- 1State Key Laboratory of Shale Oil and Gas Enrichment Mechanisms and Effective Development, Beijing, China
- 2School of Civil and Resources Engineering, University of Science and Technology Beijing, Beijing, China
- 3Research Institute of Exploration and Production, Beijing, China
- 4School of Energy and Environmental Engineering, University of Science and Technology Beijing, Beijing, China
Shale oil has recently received considerable attention as a promising energy source due to its substantial reserves. However, the recovery of shale oil presents numerous challenges due to the low-porosity and low-permeability characteristics of shale reservoirs. To tackle this challenge, the introduction of surfactants capable of modifying wettability has been employed to enhance shale oil recovery. In this study, we perform molecular dynamics simulations to investigate the influence of surfactants on the alteration of wettability in shale reservoirs. Firstly, surfaces of kaolinite, graphene, and kerogen are constructed to represent the inorganic and organic constituents of shale reservoirs. The impact and underlying mechanisms of two types of ionic surfactants, namely, the anionic surfactant sodium dodecylbenzene sulfonate (SDBS) and cationic surfactant dodecyltrimethylammonium bromide (DTAB), on the wettability between oil droplets and surfaces are investigated. The wettability are analyzed from different aspects, including contact angle, centroid ordinates, and self-diffusion coefficient. Simulation results show that the presence of surfactants can modify the wetting characteristics of crude oil within shale reservoirs. Notably, a reversal of wettability has been observed for oil-wet kaolinite surfaces. As for kerogen surfaces, it is found that an optimal surfactant concentration exists, beyond which the further addition of surfactant may not enhance the efficiency of wettability alteration.
1 Introduction
With the rapid advancement of society and economy, traditional oil and gas resources cannot meet the increasing demand (Zheng et al., 2022; Sun et al., 2023). Hence, it is of great significance to enhance oil and gas production through advanced technologies. Despite recent great breakthroughs in untraditional oil exploration, a substantial amount of shale oil remains trapped within shale reservoirs due to their low-porosity and low-permeability characteristics, resulting in relatively low recovery rates (Zou et al., 2019; Tang et al., 2020; Sun C. et al., 2021). During oil extraction operations, residual oil gets trapped in the nanopores of shale reservoirs after water injection, presenting a significant challenge to the oil recovery process (Dang et al., 2022; Guo et al., 2022). In recent years, several enhanced oil recovery (EOR) techniques have been developed (Rezaei et al., 2018; Zhao et al., 2021; Yang et al., 2023), with surfactant flooding emerging as one of the most effective technologies for oil recovery enhancement (Liu et al., 2019a; Sun Y.-P. et al., 2021; Larestani et al., 2022). Surfactants exhibit diverse and unique physical and chemical properties, thereby offering considerable potential for optimizing oil recovery under varying conditions, including geological conditions, reservoir compositions, brine salinities, etc. (Tang et al., 2019)
From a macroscopic perspective, the deformability and mobility of oil droplets play a crucial role in determining the ease of detaching oil droplets from the rock surface of oil reservoirs, ultimately leading to enhanced oil recovery (Zhang et al., 2010; Pei et al., 2012). A Previous researchers have conducted extensive experimental and theoretical studies, which have elucidated two primary mechanisms through which surfactants enhance oil recovery. Firstly, surfactants reduce the interfacial tension between oil and water, thereby improving the fluidity of crude oil (Liu et al., 2022; Zhou et al., 2022). Secondly, they alter the wettability of reservoirs from lipophilic to hydrophilic, facilitating the release of more residual oil and enhancing oil recovery (Mirchi et al., 2015; Kubelka et al., 2021; Okunade et al., 2021). Currently, research on the influence of different wettability conditions on the adsorption and diffusion behaviors of shale reservoirs is still in its early stages. Scholars hold different views on shale wettability due to differences in tectonic features and mineral compositions. Shale surface wettability is a critical factor in shale oil recovery as it directly affects the distribution of oil within shale reservoirs, the injection of oil displacement agents into the formation, and the effectiveness of oil displacement (Zhao and Jin, 2021; Shi et al., 2022).
The wettability of reservoir rocks can be influenced by external conditions. Despite extensive research efforts made by scholars worldwide to understand the mechanisms underlying reservoir wettability, a consensus has not yet been reached. Since reservoir rocks are formed in water environments, most of the component minerals are hydrophilic (Guo et al., 2012). Consequently, the initial state of the reservoir rock surface is hydrophilic, characterized by the presence of a water film on the surface. However, the generation and migration of oil and gas within the reservoir typically result in a transition from hydrophilic to lipophilic wettability (Su et al., 2018; Zhang et al., 2020). Furthermore, the wettability of reservoirs can be further modified by drilling fluids and various oil displacement agents such as water, chemicals, and CO2 (Al Mahrouqi et al., 2017; Yao et al., 2021; Deng et al., 2020; Zhang et al., 2022; Afekare et al., 2021; Qin et al. 2022). These modifications in wettability are often desirable since altering the distribution of reservoir fluids within the pore network through the introduction of oil displacement agents can enhance shale oil recovery.
Researchers have conducted experimental investigations to study the impact of surfactants on enhancing shale oil recovery. Mirchi et al. (Mirchi et al., 2015) investigated the dynamic interfacial tensions and contact angles in two brine/oil/shale systems in the presence of surfactants using the rising/captive bubble technique. They reported that anionic surfactants exhibit higher adsorption on shale compared to nonionic surfactants. SalahEldin Hussien et al. (SalahEldin Hussien et al., 2019) studied the effects of surfactants on fracturing fluid performance by measuring surface tension using the pendant drop technique. Their results revealed that surfactants significantly influenced the wettability of shale rock, rendering it more water-wet. Liu et al. (Liu et al., 2019b) utilized nuclear magnetic resonance (NMR) to evaluate the wettability of shales. Their results indicated that anionic surfactants are more effective compared to nonionic and cationic surfactants in altering the wettability of clay surfaces. Although certain quantitative results can be obtained, it remains challenging to observe the underlying mechanisms and microscopic processes solely through macroscopic experiments (Roshan et al., 2016).
