battery pack as a beacon of durability, potentially outpacing most other vehicle parts in longevity. For the auto industry, this paints a future where the battery is not the limiting factor but a benchmark of resilience and reliability, setting a realistic yet pioneering goal for future EVs.
Just as the interfacial resistance in solid-state batteries (SSBs) is a challenge, safety stands out as a paramount concern for LMBs. While non-flammable solvents or additives show promise, their true safety merits can only be adjudicated under realistic conditions, such as nailing or heating tests. This necessity is highlighted by Ouyang et al.'s findings, which suggest that even assumed non-flammable electrolytes like trimethyl phosphate-based HCE can combust under the most rigorous conditions.
The ability of LMBs to function efficiently across a broad temperature spectrum is a pertinent challenge that mirrors the need for SSEs to maintain stable contact with electrodes across varying conditions. Whether it is high-temperature performance, contingent on the robustness of SEI and CEI, or low-temperature operation, reliant on innovative solutions such as liquified gas electrolytes or weakly solvating solvents, both spectrums necessitate rigorous research and innovation.
To conclude, the realization of optimized LMBs requires a confluence of materials chemistry, engineering, safety protocols, and an understanding of real-world operational challenges. The path forward necessitates researchers from academia, government labs, and industry alike work hand-in-hand to prioritize cost-effectiveness, eco-friendliness, safety, and operational versatility to meet the demands of a dynamic marketplace. Embracing novel chemistries, for the components of the liquid electrolytes and solid electrolyte, at the vanguard of innovation. As we continue to expand our knowledge and develop more sophisticated strategies, the promise of LMBs as an advanced energy storage solution becomes increasingly tangible, paving the way for their integration into the sustainable energy storage landscape.
HA-S: Conceptualization, Investigation, Software, Writing–original draft, Writing–review and editing. HK: Data curation, Formal Analysis, Writing–review and editing. EB: Project administration, Resources, Writing–review and editing. YA-L: Funding acquisition, Investigation, Resources, Visualization, Writing–review and editing.
The author(s) declare no financial support was received for the research, authorship, and/or publication of this article.
The authors declare that the research was conducted in the absence of any commercial or financial relationships that could be construed as a potential conflict of interest.
All claims expressed in this article are solely those of the authors and do not necessarily represent those of their affiliated organizations, or those of the publisher, the editors and the reviewers. Any product that may be evaluated in this article, or claim that may be made by its manufacturer, is not guaranteed or endorsed by the publisher.
Abraham, K. M., Foos, J. S., and Goldman, J. L. (1984). Long cycle-life secondary lithium cells utilizing tetrahydrofuran. J. Electrochem. Soc. 131, 2197–2199. doi:10.1149/1.2116049
Aguesse, F., Manalastas, W., Buannic, L., Lopez del Amo, J. M., Singh, G., Llordés, A., et al. (2017). Investigating the dendritic growth during full cell cycling of garnet electrolyte in direct contact with Li metal. ACS Appl. Mat. Interfaces 9, 3808–3816. doi:10.1021/acsami.6b13925
Albertus, P., Babinec, S., Litzelman, S., and Newman, A. (2017). Status and challenges in enabling the lithium metal electrode for high-energy and low-cost rechargeable batteries. Nat. Energy 31 (3), 16–21. doi:10.1038/s41560-017-0047-2
Al-Salih, H., Baranova, E., and Abu-Lebdeh, Y. (2024). Solid-state batteries: the interfacial challenge to replace liquid electrolytes. Encycl. Solid-Liquid Interfaces, 444–453. doi:10.1016/B978-0-323-85669-0.00146-X
Al-Salih, H., Seif, M., Houache, E., Baranova, E. A., and Abu-Lebdeh, Y. (2022). Composite cathodes for solid-state lithium batteries: “catholytes” the underrated giants. Adv. Energy Sustain. Res. 3, 2200032. doi:10.1002/aesr.202200032
Amanchukwu, C. V., Yu, Z., Kong, X., Qin, J., Cui, Y., and Bao, Z. (2020). A new class of ionically conducting fluorinated ether electrolytes with high electrochemical stability. J. Am. Chem. Soc. 142, 7393–7403. doi:10.1021/jacs.9b11056
Aurbach, D., Gofer, Y., Ben-Zion, M., and Aped, P. (1992). The behaviour of lithium electrodes in propylene and ethylene carbonate: Te major factors that influence Li cycling efficiency. J. Electroanal. Chem. 339, 451–471. doi:10.1016/0022-0728(92)80467-i
