- Department of Biotechnology, Indira Gandhi National Tribal University, Amarkantak, Madhya Pradesh, India
The present study demonstrates the potential of freshwater algal species collected from the natural habitats of Central India for biofuel production. Algal samples were collected from different waterbodies and were microscopically examined in the laboratory. Based on the morphological features using the taxonomic keys, a total of 51 algal species (18 Cyanophyta, 22 Chlorophyta, 7 Bacillariophyta, and 4 Charophyta) were identified. Among them, 18 algal species were successfully purified in the laboratory and tested for their ability to produce biomass and lipid, which are the essential criteria to be qualified as suitable feedstock for biofuel production. The data on specific growth rates suggest that Chlorella vulgaris, Scenedesmus bijugatus, and Nitzschia recta were the fastest growing species, whereas all the members of Cyanophyta showed relatively slower growth. The biomass productivity was higher in C. vulgaris, S. bijugatus, and Anabaena sp., but the lipid production was highest in C. vulgaris followed by Haematococcus sp., whereas the members of Cyanophyta showed the lowest lipid production. Furthermore, we assessed the ability of biomass and lipid production and biochemical parameters of four selected algal species, C. vulgaris, S. bijugatus, Anabaena sp., and N. recta, due to their faster growth, higher biomass and lipid production, and abundance. Based on the cluster analyses of the values of lipid and biomass production of these species against internal N:P ratio, three distinct clusters were noticed, cluster 1: near 50:1, cluster 2: between 30:35, and cluster 3 between 15:20 cellular N:P ratios. High lipid and biomass productivity were observed between the 30:35 N:P ratio. Despite the metabolic significance of N and P for algae, more research is warranted to conclude anything precisely.
1 Introduction
The global energy demand is largely met by fossil-based non-renewable resources. The rapidly depleting fossil-based energy resources and the pressing need to reduce carbon emissions have greatly boosted the use of renewable forms of energy resources. During the past few years, algae have attracted the attention of researchers as a potential resource for biofuel production. The use of algae as a potential feedstock for biofuel production has been proven to be more sustainable and ecologically and socially relevant. Compared to first- and second-generation biofuels based on edible crops and higher plants, algae, being the third-generation biofuel, produce more lipids and biomass without compromising the arable land and, therefore, do not affect the production of food and fodder (Andrew et al., 2022). A great deal of research has already been conducted on various aspects of algal biofuel, but algal biofuel production still remains a highly expensive and rigorous process (Mohsenpour et al., 2021). With the estimated cost of USD 300–2600 per barrel algal biofuel compared to USD 40–80 per barrel petroleum (Hannon et al., 2010), the current strategies for producing algal biofuel are economically not viable and, hence, restrict the algal biofuel being used as transportation fuel.
Developing superior species with the capability of producing high biomass and lipids, designing effective harvesting techniques, and simultaneously extracting multiple compounds of commercial importance can make the algal biofuel production process economically viable (Hussain and Rittmann, 2023). Algal species suitable for biofuel production can be isolated from the nature or developed in the laboratory using genetic manipulation techniques. Genetic modification techniques can be used to develop superior algal species for biofuel production. Researchers at the California Center for Algae Biotechnology at UCSD are using genetic engineering to design algae that grow faster and accumulate more lipids, making them more efficient and cost effective (Martin et al., 2020). Bioengineers forecast that microalgae will be redesigned to produce biofuels using insights from synthetic biology, an advanced method of creating genetically engineered organisms (Snow and Smith, 2012). Synthetic biology can be used to improve the efficiency of algal biofuel production. Synthetic biology is an interdisciplinary approach combining biotechnology, evolutionary biology, molecular biology, systems biology, and biophysics. Its focus on the design and construction of biological devices that perform useful functions is clear and of great utility to engineering algae. This relies on the re-engineering of biological circuits and optimization of certain metabolic pathways to reprogram algae and introduce new functions in them via the use of genetic modules (Khraiwesh et al., 2015).
However, the use of synthetic biology in algal biofuel production is still in its infancy and challenging due to the non-availability of advanced genetic tools (Jagadevan et al., 2018). The major obstacles in genetic and metabolic engineering of algae have been the lack of suitable transformation vectors to direct the engineered gene product to the specific sub-cellular location and inability to robustly express multiple nuclear-encoded transgenes within a single cell (Rasala et al., 2014). Moreover, biocontainment strategies are under development to reduce the probability that genetically engineered algae can survive outside the laboratory or industrial setting. In addition, the requirement for sophisticated infrastructure makes this strategy cumbersome for developing nations with limited resources. Given these limitations, we postulated that naturally occurring algal species could be more straightforward solutions to various challenges. Therefore, screening suitable algal species from nature is a more appropriate and feasible strategy better suited for developing nations. Although a great deal of information is available about using freshwater algal species for biofuel production, few studies have been conducted to screen freshwater algal species for testing their efficiency for biofuel production (Mutanda et al., 2011; Pandey et al., 2019; Sanathkumaran et al., 2019). However, most of these studies were restricted to particular or specific habitats or focused on a specific group or types of algal species. No serious attempt has been made for the comprehensive screening of freshwater algal species as suitable feedstock for biofuel production.
Considering the aforementioned points, the present study aims to screen freshwater algal species of Central India for their potential to be used as suitable feedstock for algal biofuel production. Central India, particularly Madhya Pradesh and Chhattisgarh, has numerous natural and man-made waterbodies that harbor a huge number of freshwater algal species. However, to the best of our knowledge, no effort has been made for the bioprospecting of these algal species for biofuel production.
2 Materials and methods
2.1 Collection of freshwater algal species
Various freshwater algal species were collected from the 200 KM periphery of the Indira Gandhi National Tribal University, Amarkantak, Madhya Pradesh. These species were primarily collected from aquatic and semi-aquatic habitats, e. g., lakes, ponds, fountains, water channels, and moist terrestrial and aerial habitats, e. g., rice fields and wet walls. The freshwater algal species were sampled during the rainy seasons from various habitats mentioned previously in Madhya Pradesh and Chhattisgarh. Central India (Madhya Pradesh and Chhattisgarh) is known for rich natural resources with less explored and undisturbed algal biodiversity. Hence, some highly suitable species for algal biofuel production can be found by exploring algal species in this area. Samples were collected using a plastic water bottle or polythene bag. For this purpose, about 100–200 mL of water was collected from each sampling site. However, algal species from terrestrial habitats were manually collected using forceps, and collected samples were kept in a Petri dish containing the appropriate volume of water. Zooplankton, if any present in the water samples, were killed by placing the samples at 4°C for 10–15 min. Usually, rotifers, cladocerans, copepods, and protozoans were found in the water samples, and incubating water samples at 4°C for 10–15 min effectively killed all of them.
2.2 Morphological identification of the algal species
The algal species in the collected samples were identified through their morphological examination using a digital inverted microscope (EVOS XL Core, Invitrogen, United States). For this purpose, glass slides of these samples were prepared, and algal species were identified based on their morphology using the study by Phillipose (1959), Desikachary (1955), and Bellinger and Sigee (2015), which describes the identifying features of chlorococcales, cyanophytes, and freshwater algae, respectively. The images of the algal species were mostly taken at 40x and saved in the JPG format for further analysis.