With the rapid development of computer technology, molecular dynamic (MD) simulation is becoming an effective tool for unravelling the underlying mechanisms governing complex processes and behaviors at the microscale level (Zhou et al., 2019a; Zhou et al., 2019b; Zhou et al., 2020; Chen et al., 2023). Researchers have applied extensive MD simulations on investigating the wettability behaviors of shale reservoir for EOR. Chai et al. (2009) examined the wettabilities of surfaces of hydroxylated and silylated amorphous silica. They claimed that the fully silylated silica surface exhibit higher hydrophobicity, while the fully hydroxylated amorphous silica surface display greater hydrophilicity. Jagadisan and Heidari. (2022) conducted MD simulations to explore the influences of thermal maturity and reservoir temperature on the wettability performances of organic kerogen surfaces. Their simulation results indicated that the formed air/water/kerogen contact angle is maximal on type III and minimal on type II kerogen surfaces.Bai et al. (2020) utilized MD simulation to examine the correlation between molecular charge distribution and the efficiency of wettability reversal of quaternary ammonium type cationic surfactants on calcite surfaces. Their results revealed that the charge distribution on the hydrogen atoms within the methylene group of the quaternary ammonium moiety plays a critical role in determining the surfactants’ ability to modify wettability. By employing MD simulations, Chen et al. (2015) studied various functional groups on the silica surface. Their results suggested that the presence of different functional groups exhibit a significant impact on the surface contact angle of silica, leading to substantial changes in wettability. Kubelka and his co-workers (Kubelka et al., 2021) investigated the altered wettability of oil-wet calcite by surfactants through MD simulations. They claimed that cationic surfactants demonstrated greater efficiency in separating organic carboxylates and wettability alteration compared to brine.
Although many efforts have been devoted on investigating wettability alteration of oil reservoirs, studies on insightful mechanism and the effects of surfactant type, concentration, and surface properties on wettability of shale reservoirs are still limited. In the present work, we perform MD simulations to investigate the effects of different surfactants and reservoir properties on wettability alteration of shale reservoirs, taking into account their diverse constituents. The obtained results are expected to provide valuable insights and guidance for the development of effective surfactant flooding strategies for enhancing shale oil recovery.
2 Models and simulation methods
2.1 Physical models and force field
In this study, three types of surfaces, i.e., kaolinite, graphene, and kerogen surfaces are constructed to characterize the structures of inorganic, simplified organic, and organic surfaces in shale reservoirs, respectively. The Silica-Face-to-Gibbsite-Face (SG-FF) manner is used to construct the {0 0 1} octahedral surface model with dimensions of 81 × 78 × 14 Å3 of kaolinite, as shown in Figure 1A (Šolc et al., 2011; Tenney and Cygan, 2014; Sun H.-m. et al., 2021). A 33 × 18 × 2 supercell of the graphene unit cell structure with the size of 74 × 77 × 4 Å3 is built for the graphene surface model, as displayed in Figure 1B. The kerogen surface model is developed based on the relaxation process proposed by Michalec and Lisaĺ (Michalec and Lísal, 2017) and the modification by Yu et al. (2021) Firstly, 40 III-A type kerogen molecules are randomly inserted into a cuboid simulation box with an initial system density of approximately 0.05 g/cm3. Subsequently, the annealing process is performed following the relaxation protocol outlined in Table 1. After 12 cycles, a kerogen surface model with the size of 63.6 × 63.6 × 22.7 Å3 is obtained, as presented in Figure 1C. The final density of the model is 1.259 g/cm3, which is in good agreement with the experimental result of 1.28 ± 0.3 g/cm3 (Stankiewicz et al., 2015).
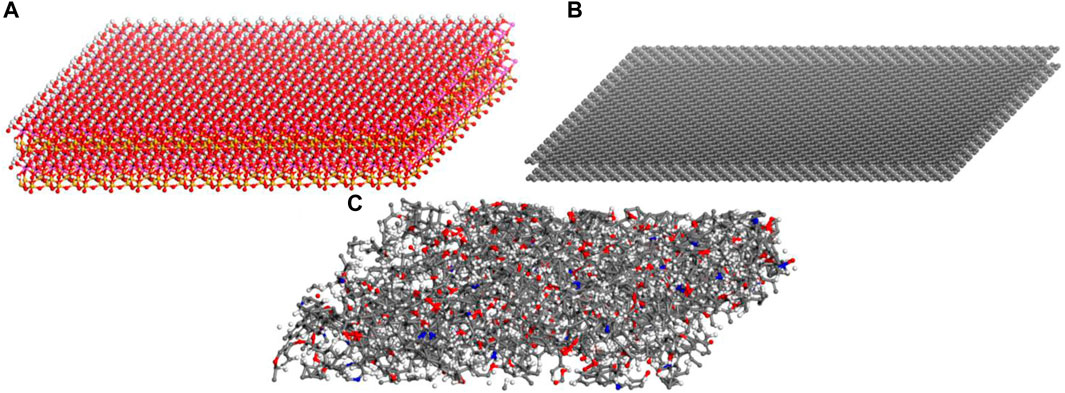
FIGURE 1. Different surface models: (A) kaolinite; (B) graphene; (C) kerogen. Red, white, yellow, purple, grey, and blue atoms represent oxygen, hydrogen, silicon, aluminium, carbon, and nitrogen, respectively.
In this study, the shale oil model is simplified with n-hexane, as depicted in Figure 2A. The oil molecules are parameterized using the polymer consistent force field (PCFF) (Sun et al., 1994; Plimpton, 1995; Chen et al., 2006; Cui et al., 2022), which has proven to be capable to accurately predict the structural and thermodynamic properties of oil components (Tang et al., 2019). The surfactants utilized in this study are the anionic surfactant sodium dodecylbenzene sulfonate (SDBS) and the cationic surfactant dodecyltrimethylammonium bromide (DTAB), as illustrated in Figures 2B,C. These surfactants are widely used in oil recovery experiments due to their commonality and ready availability. In this study, all molecular models are constructed using the Materials Studio package (version 2017).

FIGURE 2. Molecular models of (A) n-hexane, (B) sodium dodecylbenzene sulfonate, and (C) dodecyltrimethylammonium bromide. Grey, white, red, yellow, purple, earthy yellow, and blue represent carbon, hydrogen, oxygen, sulphur, sodium, bromine, and nitrogen, respectively.