Aurbach, D., and Zaban, A. (1994). Impedance spectroscopy of nonactive metal electrodes at low potentials in propylene carbonate solutions: a comparison to studies of Li electrodes. J. Electrochem. Soc. 141, 1808–1819. doi:10.1149/1.2055009
Balo, L., Gupta, H., Singh, S. K., Singh, V. K., Kataria, S., and Singh, R. K. (2018). Performance of EMIMFSI ionic liquid based gel polymer electrolyte in rechargeable lithium metal batteries. J. Ind. Eng. Chem. 65, 137–145. doi:10.1016/j.jiec.2018.04.022
Cao, X., Ren, X., Zou, L., Engelhard, M. H., Huang, W., Wang, H., et al. (2019). Monolithic solid–electrolyte interphases formed in fluorinated orthoformate-based electrolytes minimize Li depletion and pulverization. Nat. Energy 4, 796–805. doi:10.1038/s41560-019-0464-5
Chen, H., Jing, M., Han, C., Yang, H., Hua, S., Chen, F., et al. (2019a). A novel organic/inorganic composite solid electrolyte with functionalized layers for improved room-temperature rate performance of solid-state lithium battery. Int. J. Energy Res. 43, 5912–5921. doi:10.1002/er.4699
Chen, J., Li, Q., Pollard, T. P., Fan, X., Borodin, O., and Wang, C. (2020). Electrolyte design for Li metal-free Li batteries. Mat. Today 39, 118–126. doi:10.1016/j.mattod.2020.04.004
Chen, L., Fan, X., Hu, E., Ji, X., Chen, J., Hou, S., et al. (2019b). Achieving high energy density through increasing the output voltage: a highly reversible 5.3 V battery. Chem 5, 896–912. doi:10.1016/j.chempr.2019.02.003
Chen, N., Li, Y., Dai, Y., Qu, W., Xing, Y., Ye, Y., et al. (2019c). A Li+ conductive metal organic framework electrolyte boosts the high-temperature performance of dendrite-free lithium batteries. J. Mat. Chem. A 7, 9530–9536. doi:10.1039/c8ta12539b
Chen, S., Zheng, J., Mei, D., Han, K. S., Engelhard, M. H., Zhao, W., et al. (2018a). High-voltage lithium-metal batteries enabled by localized high-concentration electrolytes. Adv. Mat. 30, 1706102. doi:10.1002/adma.201706102
Chen, S., Zheng, J., Mei, D., Han, K. S., Engelhard, M. H., Zhao, W., et al. (2018c). High-voltage lithium-metal batteries enabled by localized high-concentration electrolytes. Adv. Mat. 30, 1706102. doi:10.1002/adma.201706102
Chen, S., Zheng, J., Yu, L., Ren, X., Engelhard, M. H., Niu, C., et al. (2018b). High-efficiency lithium metal batteries with fire-retardant electrolytes. Joule 2, 1548–1558. doi:10.1016/j.joule.2018.05.002
Chen, S., Zheng, J., Yu, L., Ren, X., Engelhard, M. H., Niu, C., et al. (2018d). High-efficiency lithium metal batteries with fire-retardant electrolytes. Joule 2, 1548–1558. doi:10.1016/j.joule.2018.05.002
Cheng, X. B., Zhao, M. Q., Chen, C., Pentecost, A., Maleski, K., Mathis, T., et al. (2017). Nanodiamonds suppress the growth of lithium dendrites. Nat. Commun. 8, 336. doi:10.1038/s41467-017-00519-2
Chu, S., Cui, Y., and Liu, N. (2016). The path towards sustainable energy. Nat. Mat. 16, 16–22. doi:10.1038/nmat4834
Cui, Y., Wan, J., Ye, Y., Liu, K., and Chou, L. Y. (2020). A fireproof, lightweight, polymer–polymer solid-state electrolyte for safe lithium batteries. Nano Lett. 20, 1686–1692. doi:10.1021/acs.nanolett.9b04815
Dai, Z., Yu, J., Liu, J., Liu, R., Sun, Q., Chen, D., et al. (2020). Highly conductive and nonflammable composite polymer electrolytes for rechargeable quasi-solid-state Li-metal batteries. J. Power Sources 464, 228182. doi:10.1016/j.jpowsour.2020.228182
Deng, T., Fan, X., Cao, L., Chen, J., Hou, S., Ji, X., et al. (2019). Designing in-situ-formed interphases enables highly reversible cobalt-free LiNiO2 cathode for Li-ion and Li-metal batteries. Joule 3, 2550–2564. doi:10.1016/j.joule.2019.08.004
Ding, C., Fu, X., Li, H., Yang, J., Lan, J., Yu, Y., et al. (2019). An ultrarobust composite gel electrolyte stabilizing ion deposition for long-life lithium metal batteries. Adv. Funct. Mat. 29, 1904547. doi:10.1002/adfm.201904547
Doi, T., Shimizu, Y., Hashinokuchi, M., and Inaba, M. (2017). Dilution of highly concentrated libf4/propylene carbonate electrolyte solution with fluoroalkyl ethers for 5-V LiNi0.5Mn 1.5O4 positive electrodes. J. Electrochem. Soc. 164, A6412–A6416. doi:10.1149/2.0611701jes
Dokko, K., Tachikawa, N., Yamauchi, K., Tsuchiya, M., Yamazaki, A., Takashima, E., et al. (2013). Solvate ionic liquid electrolyte for Li-S batteries. J. Electrochem. Soc. 160, A1304–A1310. doi:10.1149/2.111308jes
Dong, L., Zhong, S., Yuan, B., Ji, Y., Liu, J., Liu, Y., et al. (2022). Electrolyte engineering for high-voltage lithium metal batteries. Research 2022, 9837586. doi:10.34133/2022/9837586
