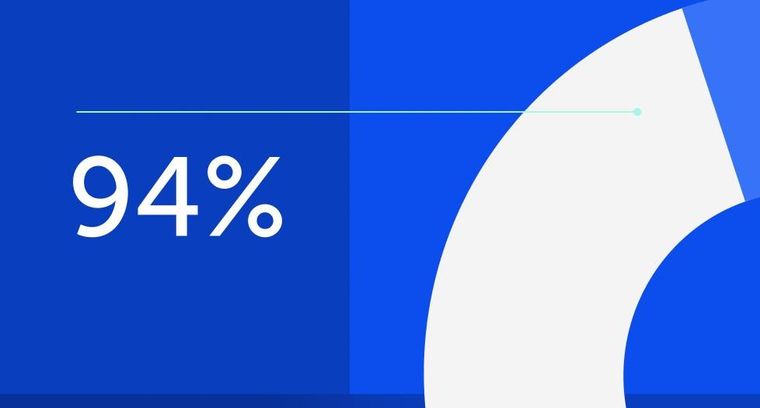
94% of researchers rate our articles as excellent or good
Learn more about the work of our research integrity team to safeguard the quality of each article we publish.
Find out more
REVIEW article
Front. Energy Res., 19 September 2023
Sec. Sustainable Energy Systems
Volume 11 - 2023 | https://doi.org/10.3389/fenrg.2023.1268270
This article is part of the Research TopicEnergy-Efficient and Energy-Flexible Buildings Towards Net-Zero Carbon EmissionView all 6 articles
Academia has a crucial role to play in informing urgently needed actions on climate mitigation. It is vital to understand what is known about the potential contribution of climate mitigation options, the barriers that exist to achieving that contribution, and to quantify the research balance and geographic focus of these various approaches across the literature. This PRISMA-based systematic literature review aims to provide the reader with the following: Firstly, an overview of the post-Paris climate mitigation research landscape and secondly, an assessment of the climate mitigation potential of those options per the literature reviewed. Analysis of the research landscape demonstrated that supply-side research greatly outnumbers that on the demand-side, which totalled just half of that which focused on the supply-side. In terms of the geographic scale, the reviewed literature was dominated by national-level studies, with sub-national studies the least common, particularly those at a local government level. Given this, it can be concluded that two key areas would benefit from further research–that focusing on demand-side mitigation, and that carrying research out at more local levels. On climate mitigation potential, wind and solar energy were found to be the biggest contributors to a decarbonised energy supply, across a range of study areas. Discrepancies were identified between findings in the academic and grey literature for several options, chiefly bioenergy and nuclear power: bioenergy made significantly higher contributions in the academic literature versus grey literature, with the opposite true for nuclear. Demand-side options all demonstrated significant mitigation potential in the literature reviewed but received very limited coverage in comparison to many of their supply-side counterparts. Future research should pursue this knowledge gap to reach a better understanding of the contributions they can make and ensure that policymakers have the data necessary to chart a course to a zero-carbon future.
The IPCC’s Sixth Assessment Report states that “any further delay in concerted anticipatory global action on adaptation and mitigation will miss a brief and rapidly closing window of opportunity to secure a liveable and sustainable future for all” (IPCC, 2022, p. 35). Academia has a crucial role to play in informing these urgently needed actions on climate mitigation. A comprehensive understanding of the choices available in mitigating climate change is therefore vital in ensuring that policymakers have the resources needed to design robust and comprehensive strategies to accelerate the transition to zero-carbon. This paper aims to contribute to that understanding, through an analysis of the potential contributions these options are judged to be capable of. This paper also provides researchers with an overview of the current research landscape surrounding climate mitigation options, through an assessment of the prevalence and geographic focus of different mitigation options and approaches within this body of research.
Numerous pathways have been proposed for achieving this zero-carbon transition. At a UK level, these include the Climate Change Committee’s (CCC) Sixth Carbon Budget and the UK Governments Net Zero Strategy. The former’s “Balanced Net Zero Pathway” (CCC, 2020) assigns 60% of the needed emission reductions for reaching net-zero to the years running to 2035, with the fastest rates of decarbonisation seen in the early 2030s. Sectorally, these reductions are most rapid in energy supply, followed by the surface transport, manufacturing and construction, and building sectors. Aviation and agriculture do not reach zero-emissions and thus require offsetting. The pathway sets key phase-out dates for carbon-intensive activities; fossil-fuel vehicles by 2032, residential oil and gas boilers by 2028 and 2033 respectively, and new build (unabated) gas power plants by 2030. Additionally, the importance of reducing demand is emphasised, with the pathway allocating 10% of emissions savings in 2035 here.
The Net Zero Strategy (BEIS, 2021) provides several illustrative 2050 scenarios, all reaching net-zero through the same rate of decarbonisation. The first explores the potential for doubling the UK’s 2021 electricity generation levels to approximately 690 TWh, of which a proportion is then utilised in scaling up hydrogen production to 240 TWh to supply the industrial and transportation sectors. As with the CCC’s pathway, residual emissions remain in the aviation, agricultural, and waste sectors, which are offset via negative emissions technologies (NETs). The second scenario develops hydrogen production further, to 500 TWh, chiefly through the large-scale deployment of CCS-equipped hydrogen-from-gas facilities (“blue hydrogen”). This lessens the necessary increase in electricity production to 610 TWh, firstly due to hydrogen’s increased use in place of electricity in meeting the heat demand of buildings, and secondly the reduced demand for hydrogen-from-electrolysis (“green hydrogen”). The final pathway assumes a high pace of innovation in sustainable fuels, successfully reducing hard-to-abate emissions from the aviation and agricultural sectors and entailing electricity and hydrogen generation levels somewhere between the two preceding pathways.