2.2 Simulation details
Firstly, 120 n-hexane molecules are randomly placed onto a surface within a simulation box. Following geometrical optimization, the alkane molecules are superimposed on the surface forming an oil droplet. The system is then immersed in water and equilibrated in a canonical (NVT) ensemble at 330 K for 1 ns (Tang et al., 2019). This equilibration stage allows for the determination of the competitive adsorption of oil and water on the surfaces, resulting in different wettability behaviors. After equilibration, water is removed and surfactants are placed on the left side of the system. The water in the simulation is controlled to redissolve the generated shale surface/oil/water/surfactant system. Finally, the shale surface/oil/water/surfactant system is simulated at 330 K for 1 ns, as illustrated in Figure 3. In this work, the time step for the simulations is 1 fs and periodic boundary conditions are adopted (Kubelka et al., 2021). The extended simple point charge (SPC/E) model is used for characterizing water molecules (Taylor et al., 1996). All molecular dynamics simulations in this study are performed on the Large-scale Atomic/Molecular Massively Parallel Simulator (LAMMPS) (Plimpton, 1995). The simulation results are visualized using OVITO software.
3 Results and discussion
3.1 Model validation
In this study, the contact angles of water droplets on the kaolinite and graphite surfaces are first simulated to validate the accuracy of the physical model and force field, as displayed in Figure 4. The simulation results demonstrate that the droplet spreads on the kaolinite surface, forming an incomplete monomolecular layer with a contact angle close to zero. This indicates an extremely hydrophilic nature of kaolinite surface, which is consistent with the results obtained by Šolc et al. (Šolc et al., 2011) The simulated contact angle of water droplet on the graphene surface is 94.2°, which is in good agreement with the simulation result of 99.1° obtained by Xiong et al. (Xiong et al., 2022) In this work, the contact angles of droplets are determined by:
where θ denotes the wetting contact angle, h is the droplet height, r is the radius of the circular contact surface between the droplet and the surface, and R is the radius of the droplet.
3.2 Effect of surfactants on the wettability of inorganic kaolinite surfaces
In order to examine the effects of different types and concentrations of surfactants on the wettability of n-hexane oil droplets on a kaolinite surface, a series of MD simulations are performed. Specifically, two sets surfactants, namely, SDBS-1/DTAB-1 (consisting of 25 surfactant molecules) and SDBS-2/DTAB-2 (consisting of 40 surfactant molecules) are examined.
3.2.1 Comparison of contact angle
To quantitatively describe the contact angles of oil droplets composed of 120 n-hexane molecules on the shale surface, we assume that the oil mass in equilibrium is a part of an ideal sphere. It should be noted that the mentioned contact angles hereinafter are measured with oil droplet on different surfaces. Figure 5 presents the variations in contact angle for the kaolinite/oil/water/surfactant system with different concentrations of SDBS and DTAB. In the SDBS-1 model group, the contact angle of n-hexane oil droplets increases from 84.55° to 94.09° after 1 ns of simulation calculations, while 93.92° for SDBS-2 system. The contact angle increase from 84.55° to 93.54° in DTAB-1 system, while 93.75° for DTAB-2 system. It is concluded that wettability alteration from intermediate-oleophilic to intermediate-oleophobic can be observed for both SDBS and DTAB. However, the concentration of surfactants indicates slight influences on the degree of wettability alteration.
3.2.2 Comparison of centroid ordinates
The vertical distance between the centroid of the oil droplet and kaolinite surface, i.e., the centroid ordinates of the oil droplet, is calculated by the COM (centre of mass) command in LAMMPS, and the centroid ordinates of the oil droplets in the Z direction are recorded every 2000 steps to observe the trend of oil droplets. Figure 6 shows the comparison of centroid ordinate differences in kaolinite system. The rise in the centroid ordinates indicates that the oil droplets have a tendency to move upwards away from the kaolinite surface due to the action of the surfactant. After 1 ns of simulation, the centroid ordinates of n-hexane oil droplets in SDBS-1 system increases by 1.15 Å, while 1.09 Å for SDBS-2 system. The COM trajectory of DTAB-1 system with the cationic surfactant also shows that the volume of oil droplet increases after the addition of DTAB, and the centroid ordinate in the Z direction rises by 0.84 Å. The centroid ordinates increase by 0.98 Å in the DTAB-2 system, as can be seen in Figure 7. It can be observed that n-hexane aggregates move much faster in the Z direction due to the interaction with SDBS. However, this effect decreases with the increasing concentration. Under the action of DTAB, the aggregates move faster with higher concentrations.
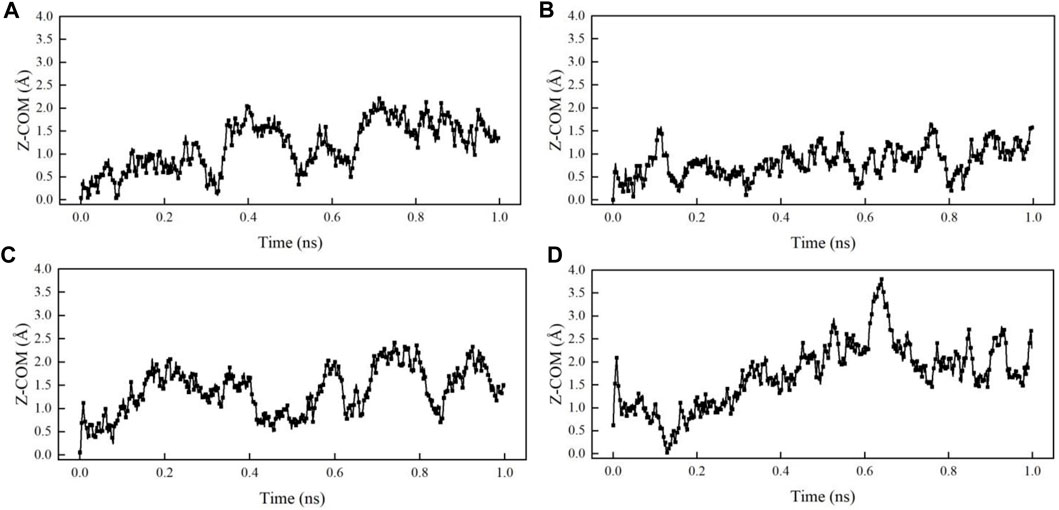
FIGURE 6. Variations of centroid ordinates in different systems: (A) SDBS-1; (B) SDBS-2; (C) DTAB-1; (D) DTAB-2.