Duan, H., Fan, M., Chen, W., Li, J., Wang, P., Wang, W., et al. (2019). Extended electrochemical window of solid electrolytes via heterogeneous multilayered structure for high-voltage lithium metal batteries. Adv. Mat. 31, 1807789. doi:10.1002/adma.201807789
Fan, X., Chen, L., Borodin, O., Ji, X., Chen, J., Hou, S., et al. (2018a). Non-flammable electrolyte enables Li-metal batteries with aggressive cathode chemistries. Nat. Nanotechnol. 138 (13), 715–722. doi:10.1038/s41565-018-0183-2
Fan, X., Chen, L., Ji, X., Deng, T., Hou, S., Chen, J., et al. (2018b). Highly fluorinated interphases enable high-voltage Li-metal batteries. Chem 4, 174–185. doi:10.1016/j.chempr.2017.10.017
Fan, X., Ji, X., Chen, L., Chen, J., Deng, T., Han, F., et al. (2019). All-temperature batteries enabled by fluorinated electrolytes with non-polar solvents. Nat. Energy 4, 882–890. doi:10.1038/s41560-019-0474-3
Fu, C., Ma, Y., Zuo, P., Zhao, W., Tang, W., Yin, G., et al. (2021). In-situ thermal polymerization boosts succinonitrile-based composite solid-state electrolyte for high performance Li-metal battery. J. Power Sources 496, 229861. doi:10.1016/j.jpowsour.2021.229861
Guo, X., Hao, L., Yang, Y., Wang, Y., Lu, Y., and Yu, H. (2019). High cathode utilization efficiency through interface engineering in all-solid-state lithium-metal batteries. J. Mat. Chem. A 7, 25915–25924. doi:10.1039/c9ta09935b
Hagos, T. T., Thirumalraj, B., Huang, C. J., Abrha, L. H., Berhe, G. B., et al. (2019). Locally concentrated LiPF 6 in a carbonate-based electrolyte with fluoroethylene carbonate as a diluent for anode-free lithium metal batteries. ACS Appl. Mat. Interfaces 11, 9955–9963. doi:10.1021/acsami.8b21052
Hu, J., He, P., Zhang, B., Wang, B., and Fan, L. Z. (2020b). Porous film host-derived 3D composite polymer electrolyte for high-voltage solid state lithium batteries. Energy Storage Mater 26, 283–289. doi:10.1016/j.ensm.2020.01.006
Hu, J., Yao, Z., Chen, K., and Li, C. (2020a). High-conductivity open framework fluorinated electrolyte bonded by solidified ionic liquid wires for solid-state Li metal batteries. Energy Storage Mater 28, 37–46. doi:10.1016/j.ensm.2020.02.018
Jeong, S. K., Inaba, M., Iriyama, Y., Abe, T., and Ogumi, Z. (2003). Electrochemical intercalation of lithium ion within graphite from propylene carbonate solutions. Electrochem. Solid-State Lett. 6, A13. doi:10.1149/1.1526781
Jeong, S. K., Inaba, M., Iriyama, Y., Abe, T., and Ogumi, Z. (2008a). Interfacial reactions between graphite electrodes and propylene carbonate-based solutions: electrolyte-concentration dependence of electrochemical lithium intercalation reaction. J. Power Sources 175, 540–546. doi:10.1016/j.jpowsour.2007.08.065
Jeong, S. K., Seo, H. Y., Kim, D. H., Han, H. K., Kim, J. G., Lee, Y. B., et al. (2008b). Suppression of dendritic lithium formation by using concentrated electrolyte solutions. Electrochem. Commun. 10, 635–638. doi:10.1016/j.elecom.2008.02.006
Jiang, Z., Wang, S., Chen, X., Yang, W., Yao, X., Hu, X., et al. (2020). Tape-casting Li0.34La0.56TiO3 ceramic electrolyte films permit high energy density of lithium-metal batteries. Adv. Mat. 32, 1906221. doi:10.1002/adma.201906221
Jiao, S., Ren, X., Cao, R., Engelhard, M. H., Liu, Y., Hu, D., et al. (2018). Stable cycling of high-voltage lithium metal batteries in ether electrolytes. Nat. Energy 39 (3), 739–746. doi:10.1038/s41560-018-0199-8
Kaup, K., Bazak, J. D., Vajargah, S. H., Wu, X., Kulisch, J., Goward, G. R., et al. (2020). A lithium oxythioborosilicate solid electrolyte glass with superionic conductivity. Adv. Energy Mat. 10, 1902783. doi:10.1002/aenm.201902783
Khurana, R., Schaefer, J. L., Archer, L. A., and Coates, G. W. (2014). Suppression of lithium dendrite growth using cross-linked polyethylene/poly(ethylene oxide) electrolytes: a new approach for practical lithium-metal polymer batteries. J. Am. Chem. Soc. 136, 7395–7402. doi:10.1021/ja502133j
Kim, M. S., Zhang, Z., Rudnicki, P. E., Yu, Z., Wang, J., Wang, H., et al. (2022). Suspension electrolyte with modified Li+ solvation environment for lithium metal batteries. Nat. Mat. 21, 445–454. doi:10.1038/s41563-021-01172-3
Li, A., Liao, X., Zhang, H., Shi, L., Wang, P., Cheng, Q., et al. (2020d). Nacre-Inspired composite electrolytes for load-bearing solid-state lithium-metal batteries. Adv. Mat. 32, 1905517. doi:10.1002/adma.201905517
Li, L., Wang, M., Wang, J., Ye, F., Wang, S., Xu, Y., et al. (2020a). Asymmetric gel polymer electrolyte with high lithium ion conductivity for dendrite-free lithium metal batteries. J. Mat. Chem. A 8, 8033–8040. doi:10.1039/d0ta01883j