2.3 Isolation and purification of algal species
The collected samples usually possessed different algal species. Hence, after the morphological identification, algal species were isolated and purified using standard microbiological procedures involving serial dilution, plating, and streaking methods as elaborated by Guillard (2005) without any modifications. Initially, the algal samples were inoculated in 1/4th concentration of BG 11 algal culture media (Tripathi et al., 2003) and kept at standard conditions of light and temperature (Tripathi et al., 2004) for 5–7 days for the acclimatization of the algal species in the laboratory. Thereafter, the algal samples maintained in the BG 11 media were serially diluted and transferred to the Petri plates containing BG 11 media and agar-agar as a solidifying agent. A drop of dilute cell suspension was streaked on an agar plate. The plates were incubated in light and monitored regularly. After 6–7 days, small colonies appeared on the agar plates and were again observed microscopically, and pure colonies were picked up aseptically and transferred into culture flasks containing 100 mL of liquid algal culture medium (BG 11). The culture tubes were kept in the culture room at 72 µmol photons m−2·s−1 PAR in a 12:12 h light and dark cycle at 27°C, as mentioned by Tripathi et al. (2004). The pH of the culture medium was adjusted to 7.0.
2.4 Cultivation of purified algal species in the laboratory
After the purification of the algal species as specified previously, these species were cultivated in the laboratory for further experiments. The algal species were cultivated in appropriate algal culture medium, e. g. BG 11, BG 11 N−, and Allen and Arnon’s culture medium, depending on the type of algal species. The members of Chlorophyta were cultivated in BG 11 culture media, whereas for the cultivation of the members of Bacillariophyta, the BG 11 culture medium was supplemented with sodium silicate 2.3 mM. The members of Cyanophyta, especially nitrogen-fixing species, were either cultivated in nitrogen-deficient BG 11 (BG 11 N−) or in Allen and Arnon’s culture media. However, non-N2-fixing species of Cyanophyta were cultivated in a normal BG 11 culture medium. The composition of these culture media is given in Tables 1, 2. The pH of the culture medium was adjusted to 7.0 (Chlorophyta and Bacillariophyta) or 7.5 (Cyanophyta) by using NaOH/HCl (0.1 N), and the cultures were incubated at 27°C in an air-conditioned culture room and illuminated by 40 W fluorescent tubes (PAR 72 μmol·m−2·s−1) for 12 h daily. The cultures were hand shaken at least 3–4 times daily. However, shaking incubators are the better choice for continuous mixing, but hand shaking 3–4 times daily has also been effective for uniform mixing (Tripathi et al., 2004).
2.5 Measurement of the growth and biomass of the algal species
The growth of the algal species was expressed in terms of the specific growth rate. The specific growth rate was calculated using the following formula:
where N2 and N1 are the absorbance of the algal suspension at 663 nm at times t2 and t1, respectively.
For this purpose, a 3.0 mL culture suspension of the algal species was harvested at 0, 3, 6, 9, 12, 15, 18, and 21 days, and the absorbance of the suspension was recorded at 663 nm using a UV–Vis spectrophotometer (Shimadzu, Japan).
In addition to the specific growth rate, the biomass productivity of the algal species was also calculated. In order to determine the biomass of the algal species, a 10 mL culture suspension was harvested at different time intervals as mentioned previously in pre-weighed 15 mL falcon tubes, and the weight the falcon tubes containing 10 mL algal suspension was determined (fresh weight). Subsequently, the algal suspension was poured into a 25 mL crucible and oven dried at 70°C for 24–36 h, and the weight of the dried samples (dry weight) was again recorded. The volumetric biomass productivity was calculated using the following equation by Hempel et al. (2012):
Biomass productivity (mgL−1Day−1): (X2-X1)/(t2-t1), where X1 and X2 represent the biomass (dry weight) at the starting time point (t1) and endpoint of cultivation (t2), respectively.
2.6 Measurement of the photosynthetic pigments
The photosynthetic pigments (chlorophyll a and carotenoids) of the algal species were also determined. For the quantification of the photosynthetic pigments, a 5 mL algal suspension was harvested from exponentially growing algal cultures and centrifuged at 5,000 rpm for 10 min at room temperature. The algal pellets were resuspended in 80% acetone for 24 h at 4°C. Thereafter, the suspension was centrifuged, and the absorbance of the supernatant was recorded at 663, 645, and 480 nm for the quantitative estimation of chlorophyll a and carotenoids. Chlorophyll a content was calculated according to the work of Mackinney (1941) and carotenoids as per the work of Myers and Kratz (1955).
2.7 Extraction and estimation of total lipids
The extraction and estimation of lipids is a very tedious process; however, researchers have developed several methods for the extraction and analysis of lipids of algae. However, the gravimetric method given by Bligh and Dyer (1959) and the colorimetric, sulfo-phospho-vanillin (SPV) method (Anschau et al., 2017) have been proven effective in estimating lipids of the algal cell. Therefore, we used these two methods for the estimation of the total lipids of the algal species.
2.7.1 Gravimetric method of lipid estimation
A known volume (10 mL) of the algal culture was harvested by centrifugation at the end of the experimental period, and the pellet was washed 5–6 times in Milli-Q water and then extracted with a chloroform:methanol mixture (2:1; v/v) using the method given by Bligh and Dyer (1959). The extraction was carried out until a blue-colored residue was left. The extract was evaporated to dryness at 37°C. Subsequently, the weight of the lipid was measured using a pre-weighted beaker.
2.7.2 Colorimetric method for the determination of lipids (adapted from the SPV method)
800 µL algal suspension was mixed with 200 µL concentrated H2SO4 in a glass test tube and incubated at 100°C for 10 min. Subsequently, the mixer was cooled at room temperature, and thereafter, 0.5 mL of the phosphoric acid and vanillin (PV) reagent was added for the development of color. The PV reagent was prepared by dissolving 6.0 mg of vanillin in 100 mL of hot water. Furthermore, the aforementioned solution was diluted to 500 mL using 85% phosphoric acid. The algal suspension mixed with the PV reagent was heated at 37°C for 15 min and then cooled at room temperature and incubated in the dark for 45 min. Thereafter, the absorbance of the suspension was measured at 530 nm. The amount of the lipid was calculated using oleic acid as the standard.
2.7.3 Calculation of lipid productivity
The lipid productivity expressed in mgL−1day−1 was calculated using the following formula, as described by Sanathkumaran et al. (2019):
Lipid productivity = biomass productivity × TL/100, where TL is the total lipid content.
2.8 Estimation of total protein
The protein content of the algal species was measured quantitatively by using the method given by Lowry et al. (1951).