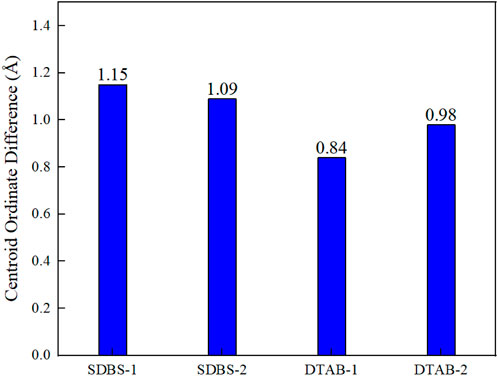
FIGURE 7. Comparison of centroid ordinate differences in different kaolinite systems with different surfactants.
3.2.3 Comparison of self-diffusion coefficients
The self-diffusion coefficient can fully reflect the diffusion and migration ability of n-hexane molecules on the shale surface. The self-diffusion coefficient of n-hexane molecules needs to be calculated to describe their movement and changes on the shale surface. The calculation formula can be written as:
where D is the self-diffusion coefficient of the n-hexane molecule,
Figure 8 shows the comparison of self-diffusion coefficients of the oil droplets. The self-diffusion coefficient of oil droplets in the SDBS-1 and SDBS-2 model are 8.61 × 10−9 and 7.11 × 10−9 m2
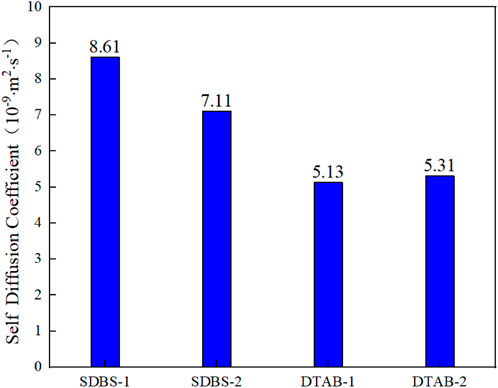
FIGURE 8. Comparison of self-diffusion coefficients in different kaolinite systems with different surfactants.
The magnitudes of contact angle and self-diffusion coefficient and the centroid position variation of the oil droplets clearly indicate the different effects of different surfactants on the oil adsorption onto the kaolinite surface. According to the simulation results, SDBS has better oil displacement performance than DTAB. This difference in oil displacement performance of the two surfactants was attributed to the head group effect. The groups in SDBS have a stronger attraction for water molecules. As a result, the SDBS migrates faster over the oil aggregation surface, and the oil molecule deformation separation is faster.
Although the data are somewhat scattered, a clear correlation between the wettability index and surfactant concentration can be observed. At higher concentrations, SDBS has less effect on the wettability variation of oil droplets on the kaolinite surface and less wettability inversion effect. Meanwhile, DTAB shows the opposite trend. Therefore, the effect of DTAB is opposite to that of SDBS. At higher concentrations, DTAB has a better effect on the wettability variation of oil droplets on the kaolinite surface and a better wettability inversion effect.
3.3 Effect of surfactants on the wettability of graphene surfaces
In the section, the effects of surfactant on the wettability on the simplified graphene surfaces are focused. We develop the system models containing 25 surfactant molecules, denoted as SDBS-1 and DTAB-1, respectively. After 1 ns of simulation, the contact angle of n-hexane oil droplets in SDBS-1 increases from 80.22° to 91.02°, while that in DTAB-1 increases to 89.96°, as shown in Figure 9. The vertical distances between the centroid of the oil droplet and the graphene surface, i.e., the centroid ordinates of the oil droplet, are recorded every 2000 steps to observe the trend of the oil droplets, as shown in Figure 10.
After 1 ns of simulation calculation, the centroid ordinate of n-hexane oil droplets rises by 1.14 Å in SDBS-1 and 0.86 Å in DTSB-1, as shown in Figure 11. The diffusion coefficient can fully reflect the diffusion and migration ability of n-hexane molecules on the shale surface. The diffusion coefficient of n-hexane molecules needs to be calculated to describe their movement and changes on the shale surface. As shown in Figure 12, the diffusion coefficient is 6.91 × 10−9 m2
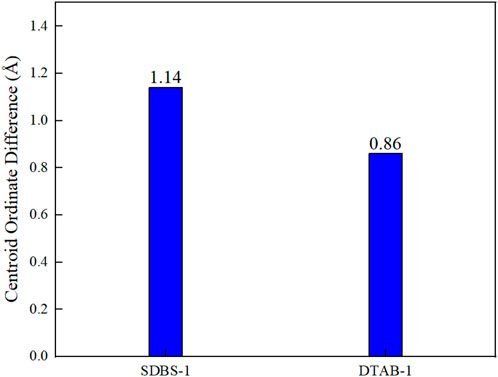
FIGURE 11. Comparison of centroid ordinate variations in different graphene systems with different surfactants.
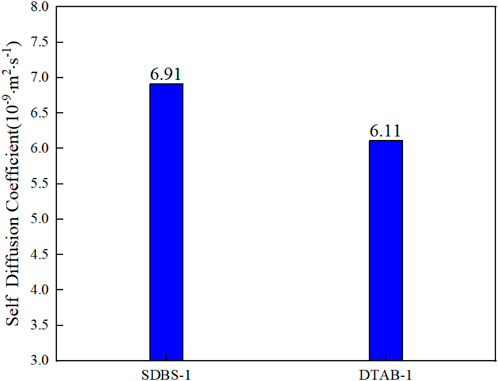
FIGURE 12. Comparison of self-diffusion coefficients in different graphene systems with different surfactants.
3.4 Effect of surfactants on the wettability of kerogen surfaces
To examine the effects of surfactant on wettability alteration of organic matter in shale reservoirs, a realistic kerogen surface is also developed. Different surfactant concentrations of SDBS-1 and DTAB-1 to SDBS-5 and DTAB-5 (20, 25, 30, 35, 40 surfactant molecules) are further designed.