Li, S., Zhang, W., Wu, Q., Fan, L., Wang, X., Wang, X., et al. (2020b). Synergistic dual-additive electrolyte enables practical lithium-metal batteries. Angew. Chem. - Int. Ed. 59, 14935–14941. doi:10.1002/anie.202004853
Li, T., Zhang, X. Q., Shi, P., and Zhang, Q. (2019a). Fluorinated solid-electrolyte interphase in high-voltage lithium metal batteries. Joule 3, 2647–2661. doi:10.1016/j.joule.2019.09.022
Li, W., Yao, H., Yan, K., Zheng, G., Liang, Z., Chiang, Y. M., et al. (2015). The synergetic effect of lithium polysulfide and lithium nitrate to prevent lithium dendrite growth. Nat. Commun. 6, 7436. doi:10.1038/ncomms8436
Li, Y., Sun, Z., Liu, D., Gao, Y., Wang, Y., Bu, H., et al. (2020c). A composite solid polymer electrolyte incorporating MnO2 nanosheets with reinforced mechanical properties and electrochemical stability for lithium metal batteries. J. Mat. Chem. A 8, 2021–2032. doi:10.1039/c9ta11542k
Li, Y., Zhang, W., Dou, Q., Wong, K. W., and Ng, K. M. (2019b). Li7La3Zr2O12 ceramic nanofiber-incorporated composite polymer electrolytes for lithium metal batteries. J. Mat. Chem. A 7, 3391–3398. doi:10.1039/c8ta11449h
Lin, D., Liu, Y., and Cui, Y. (2017). Reviving the lithium metal anode for high-energy batteries. Nat. Nanotechnol. 123 (12), 194–206. doi:10.1038/nnano.2017.16
Lin, X., Chu, C., Li, Z., Zhang, T., Chen, J., Liu, R., et al. (2021). A high-performance, solution-processable polymer/ceramic/ionic liquid electrolyte for room temperature solid-state Li metal batteries. Nano Energy 89, 106351. doi:10.1016/j.nanoen.2021.106351
Liu, J., Bao, Z., Cui, Y., Dufek, E. J., Goodenough, J. B., Khalifah, P., et al. (2019a). Pathways for practical high-energy long-cycling lithium metal batteries. Nat. Energy 4, 180–186. doi:10.1038/s41560-019-0338-x
Liu, J., Yuan, H., Liu, H., Zhao, C., Lu, Y., Cheng, X., et al. (2022). Unlocking the failure mechanism of solid state lithium metal batteries. Adv. Energy Mat. 12, 2100748. doi:10.1002/aenm.202100748
Liu, J., Zhou, J., Wang, M., Niu, C., Qian, T., and Yan, C. (2019b). A functional-gradient-structured ultrahigh modulus solid polymer electrolyte for all-solid-state lithium metal batteries. J. Mat. Chem. A 7, 24477–24485. doi:10.1039/c9ta07876b
Liu, K., Wu, M., Wei, L., Lin, Y., and Zhao, T. (2020). A composite solid electrolyte with a framework of vertically aligned perovskite for all-solid-state Li-metal batteries. J. Memb. Sci. 610, 118265. doi:10.1016/j.memsci.2020.118265
Liu, L., and Sun, C. (2020). Flexible quasi-solid-state composite electrolyte membrane derived from a metal-organic framework for lithium-metal batteries. ChemElectroChem 7, 707–715. doi:10.1002/celc.201902032
Liu, W., Yi, C., Li, L., Liu, S., Gui, Q., Ba, D., et al. (2021b). Designing polymer-in-salt electrolyte and fully infiltrated 3D electrode for integrated solid-state lithium batteries. Angew. Chem. Int. Ed. 60, 12931–12940. doi:10.1002/anie.202101537
Liu, Y., Lin, D., Li, Y., Chen, G., Pei, A., Nix, O., et al. (2018). Solubility-mediated sustained release enabling nitrate additive in carbonate electrolytes for stable lithium metal anode. Nat. Commun. 9, 3656. doi:10.1038/s41467-018-06077-5
Liu, Y., Zhao, Y., Lu, W., Sun, L., Lin, L., Zheng, M., et al. (2021a). PEO based polymer in plastic crystal electrolytes for room temperature high-voltage lithium metal batteries. Nano Energy 88, 106205. doi:10.1016/j.nanoen.2021.106205
Liu, Y., Zhou, G., Liu, K., and Cui, Y. (2017). Design of complex nanomaterials for energy storage: past success and future opportunity. Acc. Chem. Res. 50, 2895–2905. doi:10.1021/acs.accounts.7b00450
Louli, A. J., Eldesoky, A., Weber, R., Genovese, M., Coon, M., deGooyer, J., et al. (2020). Diagnosing and correcting anode-free cell failure via electrolyte and morphological analysis. Nat. Energy 59 (5), 693–702. doi:10.1038/s41560-020-0668-8
Louli, A. J., Genovese, M., Weber, R., Hames, S. G., Logan, E. R., and Dahn, J. R. (2019). Exploring the impact of mechanical pressure on the performance of anode-free lithium metal cells. J. Electrochem. Soc. 166, A1291–A1299. doi:10.1149/2.0091908jes
Lu, Y., Tu, Z., and Archer, L. A. (2014). Stable lithium electrodeposition in liquid and nanoporous solid electrolytes. Nat. Mat. 13, 961–969. doi:10.1038/nmat4041
Lu, Y., Zhang, X., Xue, C., Xin, C., Li, M., Nan, C. w., et al. (2020). Three-dimensional structured asymmetric electrolytes for high interface stability and fast Li-ion transport in solid-state Li-metal batteries. Mat. Today Energy 18, 100522. doi:10.1016/j.mtener.2020.100522