2.9 Determination of cellular N and P and calculation of N:P ratio
The residual phosphate content of the culture medium was estimated by the stannous chloride method (APHA, 1995). The residual nitrate of the culture medium was estimated colorimetrically by the brucine sulfuric acid method (Nicholas and Nason, 1957). The cellular N and P were calculated by using the following formula: initial nitrate or phosphate content-residual nitrate or phosphate content in the culture medium = total cellular N (nitrate) or P (phosphate)/total number of cells per ml.
2.10 Cluster and statistical analyses
Cluster and statistical analyses were performed using DATAtab (Datatab team, 2023). For cluster analyses, two metric variables, e. g., lipid productivity or biomass productivity and cellular N:P ratio, were used using a K-means cluster with 10 times repetition of analysis to get the best results. The start centroids were set randomly. For the cluster analysis, all the datasets of all four algal species were merged to get generalized information about algae. All the statistical analyses were performed at a significance level of <0.5. Regression analysis was performed using cellular N:P ratio as the independent variable and lipid or biomass productivity as the dependent variable.
3 Results
3.1 Collection, identification, purification, and cultivation of freshwater algal species
Based on the microscopic examination of the water samples collected from different waterbodies in Central India (parts of Madhya Pradesh and Chhattisgarh), more than 48 algal species were recorded. Among these species, we identified 41 algal species generally belonging to the three major divisions of the algae: Chlorophyta (green algae), Cyanophyta (blue–green algae), and Bacillariophyta (diatoms). A few members of Charophyta were also observed. However, no algae belonging to Rhodophyta or Pheophyta were observed. Table 1 shows the details of the algae collected from different waterbodies in Madhya Pradesh and Chhattisgarh. Photographs of these algal species are presented in photo plates 1–4.
Furthermore, Table 4 shows the list of algal species that were successfully purified and cultivated in our laboratory. Among these species, nine belong to Chlorophyta, five belong to Cyanophyta, two belong to Bacillariophyta, and two belong to Charophyta. Most of the members of Chlorophyta were successfully cultivated in a normal BG 11 culture medium, whereas Haematococcus sp. was grown in a BG 11 medium supplemented with borosilicate. On the other hand, members of Cyanophyta were cultivated in different culture media: Anbaena sp. and N. calcicola were grown in the BG 11 N− medium; A. doliolum was cultivated in Allen and Arnon’s culture medium; and M. aeruginosa and Lyngbya sp. were cultivated in the DP and BG 11 medium, respectively. Like Chlorophyta, the members of Charophyta were successfully cultivated in the BG 11 medium. The members of Bacillariophyta were cultivated in a BG 11 medium supplemented with borosilicate.
3.2 Assessment of the growth and biomass and lipid productivity of the purified algal species
Figure 1 shows the specific growth rates of 18 purified algal species cultivated in the laboratory. In general, the growth rate of Chlorophytes was higher than the that of Bacillariophytes and other groups. The growth rate of cyanophytes was relatively slower than that of others. The members of Charophyta showed either a moderate or higher growth rate. Among these species, the growth rate (µ day−1) of S. bijugatus was the highest (0.6), followed by C. vulgaris (0.58) and S. dimorphus (0.55). The growth rates of R. subcapitata, Closterium sp., and N. recta were almost equal (0.53). N. calcicola and Lyngbya sp. were found to be the slowest growing species, as evident from their growth rates.
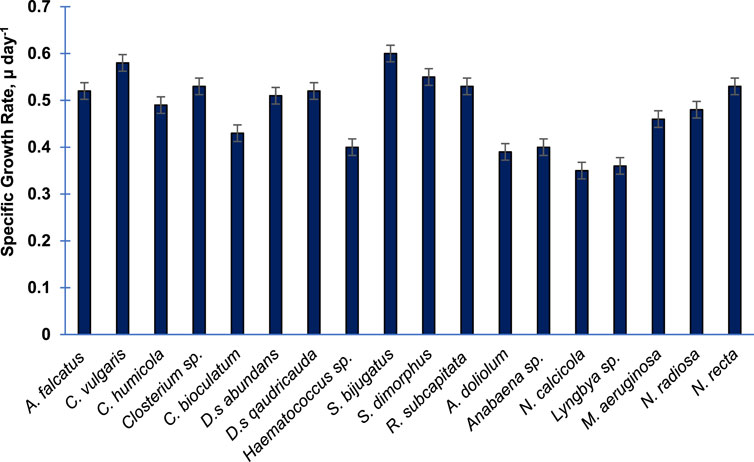
FIGURE 1. Specific growth rate of 18 purified algal species cultivated in the laboratory. The specific growth rate was calculated during the exponential phase of growth. Bars are means of triplicates ± S.D.
Figure 2 presents the biomass productivity of the 18 purified algal species. Unlike the specific growth rate, the biomass productivity has shown different trends. However, the two fastest growing algal species, namely, S. bijugatus and C. vulgaris, also showed the highest biomass productivity. Interestingly, the slow growing species of Cyanophyta produced remarkable biomass productivity, e. g., Anabaena sp. (7.9), A. doliolum (7.1), N. calcicola (6.8), and M. aeruginosa (6.7 mgL−1Day−1).
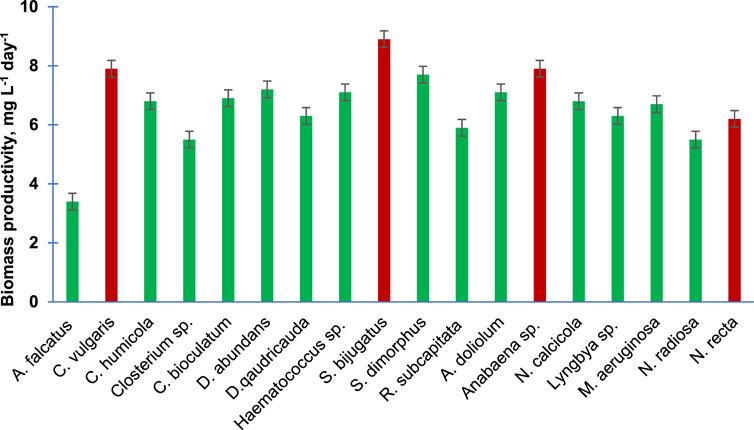
FIGURE 2. Biomass productivity of the purified algal species cultivated in the laboratory. Bars are means of triplicates ± S.D.
Figure 3 shows the lipid productivity of 18 purified algal species. C. vulgaris and Haematococcus sp. produced 2.41 and 2.31 mg lipid L−1day−1 followed by S. bijugatus and S. dimorphus, which produced 1.71 and 1.62 mg lipid L−1day−1, respectively. The lipid productivity was lowest in the members of the Cyanophyta. The lipid productivity of the members of Bacillariophyta and Charophyta was relatively lower than the average lipid productivity of chlorophytes.