3.4.1 Comparison of contact angles
Figure 13 shows the contact angle variation of the kerogen/oil/water/surfactant system with different concentrations of SDBS or DTAB. Without surfactant, the contact angle of the equilibrated oil droplet is 104.05°on the kerogen surface. With the increase of SDBS concentration, the contact angle of oil droplet increases from 114.91° to 119.67° and then shows a slight decrease to 118.87°. While for DTAB, a similar tendency is observed, with the contact angle increasing from 117.73° to 122.58° and declining to 110.23°. It indicates that there is an optimal concentration of surfactant for wettability alteration. It can be observed from Figure 13 that with 25 surfactant molecules, the interaction between DTAB and the oil/water interface is more intense, and the contact angle of n-hexane oil droplets changes more rapidly. While for SDBS, the contact angle changes more drastically with 35 surfactant molecules.
3.4.2 Comparison of centroid ordinates
Figure 14 shows the comparison of centroid ordinate differences in all kerogen systems. After 1 ns of calculation, the centroid ordinates of oil droplets in the system with SDBS increase by 0.98, 1.09, 1.01, 1.48 and 1.37 Å, respectively. The trajectory of the DTAB system also shows that the centroid ordinates in the Z direction increase by 1.46, 1.64, 1.31, 1.21 and 1.33 Å, respectively. Figure 15 shows a comparison of oil droplet centroid ordinate variations along the vertical direction. It is observed that the droplet move much faster in Z direction due to the interaction with DTAB. It is clear from the figure that there are optimal concentrations for both SDBS and DTAB in altering wettability of kerogen surface. The variation pattern of oil droplet centroid is consistent with that of contact angle.
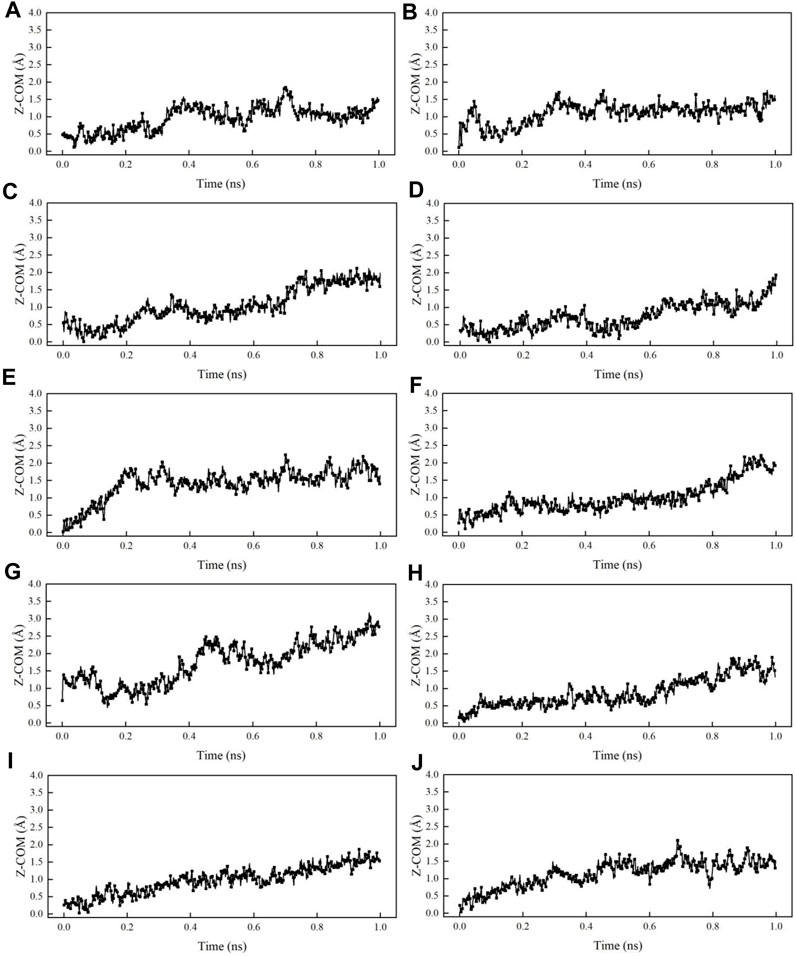
FIGURE 14. Variations of centroid ordinates in different systems: (A) SDBS-1; (B) SDBS-2; (C) SDBS-3; (D) SDBS-4; (E) SDBS-5; (F) DTAB-1; (G) DTAB-2; (H) DTAB-3; (I) DTAB-4; (J) DTAB-5.
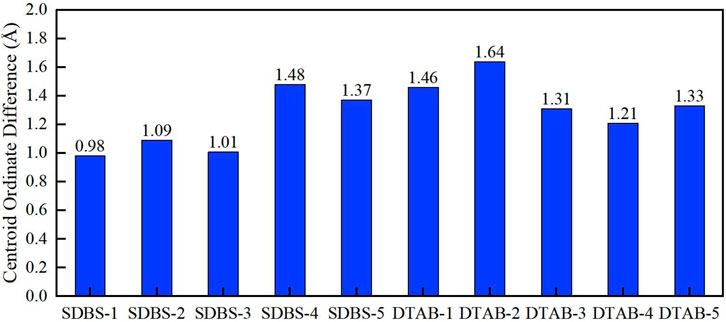
FIGURE 15. Comparison of centroid ordinate differences in different kerogen systems with different surfactants.
3.4.3 Comparison of self-diffusion coefficients
Figure 16 shows the comparison of self-diffusion coefficients of oil droplets. It is found that the self-diffusion coefficients of oil molecules increases and then decreases with the increment of the concentrations of SDBS and DTAB, which are consistent with earlier findings. It is observed that the effects of surfactant on the wettability of organic kerogen surfaces is significantly different from that on inorganic kaolinite surfaces. The difference is due to the different nature of the substrate. Different types of surfactants have different effects on the solution surface/interfacial tension. Surfactants significantly decrease the interfacial tension, but the extents of the decreases vary. As a water-bearing aluminosilicate, kaolinite is a clay mineral. Kerogen is a dispersed organic matter in sedimentary rocks insoluble in alkalis, non-oxidizing acids, and organic solvents. The differences in the structure and properties of kaolinite and kerogen lead to different effects of surfactants on their surfaces.
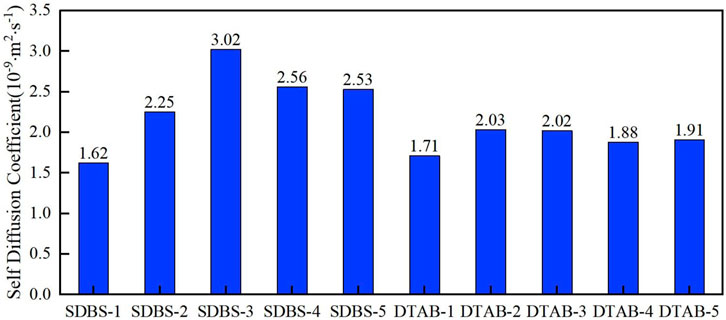
FIGURE 16. Comparison of self-diffusion coefficients in different kerogen systems with different surfactants.