Maeyoshi, Y., Ding, D., Kubota, M., Ueda, H., Abe, K., Kanamura, K., et al. (2019). Long-term stable lithium metal anode in highly concentrated sulfolane-based electrolytes with ultrafine porous polyimide separator. ACS Appl. Mat. Interfaces 11, 25833–25843. doi:10.1021/acsami.9b05257
Matsuda, Y. (1993). Behavior of lithium/electrolyte interface in organic solutions. J. Power Sources 43, 1–7. doi:10.1016/0378-7753(93)80096-8
McKinnon, W. R., and Dahn, J. R. (1985). How to reduce the cointercalation of propylene carbonate in LixZrS2 and other layered compounds. J. Electrochem. Soc. 132, 364–366. doi:10.1149/1.2113839
Mezzomo, L., Pianta, N., Ostroman, I., Aloni, N., Golodnitsky, D., Peled, E., et al. (2023). Deep eutectic solvent electrolytes based on trifluoroacetamide and LiPF6 for Li-metal batteries. J. Power Sources 561, 232746. doi:10.1016/j.jpowsour.2023.232746
Monroe, C., and Newman, J. (2005). The impact of elastic deformation on deposition kinetics at lithium/polymer interfaces. J. Electrochem. Soc. 152, A396. doi:10.1149/1.1850854
Moon, H., Mandai, T., Tatara, R., Ueno, K., Yamazaki, A., Yoshida, K., et al. (2015). Solvent activity in electrolyte solutions controls electrochemical reactions in Li-Ion and Li-Sulfur batteries. J. Phys. Chem. C 119, 3957–3970. doi:10.1021/jp5128578
Pan, K., Zhang, L., Qian, W., Wu, X., Dong, K., Zhang, H., et al. (2020). A flexible ceramic/polymer hybrid solid electrolyte for solid-state lithium metal batteries. Adv. Mat. 32, 2000399. doi:10.1002/adma.202000399
Peled, E. (1979). The electrochemical behavior of alkali and alkaline earth metals in nonaqueous battery systems—the solid electrolyte interphase model. J. Electrochem. Soc. 126, 2047–2051. doi:10.1149/1.2128859
Pervez, S. A., Ganjeh-Anzabi, P., Farooq, U., Trifkovic, M., Roberts, E. P. L., and Thangadurai, V. (2019). Fabrication of a dendrite-free all solid-state Li metal battery via polymer composite/garnet/polymer composite layered electrolyte. Adv. Mat. Interfaces 6. doi:10.1002/admi.201900186
Qian, J., Adams, B. D., Zheng, J., Xu, W., Henderson, W. A., Wang, J., et al. (2016). Anode-free rechargeable lithium metal batteries. Adv. Funct. Mat. 26, 7094–7102. doi:10.1002/adfm.201602353
Qian, J., Henderson, W. A., Xu, W., Bhattacharya, P., Engelhard, M. H., Borodin, O., et al. (2015). High rate and stable cycling of lithium metal anode. Nat. Commun. 61 (6), 1155–1159. doi:10.1149/ma2015-01/15/1155
Qiao, Y., Yang, H., Chang, Z., Deng, H., Li, X., and Zhou, H. (2021). A high-energy-density and long-life initial-anode-free lithium battery enabled by a Li2O sacrificial agent. Nat. Energy 66 (6), 653–662. doi:10.1038/s41560-021-00839-0
Qiu, G., and Sun, C. (2020). A quasi-solid composite electrolyte with dual salts for dendrite-free lithium metal batteries. New J. Chem. 44, 1817–1824. doi:10.1039/c9nj04897a
Qiu, J., Liu, X., Chen, R., Li, Q., Wang, Y., Chen, P., et al. (2020). Enabling stable cycling of 4.2 V high-voltage all-solid-state batteries with PEO-based solid electrolyte. Adv. Funct. Mat. 30, 1909392. doi:10.1002/adfm.201909392
Ren, X., Zhang, Y., Engelhard, M. H., Li, Q., Zhang, J. G., and Xu, W. (2018). Guided lithium metal deposition and improved lithium coulombic efficiency through synergistic effects of LiAsF6 and cyclic carbonate additives. ACS Energy Lett. 3, 14–19. doi:10.1021/acsenergylett.7b00982
Ren, X., Zou, L., Cao, X., Engelhard, M. H., Liu, W., Burton, S. D., et al. (2019). Enabling high-voltage lithium-metal batteries under practical conditions. Joule 3, 1662–1676. doi:10.1016/j.joule.2019.05.006
Shadike, Z., Lee, H., Borodin, O., Cao, X., Fan, X., Wang, X., et al. (2021). Identification of LiH and nanocrystalline LiF in the solid–electrolyte interphase of lithium metal anodes. Nat. Nanotechnol. 16, 549–554. doi:10.1038/s41565-020-00845-5
Shi, P., Zheng, H., Liang, X., Sun, Y., Cheng, S., Chen, C., et al. (2018). A highly concentrated phosphate-based electrolyte for high-safety rechargeable lithium batteries. Chem. Commun. 54, 4453–4456. doi:10.1039/c8cc00994e
Song, X., Yu, W., Zhou, S., Zhao, L., Li, A., Wu, A., et al. (2023). Enhancement of Mn-doped LiPON electrolyte for higher performance of all-solid-state thin film lithium battery. Mat. Today Phys. 33, 101037. doi:10.1016/j.mtphys.2023.101037
Stark, J. K., Ding, Y., and Kohl, P. A. (2011). Dendrite-free electrodeposition and reoxidation of lithium-sodium alloy for metal-anode battery. J. Electrochem. Soc. 158, A1100–A1105. doi:10.1149/1.3622348
Su, L., and Manthiram, A. (2022). Lithium-metal batteries via suppressing Li dendrite growth and improving coulombic efficiency. Small Struct. 3, 2200114. doi:10.1002/sstr.202200114