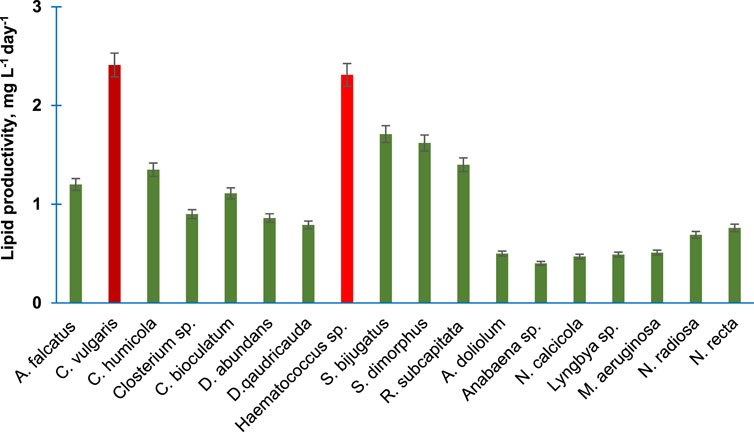
FIGURE 3. Lipid productivity of the purified algal species cultivated in the laboratory. Bars are means of triplicates ±S.D.
Subsequently, the percentage of lipid content presenting per unit dry weight of 18 purified algal species is presented in Figure 4. The percentage lipid content was highest in C. vulgaris (31%) followed by S. bijugatus and Haematococuss sp. by approximately 25%. The lipid content of all members of Cyanophyta was relatively lower than the average lipid content of other groups.
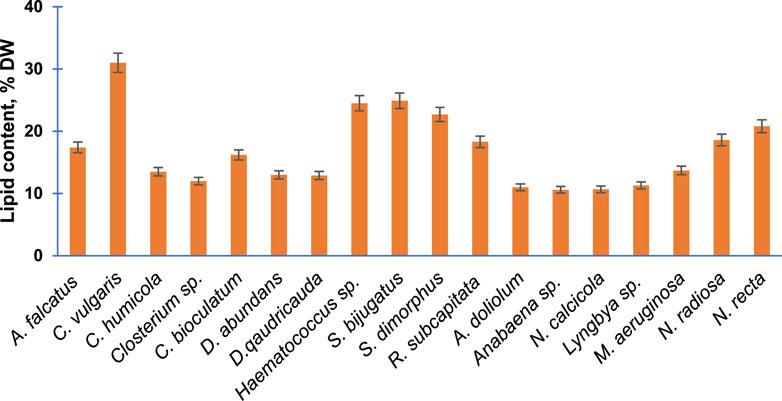
FIGURE 4. Total lipid content of the purified algal species cultivated in the laboratory. Bars are means of triplicates ±S.D.
3.3 Comparative assessment of the four selected algal species for growth and biomass and lipid productivity
The four algal species selected for the study (C. vulgaris, S. bijugatus, Anabaena sp., and N. recta) exhibited an almost sigmoid curve (Figure 5) that consisted of three phases: lag, log (exponential), and stationary. The lag phase for C. vulgaris, S. bijugatus, and N. recta lasted between 0 and 3 days, while for Anabaena sp., it extended up to the 6th day after initial inoculation. Afterward, all the algal species remained in the exponential phase for the next 12 days, followed by a stationary phase.
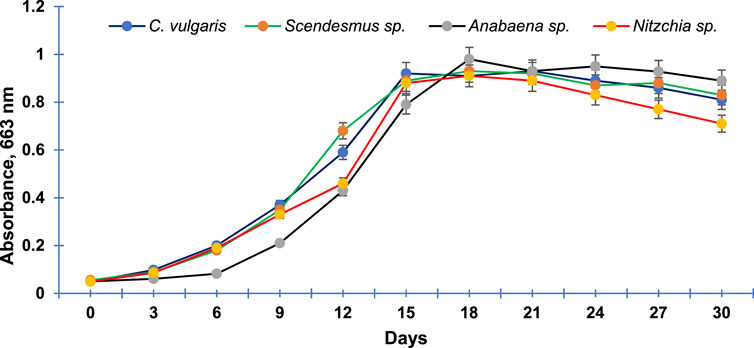
FIGURE 5. Growth curve of the most abundant freshwater algal species of Central India. Data points are means of triplicates ±S.D.
Figures 6A–D present the lipid and biomass productivity of the four selected algal species (C. vulgaris, S. bijugatus, Anabaena sp., and N. recta) at different time points. The lipid and biomass productivity of these species increased significantly over time. C. vulgaris had the highest lipid production, followed by S. bijugatus, while Anabaena sp. showed the lowest lipid production, and N. recta produced a moderate amount of lipids. The lipid productivity gradually increased in a time-dependent manner in all the selected algal species, except for N. recta which showed a continuous increment in lipid productivity until the end of the experiment (2 days). After 15 days, the lipid productivity remained almost constant in C. vulgaris, S. bijugatus, and Anabaena sp. The pattern of biomass productivity in these species was similar to their growth curve patterns: slower during the lag phase, increasing gradually during the exponential phase, and remaining almost unchanged during the stationary phase (Figures 6A–D). S. bijugatus had the highest biomass production followed by Anabaena sp., while Anabaena sp. showed higher biomass productivity than other species during the later phase of the experiment (late exponential phase and stationary phase). C. vulgaris had the highest lipid productivity but produced less biomass than S. bijugatus and Anabaena sp., while N. recta had slightly lower biomass productivity than C. vulgaris but its pattern was almost similar to that of C. vulgaris and S. bijugatus.
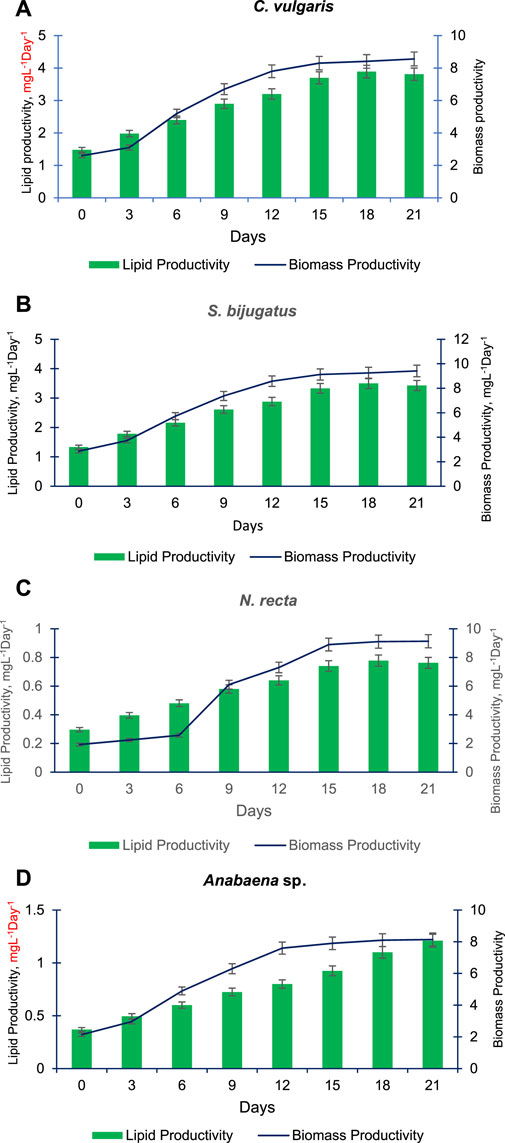
FIGURE 6. Lipid and biomass productivity of C. vulgaris (A), S. bijugatus (B), N. recta (C) and Anabaena sp. (D) at different time points.