4 Conclusion
In the present work, molecular dynamics simulations have been adopted to investigate the effects of surfactant on wettability alterations on kaolinite, graphene, and kerogen surfaces in shale reservoirs. The following conclusions can be drawn.
(1) The changes of contact angle, centroid ordinates, and self-diffusion coefficients in the simulations indicate that surfactants can alter the wettability of oil droplets on the surfaces of kaolinite, graphene, and kerogen.
(2) For oil-wet kaolinite surfaces with contact angle of 84.55°, the wettability reversals have been observed for both SDBS and DTAB. However, compared with graphene and kerogen surfaces, the degree of wettability alteration is less sensitive to surfactant concentration.
(3) For organic graphene and kerogen surfaces, surfactants are found to be able to further improve the contact angels of oil droplets. However, for kerogen surfaces, there is an optimal surfactant concentration for both SDBS and DTAB, beyond which the further addition of surfactant could decrease the contact angel.
(4) The obtained results suggest that the effects of SDBS and DTAB on the surfaces of kaolinite, graphene, and kerogen are obviously different, which is due to the interactions between headgroups of surfactants and surfaces.
Data availability statement
The original contributions presented in the study are included in the article/Supplementary Material, further inquiries can be directed to the corresponding authors.
Author contributions
DK: Funding acquisition, Investigation, Project administration, Validation, Writing–review and editing. XM: Conceptualization, Data curation, Validation, Writing–review and editing. JZ: Data curation, Investigation, Visualization, Writing–original draft, WZ: Validation, Visualization, Writing–original draft, Writing–review and editing.
Acknowledgments
The authors acknowledge the financial support from the Natural Sciences for Youth Foundation of China (No. 42102163), the Fundamental Research Funds of the Central Universities (No. FRF-TP-20-006A1) and the Open Fund of State Key Laboratory of Shale Oil and Gas Enrichment Mechanisms and Effective Development (No. 33550000-21-FW2099-0166).
Conflict of interest
The authors declare that the research was conducted in the absence of any commercial or financial relationships that could be construed as a potential conflict of interest.
Publisher’s note
All claims expressed in this article are solely those of the authors and do not necessarily represent those of their affiliated organizations, or those of the publisher, the editors and the reviewers. Any product that may be evaluated in this article, or claim that may be made by its manufacturer, is not guaranteed or endorsed by the publisher.
References
Afekare, D., Garno, J., and Rao, D. (2021). Enhancing oil recovery using silica nanoparticles: nanoscale wettability alteration effects and implications for shale oil recovery. J. Petrol. Sci. Eng. 203, 108897. doi:10.1016/j.petrol.2021.108897
Al Mahrouqi, D., Vinogradov, J., and Jackson, M. D. (2017). Zeta potential of artificial and natural calcite in aqueous solution. Adv. Colloid Interface Sci. 240, 60–76. doi:10.1016/j.cis.2016.12.006
Bai, S., Kubelka, J., and Piri, M. (2020). Relationship between molecular charge distribution and wettability reversal efficiency of cationic surfactants on calcite surfaces. J. Mol. Liq. 318, 114009. doi:10.1016/j.molliq.2020.114009
Chai, J., Liu, S., and Yang, X. (2009). Molecular dynamics simulation of wetting on modified amorphous silica surface. Appl. Surf. Sci. 255, 9078–9084. doi:10.1016/j.apsusc.2009.06.109
Chen, C., Zhang, N., Li, W., and Song, Y. (2015). Water contact angle dependence with hydroxyl functional groups on silica surfaces under CO2 sequestration conditions. Environ. Sci. Technol. 49, 14680–14687. doi:10.1021/acs.est.5b03646
Chen, Y.-J., Xu, G.-Y., Yuan, S.-L., and Sun, H.-Y. (2006). Molecular dynamics simulations of AOT at isooctane/water interface. Colloid Surf. A 273, 174–178. doi:10.1016/j.colsurfa.2005.08.031
Chen, Z., Sun, J., Wu, P., Liu, W., Chen, C., Lang, C., et al. (2023). Cyclodextrin as a green anti-agglomerant agent in oil–water emulsion containing asphalt. Fuel 335, 127041. doi:10.1016/j.fuel.2022.127041
Cui, F., Jin, X., Liu, H., Wu, H., and Wang, F. (2022). Molecular modeling on Gulong shale oil and wettability of reservoir matrix. Capillarity 5 (4), 65–74. doi:10.46690/capi.2022.04.01
Dang, W., Nie, H., Zhang, J., Tang, X., Jiang, S., Wei, X., et al. (2022). Pore-scale mechanisms and characterization of light oil storage in shale nanopores: new method and insights. Geosci. Front. 13, 101424. doi:10.1016/j.gsf.2022.101424
Deng, X., Kamal, M. S., Patil, S., Hussain, S. M. S., and Zhou, X. (2020). A review on wettability alteration in carbonate rocks: wettability modifiers. Energy fuels. 34, 31–54. doi:10.1021/acs.energyfuels.9b03409
Guo, H., He, M., Sun, C., Li, B., and Zhang, F. (2012). Hydrophilic and strength-softening characteristics of calcareous shale in deep mines. J. Rock Mech. Geotech. Eng. 4, 344–351. doi:10.3724/sp.j.1235.2012.00344
Guo, Q., Yao, Y., Hou, L., Tang, S., Pan, S., and Yang, F. (2022). Oil migration, retention, and differential accumulation in “sandwiched” lacustrine shale oil systems from the Chang 7 member of the Upper Triassic Yanchang Formation, Ordos Basin, China. Int. J. Coal Geol. 261, 104077. doi:10.1016/j.coal.2022.104077