Sun, H., Zhu, G., Zhu, Y., Lin, M., Chen, H., et al. (2020). High-safety and high-energy-density lithium metal batteries in a novel ionic-liquid electrolyte. Adv. Mat. 32, 2001741. doi:10.1002/adma.202001741
Sun, J., Yao, X., Song, A., Li, Y., Zhang, Q., et al. (2021). Hierarchical composite-solid-electrolyte with high electrochemical stability and interfacial regulation for boosting ultra-stable lithium batteries. Adv. Funct. Mat. 31, 2006381. doi:10.1002/adfm.202006381
Suo, L., Borodin, O., Gao, T., Olguin, M., Ho, J., Fan, X., et al. (2015). ‘Water-in-salt’ electrolyte enables high-voltage aqueous lithium-ion chemistries. Sci. (80) 350, 938–943. doi:10.1126/science.aab1595
Suo, L., Xue, W., Gobet, M., Greenbaum, S. G., Wang, C., Chen, Y., et al. (2018). Fluorine-donating electrolytes enable highly reversible 5-V-class Li metal batteries. Proc. Natl. Acad. Sci. U. S. A. 115, 1156–1161. doi:10.1073/pnas.1712895115
Tan, S. J., Yue, J., Hu, X., Shen, Z., Wang, W., Li, J., et al. (2019). Nitriding-interface-regulated lithium plating enables flame-retardant electrolytes for high-voltage lithium metal batteries. Angew. Chem. - Int. Ed. 58, 7802–7807. doi:10.1002/anie.201903466
Tian, L. W., Kim, J. W., Hong, S. B., Ryu, H. H., Kim, U. H., Sun, Y. K., et al. (2022). All-solid-state lithium batteries featuring hybrid electrolytes based on Li+ ion-conductive Li7La3Zr2O12 framework and full-concentration gradient Ni-rich NCM cathode. Chem. Eng. J. 450, 138043. doi:10.1016/j.cej.2022.138043
Tsai, C. L., Roddatis, V., Chandran, C. V., Ma, Q., Uhlenbruck, S., Bram, M., et al. (2016). Li7La3Zr2O12 interface modification for Li dendrite prevention. ACS Appl. Mat. Interfaces 8, 10617–10626. doi:10.1021/acsami.6b00831
Ueno, K., Murai, J., Ikeda, K., Tsuzuki, S., Tsuchiya, M., Tatara, R., et al. (2016). Li+ solvation and ionic transport in lithium solvate ionic liquids diluted by molecular solvents. J. Phys. Chem. C 120, 15792–15802. doi:10.1021/acs.jpcc.5b11642
Vega, J. A., Zhou, J., and Kohl, P. A. (2009). Electrochemical comparison and deposition of lithium and potassium from phosphonium- and ammonium-TFSI ionic liquids. J. Electrochem. Soc. 156, A253–A259. doi:10.1149/1.3070657
Wang, H., Yu, Z., Kong, X., Huang, W., Zhang, Z., Mackanic, D. G., et al. (2021a). Dual-solvent Li-ion solvation enables high-performance Li-metal batteries. Adv. Mat. 33, 2008619. doi:10.1002/adma.202008619
Wang, H., Yu, Z., Kong, X., Kim, S. C., Boyle, D. T., Qin, J., et al. (2022). Liquid electrolyte: the nexus of practical lithium metal batteries. Joule 6, 588–616. doi:10.1016/j.joule.2021.12.018
Wang, J., Huang, W., Pei, A., Shi, F., Yu, X., et al. (2019a). Improving cyclability of Li metal batteries at elevated temperatures and its origin revealed by cryo-electron microscopy. Nat. Energy 48 (4), 664–670. doi:10.1038/s41560-019-0413-3
Wang, J., Lin, F., Jia, H., Yang, J., Monroe, C. W., and NuLi, Y. (2014). Towards a safe lithium-sulfur battery with a flame-inhibiting electrolyte and a sulfur-based composite cathode. Angew. Chem. - Int. Ed. 53, 10099–10104. doi:10.1002/anie.201405157
Wang, Q., Liu, B., Shen, Y., Wu, J., Zhao, Z., Zhong, C., et al. (2021b). Confronting the challenges in lithium anodes for lithium metal batteries. Adv. Sci. 8, 2101111. doi:10.1002/advs.202101111
Wang, X., Girard, G. M. A., Zhu, H., Yunis, R., MacFarlane, D. R., Mecerreyes, D., et al. (2019b). Poly(ionic liquid)s/electrospun nanofiber composite polymer electrolytes for high energy density and safe Li metal batteries. ACS Appl. Energy Mat. 2, 6237–6245. doi:10.1021/acsaem.9b00765
Wen, J., Huang, Y., Duan, J., Wu, Y., Luo, W., Zhou, L., et al. (2019). Highly adhesive Li-bn nanosheet composite anode with excellent interfacial compatibility for solid-state Li metal batteries. ACS Nano 13, 14549–14556. doi:10.1021/acsnano.9b08803
Wu, H., Xu, Y., Ren, X., Liu, B., Engelhard, M. H., Ding, M. S., et al. (2019). Polymer-in-“Quasi-Ionic liquid” electrolytes for high-voltage lithium metal batteries. Adv. Energy Mat. 9, 1902108. doi:10.1002/aenm.201902108
Wu, X., Chen, K., Yao, Z., Hu, J., Huang, M., Meng, J., et al. (2021). Metal organic framework reinforced polymer electrolyte with high cation transference number to enable dendrite-free solid state Li metal conversion batteries. J. Power Sources 501, 229946. doi:10.1016/j.jpowsour.2021.229946
Xiong, S., Liu, Y., Jankowski, P., Liu, Q., Nitze, F., Xie, K., et al. (2020). Design of a multifunctional interlayer for NASCION-based solid-state Li metal batteries. Adv. Funct. Mat. 30, 2001444. doi:10.1002/adfm.202001444