3.4 Comparative assessment of the four selected algal species for total proteins and carbohydrate
Figure 7A shows the total proteins and total carbohydrate contents of the four selected algal species. As evident from the data, C. vulgaris possesses the highest amount of proteins (0.35 mg L−1) compared to other algal species. The carbohydrate contents of all algal species were almost equal. Similarly, Figure 7B presents the amount of chlorophyll a and carotenoids of the selected algal species. Chlorophyll a was almost equal in these algal species, and S. bijugatus showed slightly higher chlorophyll a compared to other species. The carotenoid contents of these algal species were also found to be almost equal.
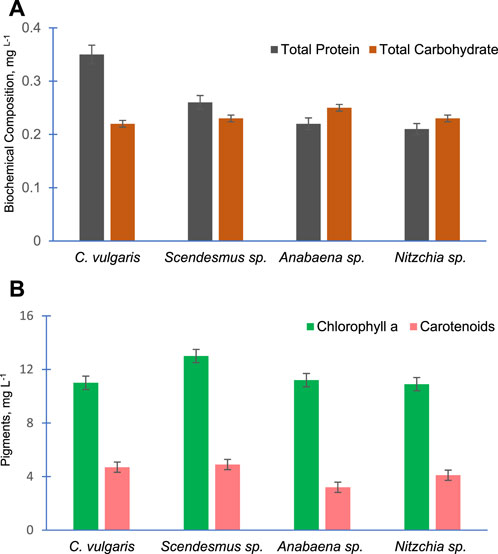
FIGURE 7. Total protein and total carbohydrate content (A) and chlorophyll a and carotenoid content (B) of the four freshwater algal species.
Figure 8A demonstrates the changes in the cellular content of N in the four algal species with time. Cellular N increased gradually with time in all these algal species. The pattern of change (increase) in cellular N in Anabaena sp. was different and slower compared to other algal species. Initially, the amount of cellular N was almost equal in C. vulgaris, S. bijugatus, and N. recta. However, cellular N started varying in these species during the later stage of algal culture, and S. bijugatus accumulated higher cellular N than C. vulgaris and N. recta, which accumulated the lowest amount of cellular N. The cellular N remained unchanged up to the 6th day of culturing Anabaena sp. and thereafter increased gradually with time.
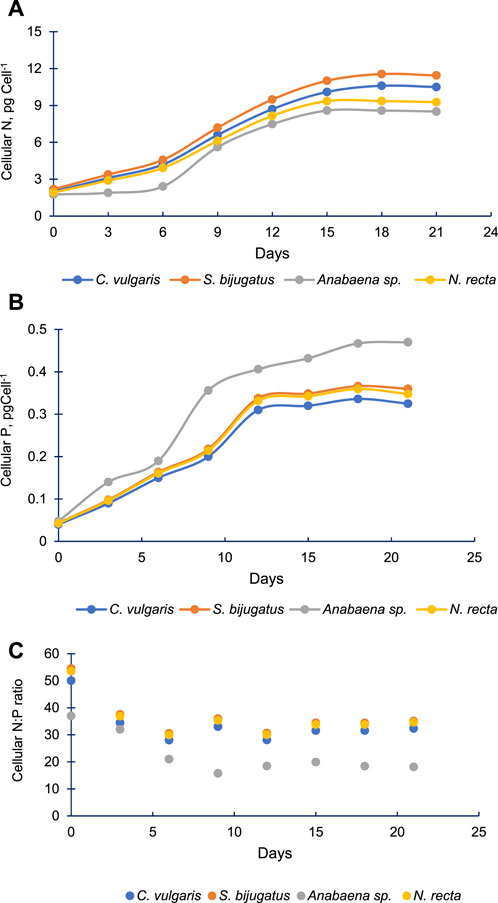
FIGURE 8. Cellular N (A), cellular P (B) and cellular N:P ratio (C) in four freshwater algal species at different time points.
3.5 Cellular N and P of the four algal species
Cellular P increased faster than cellular N in all four algal species (Figure 8B). All species increased their cellular P up to 12th day of culturing, and thereafter, cellular P remained constant till the end of the experiment (21st day) in C. vulgaris, S. bijugatus, and N. recta. However, in the case of Anabaena sp., the content of cellular P continuously increased till the end of the experiment. The cellular P of Anabaena sp. remained significantly higher than that of other species. The data reflect that Anabaena sp. accumulated high proportion of P than N compared to other algal species.
Based on the data on cellular N and P, it was observed that the cellular N:P ratio declined from approximately 50:1 to 30:1 in C. vulgaris, S. bijugatus, and N. recta and from approximately 37:1 to 18:1 in the case of Anabaena sp. (Figure 8C). The cellular N:P ratio in the first three algal species declined till the 6th day, whereas in Anabaena sp., it declined up to the 9th day and thereafter remained almost constant in all species.
3.6 Clustering of lipid and biomass productivity based on cellular N:P ratio
Clustering of lipid productivity according to the cellular N:P ratios of all algal species suggest three clusters, namely, cluster 1: near 50:1, cluster 2: between 30:35, and cluster 3 between 15:20 cellular N:P ratios (Figure 9A). Among these clusters, higher lipid productivity was observed between the 30:35 cellular N:P ratio. However, regression analysis suggests that N:P ratio has no significant impact on the lipid productivity of the algal species (Figure 9B). Similarly, three clusters were observed for biomass productivity (Figure 10A). Like lipid productivity, higher values of biomass productivity were observed between the 30:35 N:P ratio. Regression analysis suggested a negative relationship between N:P and biomass productivity. Biomass productivity was increased with the decrease in N:P ratio (Figure 10B).
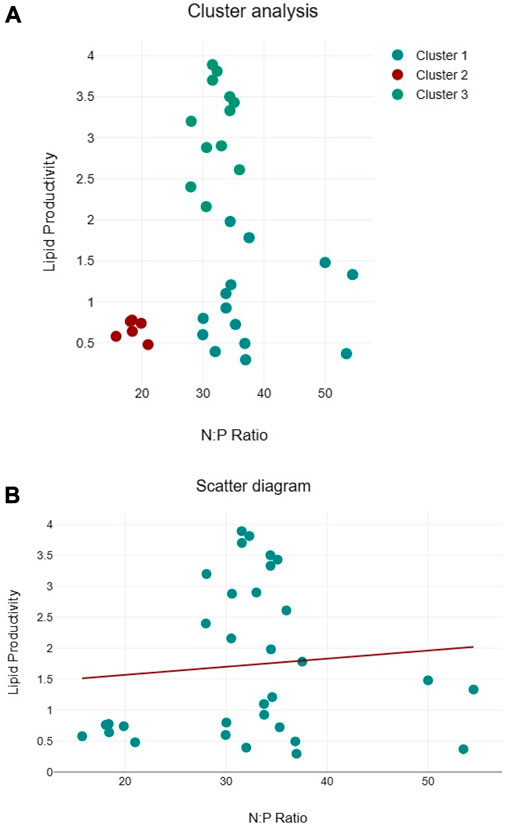
FIGURE 9. Cluster analyses between N:P ratio and lipid productivity (A) and regression analysis between N:P ratio and lipid productivity (B) of algal species.