Jagadisan, A., and Heidari, Z. (2022). Molecular dynamic simulation of the impact of thermal maturity and reservoir temperature on the contact angle and wettability of kerogen. Fuel 309, 122039. doi:10.1016/j.fuel.2021.122039
Kubelka, J., Bai, S., and Piri, M. (2021). Effects of surfactant charge and molecular structure on wettability alteration of calcite: insights from molecular dynamics simulations. J. Phys. Chem. B 125, 1293–1305. doi:10.1021/acs.jpcb.0c10361
Larestani, A., Mousavi, S. P., Hadavimoghaddam, F., Ostadhassan, M., and Hemmati-Sarapardeh, A. (2022). Predicting the surfactant-polymer flooding performance in chemical enhanced oil recovery: cascade neural network and gradient boosting decision tree. Alexandria Eng. J. 61, 7715–7731. doi:10.1016/j.aej.2022.01.023
Liu, J., Sheng, J. J., and Huang, W. (2019b). Experimental investigation of microscopic mechanisms of surfactant-enhanced spontaneous imbibition in shale cores. Energy fuels. 33, 7188–7199. doi:10.1021/acs.energyfuels.9b01324
Liu, J., Sheng, J. J., Wang, X., Ge, H., and Yao, E. (2019a). Experimental study of wettability alteration and spontaneous imbibition in Chinese shale oil reservoirs using anionic and nonionic surfactants. J. Petrol. Sci. Eng. 175, 624–633. doi:10.1016/j.petrol.2019.01.003
Liu, X., Kang, Y., Yan, L., Tian, J., Li, J., and You, L. (2022). Implication of interfacial tension reduction and wettability alteration by surfactant on enhanced oil recovery in tight oil reservoirs. Energy Rep. 8, 13672–13681. doi:10.1016/j.egyr.2022.10.052
Michalec, L., and Lísal, M. (2017). Molecular simulation of shale gas adsorption onto overmature type II model kerogen with control microporosity. Mol. Phys. 115, 1086–1103. doi:10.1080/00268976.2016.1243739
Mirchi, V., Saraji, S., Goual, L., and Piri, M. (2015). Dynamic interfacial tension and wettability of shale in the presence of surfactants at reservoir conditions. Fuel 148, 127–138. doi:10.1016/j.fuel.2015.01.077
Okunade, O. A., Yekeen, N., Padmanabhan, E., Al-Yaseri, A., Idris, A. K., and Khan, J. A. (2021). Shale core wettability alteration, foam and emulsion stabilization by surfactant: impact of surfactant concentration, rock surface roughness and nanoparticles. J. Petrol. Sci. Eng. 207, 109139. doi:10.1016/j.petrol.2021.109139
Pei, H., Zhang, G., Ge, J., Tang, M., and Zheng, Y. (2012). Comparative effectiveness of alkaline flooding and alkaline–surfactant flooding for improved heavy-oil recovery. Energy fuels. 26, 2911–2919. doi:10.1021/ef300206u
Plimpton, S. (1995). Fast Parallel algorithms for short-range molecular dynamics. J. Comput. Phys. 117, 1–19. doi:10.1006/jcph.1995.1039
Qin, C., Jiang, Y., Zhou, J., Zuo, S., Chen, S., Liu, Z., et al. (2022). Influence of supercritical CO2 exposure on water wettability of shale: implications for co2 sequestration and shale gas recovery. Energy 242, 122551. doi:10.1016/j.energy.2021.122551
Rezaei, N., Zendehboudi, S., Chatzis, I., and Lohi, A. (2018). Combined benefits of capillary barrier and injection pressure control to improve fluid recovery at breakthrough upon gas injection: an experimental study. Fuel 211, 638–648. doi:10.1016/j.fuel.2017.09.048
Roshan, H., Al-Yaseri, A. Z., Sarmadivaleh, M., and Iglauer, S. (2016). On wettability of shale rocks. J. Colloid Interface Sci. 475, 104–111. doi:10.1016/j.jcis.2016.04.041
SalahEldin Hussien, O., Elraies, K. A., Almansour, A., Husin, H., Belhaj, A., and Ern, L. (2019). Experimental study on the use of surfactant as a fracking fluid additive for improving shale gas productivity. J. Petrol. Sci. Eng. 183, 106426. doi:10.1016/j.petrol.2019.106426
Shi, K., Chen, J., Pang, X., Jiang, F., Hui, S., Pang, H., et al. (2022). Effect of wettability of shale on CO2 sequestration with enhanced gas recovery in shale reservoir: implications from molecular dynamics simulation. J. Nat. Gas. Sci. Eng. 107, 104798. doi:10.1016/j.jngse.2022.104798
Šolc, R., Gerzabek, M. H., Lischka, H., and Tunega, D. (2011). Wettability of kaolinite (001) surfaces — molecular dynamic study. Geoderma 169, 47–54. doi:10.1016/j.geoderma.2011.02.004
Stankiewicz, A., Bennett, B., Wint, O., Ionkina, N., Motherwell, B., and Mastalerz, M. (2015). “Kerogen density revisited – lessons from the duvernay shale,” in Unconventional Resources Technology Conference, San Antonio, Texas, 20-22 July 2015, 864–874. doi:10.15530/urtec-2015-2157904
Su, S., Jiang, Z., Shan, X., Zhu, Y., Wang, P., Luo, X., et al. (2018). The wettability of shale by NMR measurements and its controlling factors. J. Petrol. Sci. Eng. 169, 309–316. doi:10.1016/j.petrol.2018.05.067
Sun, C., Nie, H., Dang, W., Chen, Q., Zhang, G., Li, W., et al. (2021a). Shale gas exploration and development in China: current status, geological challenges, and future directions. Energy fuels. 35, 6359–6379. doi:10.1021/acs.energyfuels.0c04131
Sun, H.-m., Yang, W., Chen, R.-p., and Kang, X. (2021c). Microfabric characteristics of kaolinite flocculates and aggregates — insights from large-scale molecular dynamics simulations. Appl. Clay Sci. 206, 106073. doi:10.1016/j.clay.2021.106073
Sun, H., Mumby, S. J., Maple, J. R., and Hagler, A. T. (1994). An ab initio CFF93 all-atom force field for polycarbonates. J. Am. Chem. Soc. 116, 2978–2987. doi:10.1021/ja00086a030