Xu, G., Li, J., Wang, C., Du, X., Lu, D., Xie, B., et al. (2021). The Formation/Decomposition equilibrium of LiH and its contribution on anode failure in practical lithium metal batteries. Angew. Chem. - Int. Ed. 60, 7770–7776. doi:10.1002/anie.202013812
Xu, K. (2004). Nonaqueous liquid electrolytes for lithium-based rechargeable batteries. Chem. Rev. 104, 4303–4417. doi:10.1021/cr030203g
Xue, W., Shi, Z., Huang, M., Feng, S., Wang, C., Wang, F., et al. (2020). FSI-inspired solvent and “full fluorosulfonyl” electrolyte for 4 V class lithium-metal batteries. Energy Environ. Sci. 13, 212–220. doi:10.1039/c9ee02538c
Yamada, Y., Furukawa, K., Sodeyama, K., Kikuchi, K., Yaegashi, M., Tateyama, Y., et al. (2014). Unusual stability of acetonitrile-based superconcentrated electrolytes for fast-charging lithium-ion batteries. J. Am. Chem. Soc. 136, 5039–5046. doi:10.1021/ja412807w
Yamada, Y., Takazawa, Y., Miyazaki, K., and Abe, T. (2010). Electrochemical lithium intercalation into graphite in dimethyl sulfoxide-based electrolytes: effect of solvation structure of lithium ion. J. Phys. Chem. C 114, 11680–11685. doi:10.1021/jp1037427
Yamada, Y., and Yamada, A. (2015a). Review—superconcentrated electrolytes for lithium batteries. J. Electrochem. Soc. 162, A2406–A2423. doi:10.1149/2.0041514jes
Yamada, Y., and Yamada, A. (2015b). Review—superconcentrated electrolytes for lithium batteries. J. Electrochem. Soc. 162, A2406–A2423. doi:10.1149/2.0041514jes
Yan, C., Li, H. R., Chen, X., Zhang, X. Q., Cheng, X. B., Xu, R., et al. (2019). Regulating the inner Helmholtz plane for stable solid electrolyte interphase on lithium metal anodes. J. Am. Chem. Soc. 141, 9422–9429. doi:10.1021/jacs.9b05029
Yan, C., Yao, Y., Chen, X., Cheng, X., Zhang, X., Huang, J., et al. (2018). Lithium nitrate solvation chemistry in carbonate electrolyte sustains high-voltage lithium metal batteries. Angew. Chem. - Int. Ed. 57, 14055–14059. doi:10.1002/anie.201807034
Yang, H., Zhang, B., Jing, M., Shen, X., Wang, L., Xu, H., et al. (2022). In situ catalytic polymerization of a highly homogeneous PDOL composite electrolyte for long-cycle high-voltage solid-state lithium batteries. Adv. Energy Mat. 12, 2201762. doi:10.1002/aenm.202201762
Yang, T., Li, S., Wang, W., Lu, J., Fan, W., Zuo, X., et al. (2021b). Nonflammable functional electrolytes with all-fluorinated solvents matching rechargeable high-voltage Li-metal batteries with Ni-rich ternary cathode. J. Power Sources 505, 230055. doi:10.1016/j.jpowsour.2021.230055
Yang, Z., Sun, Z., Liu, C., Li, Y., Zhou, G., Zuo, S., et al. (2021a). Lithiated nanosheets hybridized solid polymer electrolyte to construct Li+ conduction highways for advanced all-solid-state lithium battery. J. Power Sources 484, 229287. doi:10.1016/j.jpowsour.2020.229287
Yoon, S., Lee, J., Kim, S. O., and Sohn, H. J. (2008). Enhanced cyclability and surface characteristics of lithium batteries by Li-Mg co-deposition and addition of HF acid in electrolyte. Electrochim. Acta 53, 2501–2506. doi:10.1016/j.electacta.2007.10.019
Yu, Z., Wang, H., Kong, X., Huang, W., Tsao, Y., Mackanic, D. G., et al. (2020). Molecular design for electrolyte solvents enabling energy-dense and long-cycling lithium metal batteries. Nat. Energy 57 (5), 526–533. doi:10.1038/s41560-020-0634-5
Yuan, S., Ding, K., Zeng, X., Bin, D., Zhang, Y., Dong, P., et al. (2023). Advanced nonflammable organic electrolyte promises safer Li-metal batteries: from solvation structure perspectives. Adv. Mat. 35, 2206228. doi:10.1002/adma.202206228
Zeng, Z., Murugesan, V., Han, K. S., Jiang, X., Cao, Y., Xiao, L., et al. (2018a). Non-flammable electrolytes with high salt-to-solvent ratios for Li-ion and Li-metal batteries. Nat. Energy 3, 674–681. doi:10.1038/s41560-018-0196-y
Zeng, Z., Murugesan, V., Han, K. S., Jiang, X., Cao, Y., Xiao, L., et al. (2018b). Non-flammable electrolytes with high salt-to-solvent ratios for Li-ion and Li-metal batteries. Nat. Energy 3, 674–681. doi:10.1038/s41560-018-0196-y
Zhang, H., Eshetu, G. G., Judez, X., Li, C., Rodriguez-Martínez, L. M., and Armand, M. (2018c). Electrolyte additives for lithium metal anodes and rechargeable lithium metal batteries: progress and perspectives. Angew. Chem. Int. Ed. 57, 15002–15027. doi:10.1002/anie.201712702
Zhang, H., Luo, J., Qi, M., Lin, S., Dong, Q., Li, H., et al. (2021b). Enabling lithium metal anode in nonflammable phosphate electrolyte with electrochemically induced chemical reactions. Angew. Chem. - Int. Ed. 60, 19183–19190. doi:10.1002/anie.202103909
Zhang, H., Qu, W., Chen, N., Huang, Y., Li, L., Wu, F., et al. (2018b). Ionic liquid electrolyte with highly concentrated LiTFSI for lithium metal batteries. Electrochim. Acta 285, 78–85. doi:10.1016/j.electacta.2018.07.231