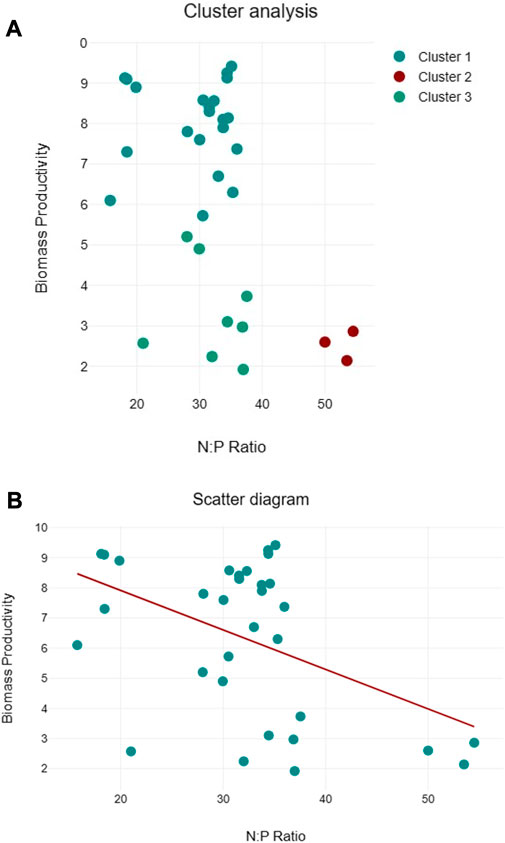
FIGURE 10. Cluster analyses between N:P ratio and lipid productivity (A) and regression analysis between N:P ratio and lipid productivity (B) of algal species.
4 Discussion
The present study provides insight into the potential of freshwater algal species of Central India for biofuel production. Central India possesses unexplored algal diversity largely unaffected by human interventions and, hence, remains a treasure for natural resources. The part of Central India (Chhattisgarh and Eastern Madhya Pradesh) has a tropical habitat, and waterbodies are relatively less degraded compared to other parts of India. Therefore, the occurrence of numerous freshwater algal species from Central India is quite possible due to suitable habitats supporting the growth of diverse types of freshwater algal species. Most of the waterbodies of the selected regions still remain oligotrophic or close to oligotrophic due to lesser human interventions, which supports the equal growth and distribution of diverse algal groups. In contrast, other parts of India have mostly eutrophic waterbodies that support the growth of only dominant algal species of a specific group (Gilbert, 2017). Nutrient availability, temperature, and pH are the key factors that influence algal diversity (Ikram et al., 2021). Collecting data on these parameters can provide better insight into the distribution of algal species in Central India. However, the present study was more focused on the collection of naturally occurring species and studying the potential of purified algal species in the laboratory. Therefore, we have not recorded the physiochemical parameters of various algal habitats. Shrivastava et al. (2018) also reported the occurrence of more than 30 freshwater algal species from the central part of India. However, this study was restricted to only the Bundelkhand region of Uttar Pradesh with fragmented forests, whereas the present study covers wider parts of Central India having dense forest areas.
The present data suggest that the members of Chlorophyta are faster in growth than those of Cyanophyta. The growth of algae largely depends on their ability to take up the essential nutrients from the external environment. In natural conditions, different algal species compete for resources due to sharing of the same nutrient resources. The ability of these species to accumulate nutrients significantly determines their position in the algal community. However, all the tested algal species were grown separately in the culture media; therefore, the ability of nutrient competition to decide the growth rate of the algal species in the present case is ruled out. The slower accumulation of nutrients by the members of Cyanophyta compared to other algal groups might be the reason for the slower growth rate of Cyanophyta. Previous studies also reported a slower growth rate of cyanophytes compared to Chlorophyta and others and linked it with a variety of factors including temperature and pH (Lurling et al., 2012). However, the climatic conditions, particularly the temperature of Central India, favor the growth of Cyanophyta, but the growth of the purified algal species was assessed in the laboratory at a fixed temperature, light, and pH. Therefore, it would be difficult to link any climatic factor to the slower growth of Cyanophyta in the present case.
The biomass productivity of several algal species, particularly cyanophyte members, was better than their specific growth rate. Biomass productivity was calculated based on their biomass measured in terms of dry weight, and we noticed that the cell weight of a few algal species with higher specific growth rates was lesser than that of slow growing algal species. Therefore, despite having lower cell numbers, as evident from the data of the specific growth rate, few species such as Anabaena sp. produced higher biomass due to heavier cell weight. According to the work of Wang et al. (2022), Cyanophyta members are known to store nutrients, particularly phosphate, in their cells, which can contribute to their cell weight. In some cases, heavier cell weight is associated with an adaptive strategy for certain habitat conditions. However, the present study was conducted under controlled laboratory conditions, and other factors were responsible for increasing the cell weight of cyanophytes but not the habitat-specific adaptation.
Furthermore, data showed the variation in lipid productivity in different algal species. The existence of variable lipid contents in different algal species has already been demonstrated previously (Aratboni et al., 2019). The lipid productivity of cyanophytes was the lowest compared to other algal groups. As elaborated by Aratboni et al. (2019), lipid production depends on a variety of factors, e.g., light, CO2, temperature, and nutrient limitation, but the available data in the present study do not permit us to conclude any precise reason for having lower lipids in the members of Cyanophyta. The present study was focused on studying total lipids of the algal species; however, fatty acid profiling, which can vary depending on species, growth conditions, and other factors, has a considerable importance in biofuel production. The information about variable biomass and lipid production in different freshwater algal species of Central India would be useful in selecting the appropriate species for biofuel production in the future.
Four algal species were selected considering multiple factors, e. g., fast growth rate, higher biomass and lipid production, and different algal groups. The growth curve of these algal species clearly showed an initial slower growth phase (lag) and thereafter a highly quicker growth phase (exponential) followed by a phase with constant growth (stationary phase). Typically, most algal species follow a sigmoid growth curve that includes a lag, log (exponential), stationary, and decline or death phase (Schanz and Zahler, 1981). The lag phase is the adaptation phase for the organism where they acclimatize themselves to the new environmental conditions provided. The growth is slow at this stage. The log phase is the phase of exponential growth where the population doubles at a constant rate. The stationary phase is when the population growth rate slows down and eventually stops due to limited resources. Finally, the decline or death phase is when the population starts to decrease due to the depletion of resources. The lipid productivity of all algal species increased over time. As previously mentioned, all algal species exhibited a typical growth curve consisting of different phases. Lipid productivity gradually increased over time and reached its highest point at the onset of the stationary growth phase. It remained almost constant during the entire stationary phase of all algal species except N. recta. Based on the observations, it can be suggested that the stationary growth phase is the suitable stage for harvesting algal species for biofuel production. Many researchers have linked lipid production with nutrient depletion (Sun et al., 2018). In this study, the algal species were grown in batch culture. Batch culture depletes nutrients over time, leading to nutrient limitation and pH drift in the culture medium (Tripathi et al., 2003; Tripathi et al., 2009). Nutrient limitation occurring over time in batch culture may have induced lipid production in these algal species in a time-dependent manner in this study. Previous research suggests that during stressful conditions including nutrient limitation, algal cells prefer to synthesize more lipids as storage products than carbohydrates (Shi et al., 2020).