Sun, J., Chen, Z., Wang, X., Zhang, Y., Qin, Y., Chen, C., et al. (2023). Displacement characteristics of CO2 to CH4 in heterogeneous surface slit pores. Energy fuels. 37, 2926–2944. doi:10.1021/acs.energyfuels.2c03610
Sun, Y.-P., Xin, Y., Lyu, F.-T., and Dai, C.-L. (2021b). Experimental study on the mechanism of adsorption-improved imbibition in oil-wet tight sandstone by a nonionic surfactant for enhanced oil recovery. Pet. Sci. 18, 1115–1126. doi:10.1016/j.petsci.2021.07.005
Tang, L., Song, Y., Jiang, S., Li, L., Li, Z., Li, Q., et al. (2020). Sealing mechanism of the roof and floor for the wufeng-longmaxi shale gas in the southern sichuan basin. Energy fuels. 34, 6999–7018. doi:10.1021/acs.energyfuels.0c00983
Tang, X., Xiao, S., Lei, Q., Yuan, L., Peng, B., He, L., et al. (2019). Molecular dynamics simulation of surfactant flooding driven oil-detachment in nano-silica channels. J. Phys. Chem. B 123, 277–288. doi:10.1021/acs.jpcb.8b09777
Taylor, R. S., Dang, L. X., and Garrett, B. C. (1996). Molecular dynamics simulations of the liquid/vapor interface of SPC/E water. J. Phys. Chem. 100, 11720–11725. doi:10.1021/jp960615b
Tenney, C. M., and Cygan, R. T. (2014). Molecular simulation of carbon dioxide, brine, and clay mineral interactions and determination of contact angles. Environ. Sci. Technol. 48, 2035–2042. doi:10.1021/es404075k
Xiong, J., Tang, J., Zhou, X., Liu, X., Liang, L., and Hou, L. (2022). Molecular dynamics simulation and experimental studies of the wettability behaviors of shales. Energy fuels. 36, 3526–3538. doi:10.1021/acs.energyfuels.1c04346
Yang, N., Yuan, R., Li, W., Tan, X., Liu, Z., Zhang, Q., et al. (2023). Magnetic-driving giant multilayer polyelectrolyte microcapsules for intelligent enhanced oil recovery. Colloid Surf. A 664, 131107. doi:10.1016/j.colsurfa.2023.131107
Yao, Y., Wei, M., and Kang, W. (2021). A review of wettability alteration using surfactants in carbonate reservoirs. Adv. Colloid Interface Sci. 294, 102477. doi:10.1016/j.cis.2021.102477
Yu, K. B., Bowers, G. M., Loganathan, N., Kalinichev, A. G., and Yazaydin, A. O. (2021). Diffusion behavior of methane in 3D kerogen models. Energy fuels. 35, 16515–16526. doi:10.1021/acs.energyfuels.1c02167
Zhang, L., Liao, H., and Cui, M. (2022). Effect of CO2 flooding in an oil reservoir with strong bottom-water drive in the Tahe Oilfield, Tarim Basin, Northwest China. Energy Geosci., 100127. doi:10.1016/j.engeos.2022.08.004
Zhang, W., Huang, Z., Li, X., Chen, J., Guo, X., Pan, Y., et al. (2020). Estimation of organic and inorganic porosity in shale by NMR method, insights from marine shales with different maturities. J. Nat. Gas. Sci. Eng. 78, 103290. doi:10.1016/j.jngse.2020.103290
Zhao, M., Guo, X., Wu, Y., Dai, C., Gao, M., Yan, R., et al. (2021). Development, performance evaluation and enhanced oil recovery regulations of a zwitterionic viscoelastic surfactant fracturing-flooding system. Colloid Surf. A 630, 127568. doi:10.1016/j.colsurfa.2021.127568
Zhao, Y., and Jin, Z. (2021). Hydrocarbon mixture phase behavior in multi-scale systems in relation to shale oil recovery: the effect of pore size distributions. Fuel 291, 120141. doi:10.1016/j.fuel.2021.120141
Zheng, J., Feng, G., Ren, Z., Qi, N., Coffman, D. M., Zhou, Y., et al. (2022). China's energy consumption and economic activity at the regional level. Energy 259, 124948. doi:10.1016/j.energy.2022.124948
Zhou, W., Jiang, L., Liu, X., Hu, Y., and Yan, Y. (2022). Molecular insights into the effect of anionic-nonionic and cationic surfactant mixtures on interfacial properties of oil-water interface. Colloid Surf. A 637, 128259. doi:10.1016/j.colsurfa.2022.128259
Zhou, W., Wang, H., Yan, Y., and Liu, X. (2019a). Adsorption mechanism of CO2/CH4 in kaolinite clay: insight from molecular simulation. Energy & Fuels 33, 6542–6551. doi:10.1021/acs.energyfuels.9b00539
Zhou, W., Wang, H., Yang, X., Liu, X., and Yan, Y. (2020). Confinement effects and CO2/CH4 competitive adsorption in realistic shale kerogen nanopores. Ind. Eng. Chem. Res. 59, 6696–6706. doi:10.1021/acs.iecr.9b06549
Zhou, W., Wang, H., Zhang, Z., Chen, H., and Liu, X. (2019b). Molecular simulation of CO2/CH4/H2O competitive adsorption and diffusion in brown coal. RSC Adv. 9, 3004–3011. doi:10.1039/c8ra10243k
Keywords: shale oil, surfactant, wettability alteration, enhanced oil recovery, molecular dynamics simulation
Citation: Kong D, Meng X, Zhu J and Zhou W (2023) Molecular dynamics simulation of surfactant induced wettability alteration of shale reservoirs. Front. Energy Res. 11:1272132. doi: 10.3389/fenrg.2023.1272132
Received: 03 August 2023; Accepted: 24 August 2023;
Published: 05 September 2023.
Edited by:
Peng Tan, CNPC Engineering Technology R&D Company Limited, ChinaReviewed by:
Qitao Zhang, The Pennsylvania State University (PSU), United StatesYi Zhang, Dalian University of Technology, China
Copyright © 2023 Kong, Meng, Zhu and Zhou. This is an open-access article distributed under the terms of the Creative Commons Attribution License (CC BY). The use, distribution or reproduction in other forums is permitted, provided the original author(s) and the copyright owner(s) are credited and that the original publication in this journal is cited, in accordance with accepted academic practice. No use, distribution or reproduction is permitted which does not comply with these terms.
*Correspondence: Debin Kong, a29uZ2RiQHVzdGIuZWR1LmNu; Wenning Zhou, d2VubmluZ3pob3VAdXN0Yi5lZHUuY24=