Zhang, J. G., Xu, W., Xiao, J., Cao, X., and Liu, J. (2020a). Lithium metal anodes with nonaqueous electrolytes. Chem. Rev. 120, 13312–13348. doi:10.1021/acs.chemrev.0c00275
Zhang, S., Liang, T., Wang, D., Xu, Y., Cui, Y., Li, J., et al. (2021a). A stretchable and safe polymer electrolyte with a protecting-layer strategy for solid-state lithium metal batteries. Adv. Sci. 8, 2003241. doi:10.1002/advs.202003241
Zhang, W., Shen, Z., Li, S., Fan, L., Wang, X., Chen, F., et al. (2020b). Engineering wavy-nanostructured anode interphases with fast ion transfer kinetics: toward practical Li-metal full batteries. Adv. Funct. Mat. 30, 2003800. doi:10.1002/adfm.202003800
Zhang, X. Q., Chen, X., Cheng, X., Shen, X., Yan, C., et al. (2018d). Highly stable lithium metal batteries enabled by regulating the solvation of lithium ions in nonaqueous electrolytes. Angew. Chem. - Int. Ed. 57, 5301–5305. doi:10.1002/anie.201801513
Zhang, X. Q., Cheng, X. B., Chen, X., Yan, C., and Zhang, Q. (2017). Fluoroethylene carbonate additives to render uniform Li deposits in lithium metal batteries. Adv. Funct. Mat. 27, 1605989. doi:10.1002/adfm.201605989
Zhang, Z., Chen, S., Yang, J., Wang, J., Yao, L., Yao, X., et al. (2018a). Interface Re-engineering of Li 10 GeP 2 S 12 electrolyte and lithium anode for all-solid-state lithium batteries with ultralong cycle life. ACS Appl. Mat. Interfaces 10, 2556–2565. doi:10.1021/acsami.7b16176
Zhang, Z., Zhang, L., Liu, Y., Yang, T., Wang, Z., Yan, X., et al. (2019). Dendrite-free lithium-metal batteries at high rate realized using a composite solid electrolyte with an ester-PO4 complex and stable interphase. J. Mat. Chem. A 7, 23173–23181. doi:10.1039/c9ta08415k
Zhao, F., Liang, J., Yu, C., Sun, Q., Li, X., Adair, K., et al. (2020a). A versatile Sn-substituted argyrodite sulfide electrolyte for all-solid-state Li metal batteries. Adv. Energy Mat. 10. doi:10.1002/aenm.201903422
Zhao, H., Deng, N., Kang, W., Li, Z., Wang, G., and Cheng, B. (2020b). Highly multiscale structural Poly(vinylidene fluoridehexafluoropropylene)/poly-m-phenyleneisophthalamide separator with enhanced interface compatibility and uniform lithium-ion flux distribution for dendrite-proof lithium-metal batteries. Energy Storage Mater 26, 334–348. doi:10.1016/j.ensm.2019.11.005
Zheng, X., Yang, T., Wei, J., Wang, C., and Chen, M. (2021). Co-contribution of quenching and nanocrystallization on ionic-conductivity improvement of a composite electrolyte of polyethylene Oxide/Li7La3Zr2O12 nanofibers at 45 °C for all-solid-state Li metal batteries. J. Power Sources 496, 229843. doi:10.1016/j.jpowsour.2021.229843
Zhou, Q., Xu, B., Chien, P., Li, Y., Huang, B., Wu, N., et al. (2020). NASICON Li 1.2 Mg 0.1 Zr 1.9 (PO 4) 3 solid electrolyte for an all-solid-state Li-metal battery. Small Methods 4, 2000764. doi:10.1002/smtd.202000764
Keywords: lithium anode, lithium metal batteries, additives, fluorinated additives, composite cathodes, catholyte, solid electrolyte, liquid electrolyte
Citation: Al-Salih H, Khan HA, Baranova EA and Abu-Lebdeh Y (2023) Back to the future: towards the realization of lithium metal batteries using liquid and solid electrolytes. Front. Energy Res. 11:1325316. doi: 10.3389/fenrg.2023.1325316
Received: 21 October 2023; Accepted: 27 November 2023;
Published: 15 December 2023.
Edited by:
Xu Liu, Helmholtz-Institut Ulm (HIU), GermanyReviewed by:
Fanglin Wu, Wuhan University of Technology, ChinaCopyright © 2023 Al-Salih, Khan, Baranova and Abu-Lebdeh. This is an open-access article distributed under the terms of the Creative Commons Attribution License (CC BY). The use, distribution or reproduction in other forums is permitted, provided the original author(s) and the copyright owner(s) are credited and that the original publication in this journal is cited, in accordance with accepted academic practice. No use, distribution or reproduction is permitted which does not comply with these terms.
*Correspondence: Yaser Abu-Lebdeh, eWFzZXIuYWJ1LWxlYmRlaEBucmMtY25yYy5nYy5jYQ==
Disclaimer: All claims expressed in this article are solely those of the authors and do not necessarily represent those of their affiliated organizations, or those of the publisher, the editors and the reviewers. Any product that may be evaluated in this article or claim that may be made by its manufacturer is not guaranteed or endorsed by the publisher.
Research integrity at Frontiers
Learn more about the work of our research integrity team to safeguard the quality of each article we publish.