The biomass productivity of the selected algal species followed the pattern of their growth curves. Several factors, e.g., light, temperature, and most importantly, nutrients, are important regulators of the growth and biomass production of algal species (Barry et al., 2015). But, we have not studied different environmental factors controlling algal growth and biomass. However, cellular N and P were assessed, and it appears from the data of cluster and regression analysis that cellular N:P ratio may have contributed to the algal biomass production in the current study. In the cluster analysis, values of higher biomass productivity are located between the N:P ratio of 30 and 35:1, but few high values of biomass productivity are located near the N:P ratio of 18:1, close to the Redfield ratio (16:1) emphasizing the relevance of the N:P ratio for algal growth (Tett et al., 1985). The Redfield ratio is useful in determining the regulation of algal growth by nutrients. Like biomass productivity, the values of lipid productivity clustered around three different N:P ratios, with the majority of higher values near N:P ratios between 30 and 35:1. Regression analysis shows no clear association between lipid productivity and cellular N:P ratios, limiting our conclusions. Since cellular N and P play pivotal roles in several metabolic activities, therefore, more research is needed to understand how the cellular N:P ratio regulates algal biomass and lipid productivity, particularly the role of the cellular N:P ratio in optimizing lipid productivity, improving nutrient utilization, and elucidating the molecular mechanisms associated with these processes.
The findings of the present study would lead to new directions of the research on algal biofuel production involving freshwater algal species. Additionally, the findings suggest exploring the possibility of using the cellular N:P ratio in regulating algal biomass and lipid production. Currently, algal biofuel production is a promising area of research worldwide; hence, naturally occurring superior freshwater algal species will have great significance as an excellent feedstock for algal biofuel production.
Data availability statement
The original contributions presented in the study are included in the article/Supplementary Material; further inquiries can be directed to the corresponding author.
Author contributions
VS: formal analysis, methodology, and writing–original draft. SI: methodology, data curation, writing–review and editing. BT: conceptualization, supervision, and writing–review and editing.
Funding
The author(s) declare that no financial support was received for the research, authorship, and/or publication of this article. Financial support from the Department of Biotechnology, New Delhi, India, in the form of a DBT-BUILDER-IGNTU project (Grant No. BT/INF/22/SP45361/2022) is gratefully acknowledged.
Acknowledgments
The authors are thankful to Akhileshwar Singh and Shubham K. Dubey for their help in the collection of algal samples.
Conflict of interest
The authors declare that the research was conducted in the absence of any commercial or financial relationships that could be construed as a potential conflict of interest.
The authors declared that they were editorial board members of Frontiers, at the time of submission. This had no impact on the peer review process and the final decision.
Publisher’s note
All claims expressed in this article are solely those of the authors and do not necessarily represent those of their affiliated organizations, or those of the publisher, the editors, and the reviewers. Any product that may be evaluated in this article, or claim that may be made by its manufacturer, is not guaranteed or endorsed by the publisher.
Supplementary material
The Supplementary Material for this article can be found online at: https://www.frontiersin.org/articles/10.3389/fenrg.2023.1271660/full#supplementary-material
References
Andrew, A. R., Yong, W. T. L., Misson, M., Anton, A., and Chin, G. J. W. L. (2022). Selection of tropical microalgae species for mass production based on lipid and fatty acid profiles. Front. Energy Res. 10, 912904. doi:10.3389/fenrg.2022.912904
Anschau, A., Caruso, C. S., Kuhn, R. C., and Franco, T. T. (2017). Validation of the sulfo-phospho-vanillin (SPV) method for the determination of lipid content in oleaginous microorganisms. Braz. J. Chem. Eng. 34, 19–27. doi:10.1590/0104-6632.20170341s20140222
Aratboni, A. H., Rafiei, N., Garcia-Granados, R., Alemzadeh, A., and Morones-Ramírez, J. R. (2019). Biomass and lipid induction strategies in microalgae for biofuel production and other applications. Microb. Cell Factories 18, 178–217. doi:10.1186/s12934-019-1228-4
Barry, A. N., Starkenburg, S. R., and Sayre, R. T. (2015). Strategies for optimizing algal biology for enhanced biomass production. Front. Energy Res. 3, 1. doi:10.3389/fenrg.2015.00001
Bellinger, E. G., and Sigee, D. C. (2015). Freshwater algae: Identification, enumeration and use as bioindicators. New York, United States: John Wiley and Sons.
Bligh, E. G., and Dyer, W. J. (1959). A rapid method of total lipid extraction and purification. Can. J. Biochem. physiology 37 (8), 911–917. doi:10.1139/y59-099
Datatab Team, (2023). DATAtab: Online statistics calculator. Graz, Austria: DATAtab eU. Available from URL: https://datatab.net.
Desikachary, T. V. (1955). On a helminthora from New Zealand. Am. J. Bot. 42 (2), 126–131. doi:10.1002/j.1537-2197.1955.tb11104.x
Glibert, P. M. (2017). Eutrophication, harmful algae and biodiversity-challenging paradigms in a world of complex nutrient changes. Mar. Pollut. Bull. 124 (2), 591–606. doi:10.1016/j.marpolbul.2017.04.027
Guillard, R. R. (2005). Purification methods for microalgae. Algal Cult. Tech., 117–132. doi:10.1016/b978-012088426-1/50009-3
Hannon, M., Gimpel, J., Tran, M., Rasala, B., and Mayfield, S. (2010). Biofuels from algae: challenges and potential. Biofuels 1 (5), 763–784. doi:10.4155/bfs.10.44
Hempel, N., Petrick, I., and Behrendt, F. (2012). Biomass productivity and productivity of fatty acids and amino acids of microalgae strains as key characteristics of suitability for biodiesel production. J. Appl. Phycol. 24, 1407–1418. doi:10.1007/s10811-012-9795-3
Hussain, J., and Rittmann, B. E. (2023). Algae as a source of renewable energy: opportunities, challenges, and recent developments. Sustain. Energy and Fuels 7, 2515–2544. doi:10.1039/d2se01599d
Ikram, S. F., Kumar, D., Singh, V., Tripathi, B. N., and Kim, B. H. (2021). Microalgal and cyanobacterial diversity of two selected hot springs of Garhwal Himalaya, Uttarakhand, India. Fundam. Appl. Limnol. 195 (2), 111–127. doi:10.1127/fal/2021/1366
Jagadevan, S., Banerjee, A., Banerjee, C., Guria, C., Tiwari, R., Baweja, M., et al. (2018). Recent developments in synthetic biology and metabolic engineering in microalgae towards biofuel production. Biotechnol. biofuels 11, 185–221. doi:10.1186/s13068-018-1181-1
Khraiwesh, B., Jijakli, K., Swift, J., Chaiboonchoe, A., Abdrabu, R., Chao, P. W., et al. (2015). Prospective applications of synthetic biology for algal bioproduct optimization. Biomass Biofuels Microalgae Adv. Eng. Biol., 137–154. doi:10.1007/978-3-319-16640-7_8
Lowry, O., Rosebrough, N., Farr, A. L., and Randall, R. (1951). Protein measurement with the Folin phenol reagent. J. Biol. Chem. 193 (1), 265–275. doi:10.1016/s0021-9258(19)52451-6
Lurling, M. F. L. L. W. (2012). Infodisruption: pollutants interfering with the natural chemical information conveyance in aquatic systems. Chem. Ecol. aquatic Syst., 250–271. doi:10.1093/acprof:osobl/9780199583096.003.0018
Mackinney, G. (1941). Absorption of light by chlorophyll solutions. J. Biol. Chem. 140 (2), 315–322. doi:10.1016/s0021-9258(18)51320-x
Martin, L. A., Popovich, C. A., Damiani, M. C., and Leonardi, P. I. (2020). A practical tool for selecting microalgal species for biodiesel production. J. Renew. Sustain. Energy 12 (6). doi:10.1063/5.0010668
Mohsenpour, S. F., Hennige, S., Willoughby, N., Adeloye, A., and Gutierrez, T. (2021). Integrating micro-algae into wastewater treatment: A review. Sci. Total Environ. 752, 142168. doi:10.1016/j.scitotenv.2020.142168
Mutanda, T., Ramesh, D., Karthikeyan, S., Kumari, S., Anandraj, A., and Bux, F. (2011). Bioprospecting for hyper-lipid producing microalgal strains for sustainable biofuel production. Bioresour. Technol. 102 (1), 57–70. doi:10.1016/j.biortech.2010.06.077
Myers, J., and Kratz, W. A. (1955). Relations between pigment content and photosynthetic characteristics in a blue-green alga. J. General Physiology 39 (1), 11–22. doi:10.1085/jgp.39.1.11
Nicholas, D. D., and Nason, A. (1957). “Determination of nitrate and nitrite,” in Methods in enzymology, 3. Editors S. P. Colowick, and N. O. Kaplan, 981–984.
Pandey, M. K., Dasgupta, C. N., Mishra, S., Srivastava, M., Gupta, V. K., Suseela, M. R., et al. (2019). Bioprospecting microalgae from natural algal bloom for sustainable biomass and biodiesel production. Appl. Microbiol. Biotechnol. 103, 5447–5458. doi:10.1007/s00253-019-09856-2
Philipose, M. T. (1959). “Freshwater phytoplankton inland fisheries,” in Proceedings of the symposium on Algology, 279, New Delhi, India, January 4-5, 1959, 291.
Rasala, B. A., Chao, S. S., Pier, M., Barrera, D. J., and Mayfield, S. P. (2014). Enhanced genetic tools for engineering multigene traits into green algae. PloS one 9 (4), e94028. doi:10.1371/journal.pone.0094028
Santhakumaran, P., Kookal, S. K., Mathew, L., and Ray, J. G. (2019). Bioprospecting of three rapid-growing freshwater green algae, promising biomass for biodiesel production. BioEnergy Res. 12, 680–693. doi:10.1007/s12155-019-09990-9
Schanz, F., and Zahler, U. (1981). Prediction of algal growth in batch cultures. Schweiz. Z. für Hydrol. 43, 103–113. doi:10.1007/bf02502475
Shi, T. Q., Wang, L. R., Zhang, Z. X., Sun, X. M., and Huang, H. (2020). Stresses as first-line tools for enhancing lipid and carotenoid production in microalgae. Front. Bioeng. Biotechnol. 8, 610. doi:10.3389/fbioe.2020.00610
Snow, A. A., and Smith, V. H. (2012). Genetically engineered algae for biofuels: a key role for ecologists. Bioscience 62 (8), 765–768. doi:10.1525/bio.2012.62.8.9
Srivastava, N., Suseela, M. R., Toppo, K., and Lawrence, R. (2018). Fresh water algal diversity of Central India. Int. J. Res. Dev. Pharm. Life Sci. 7 (4), 3039–3049. doi:10.21276/ijrdpl.2278-0238.2018.7(4).3039-3049
Sun, X. M., Ren, L. J., Zhao, Q. Y., Ji, X. J., and Huang, H. (2018). Microalgae for the production of lipid and carotenoids: A review with focus on stress regulation and adaptation. Biotechnol. biofuels 11, 272–316. doi:10.1186/s13068-018-1275-9
Tett, P., Droop, M. R., and Heaney, S. I. (1985). The Redfield ratio and phytoplankton growth rate. J. Mar. Biol. Assoc. U. K. 65 (2), 487–504. doi:10.1017/s0025315400050566
Tripathi, B. N., Kasana, R., Singh, V., Bhatt, I., Singh, A., Sharma, V., et al. (2009). Carotenoids and pH of the culture medium play an important role in displaying metal stress in batch and semi-continuous cultures of Anabaena doliolum. Ann. de Limnologie-International J. Limnol. 45 (2), 119–125. doi:10.1051/limn/2009010
Tripathi, B. N., Mehta, S. K., and Gaur, J. P. (2003). Differential sensitivity of Anabaena doliolum to Cu and Zn in batch and semicontinuous cultures. Ecotoxicol. Environ. Saf. 56 (2), 311–318. doi:10.1016/s0147-6513(02)00164-1
Tripathi, B. N., Mehta, S. K., and Gaur, J. P. (2004). Recovery of uptake and assimilation of nitrate in Scenedesmus sp. previously exposed to elevated levels of Cu2+ and Zn2+. J. plant physiology 161 (5), 543–549. doi:10.1078/0176-1617-01238
Keywords: algae, biofuel, biomass, freshwater, lipids
Citation: Singh V, Ikram SF and Tripathi BN (2023) Exploring the potential of freshwater algal species for biofuel production. Front. Energy Res. 11:1271660. doi: 10.3389/fenrg.2023.1271660
Received: 02 August 2023; Accepted: 14 September 2023;
Published: 28 September 2023.
Edited by:
G. Venkata Subhash, Reliance Industries, IndiaReviewed by:
Amar Musale, Reliance Industries, IndiaRajasri Yadavalli, Chaitanya Bharathi Institute of Technology, India
Copyright © 2023 Singh, Ikram and Tripathi. This is an open-access article distributed under the terms of the Creative Commons Attribution License (CC BY). The use, distribution or reproduction in other forums is permitted, provided the original author(s) and the copyright owner(s) are credited and that the original publication in this journal is cited, in accordance with accepted academic practice. No use, distribution or reproduction is permitted which does not comply with these terms.
*Correspondence: Bhumi Nath Tripathi, Ymh1bWluYXRodHJpcGF0aGlAaG90bWFpbC5jb20=