At a global scale, Chapter 2 of the IPCC’s Special Report: Global Warming of 1.5°C (IPCC, 2018) lays out the key characteristics of pathways consistent with maintaining 1.5°C of warming or less. To have a 50% probability of achieving this, emissions need to remain below 580 GtCO2 from 2018 onwards, and under 420 GtCO2 to bring this probability up to 66%. Again, a range of illustrative scenarios are provided to demonstrate different approaches to reaching this target. There is a great variety in different combinations of mitigation options represented, with one pathway prioritising social, business, and technological innovations in reducing demand, doing without the need for NETs, and another highly energy-intensive scenario heavily reliant on the large-scale rollout of NETs from 2030 onwards.
Within these pathways and strategies, a plethora of options have been suggested. Broadly, these can be categorised into the areas of either, a) technologies providing net-zero energy supply (including point-source carbon capture), b) changes reducing total energy demand, or the carbon intensity of demand, and c) the direct removal of CO2 from the atmosphere. These are henceforth referred to as “supply-side,” “demand-side,” and “atmospheric removal” measures, respectively. Supply-side options include those which are perhaps the more commonly discussed (CREDS, 2019; IEA, 2022a); efforts to decarbonise the energy supply through a shift in fossil-fuel use to low-carbon renewable alternatives, and the deployment of carbon-capture technologies alongside bioenergy. Measure on the demand-side encompasses an increasingly active area of research (Toma et al., 2019), with solutions here targeting technology adoption, changes in consumption and behaviour, service provision and associated socio-technical transitions (Creutzig et al., 2018). Examples here include electrification in the transport sector, efficiency improvements in the buildings and industrial sectors, and shifts towards low-carbon diets and active travel under the umbrella of behaviour change.
Atmospheric removal covers both technological means of capture, termed direct air capture (DAC), and nature-based solutions (NBS). DAC captures atmospheric CO2 either by passing air through a chemical solution or by binding it to sorbent filters where it is then stored or utilised (IEA, 2021a). NBS options include reforestation, wetland restoration and conservation, and restorative agricultural practices, as ways of preventing GHG emissions (e.g., from swamps and marshes) or actively removing them from the atmosphere. As laid out in the IPCC’s report “Global Warming of 1.5°C,” atmospheric removal has two roles to play in climate change mitigation pathways, being “i) to move more rapidly towards the point of carbon neutrality and maintain it afterwards in order to stabilize global mean temperature rise, and ii) to produce net negative CO2 emissions, drawing down anthropogenic CO2 in the atmosphere in order to decline global mean temperature after an overshoot peak” (IPCC, 2018, p. 122).
Transitioning to net-zero will require changes across all of these areas. Taking transport as an example, a zero-carbon transition could involve supply-side electricity decarbonisation through the deployment of renewable energy technology, demand-side measures to encourage the uptake of electric vehicles (EVs) and active travel options, and atmospheric removal to account for hard-to-abate emissions involved in vehicle production. Quantifying the balance of research between these three approaches in one of the objectives of this paper.
Building a better understanding of the geographic scales at which climate mitigation research is performed presents another objective in understanding the wider research landscape. Supply-side options are often envisaged at a national or global scale. The National Grid (2021) and CCC (2020), for example, have produced an array of work on pathways for decarbonising the UK’s energy supply, while organisations and multinationals including the European Commission (2020), International Energy Agency (2021b), BP (2021) and Shell (2021) perform similar work at an international level. This is not to say that supply-side options are not considered at smaller scales, however. Kobashi et al. (2020a) analyse the potential for city-scale deployment of solar photovoltaics (PV) in Kyoto, for example, while the Liverpool City Region Combined Authority’s “Pathway to Net Zero” report (2022) cites tidal power developments along the River Mersey as a major contributor. Likewise, demand-side options, such as EVs, can be observed in strategies ranging from a global scale (IEA, 2020) down to a city scale (Pamucar et al., 2021). The regions and countries in which these options are discussed vary. There are many reasons for this, such as the availability of the resource needed for a given option, the cost-effectiveness of each option, and political considerations including the level of public support for an option, all of which factor into the relative emphasis placed on each option by policymakers in national climate strategies.
Previous systematic reviews on zero-carbon transitions have tended to focus on a specific region, sector, or mitigation option. For example, Xiao, Simon and Pregger (2019) investigate policies on transitioning coastal China to net-zero, Kourgiozou et al. (2021) examine the discourse around decarbonising the education sector, and Martin, Agnoletti and Brangier (2020) explore the literature on hydrogen as an energy source. There is an absence of reviews covering the breadth of options for facilitating zero-carbon transitions more generally.
The need exists for a systematic review covering the options available for transitioning to zero-carbon. It is frequently argued that supply-side and demand-side decarbonisation, along with direct removal of CO2 from the atmosphere, will be needed in combination to reach net-zero (Rogelj et al., 2015; Creutzig et al., 2018; CREDS, 2019; IEA, 2022a). As academic research often informs policy, it is vital to understand what is known (and what is not) about the potential contribution of, and barrier to, these various approaches, and to quantify the research balance and geographic focus of the various mitigation approaches within literature. Doing so will highlight areas of significant disagreement and areas that may not be receiving adequate attention, which will be important in ensuring that academia is providing policymakers with the resources they need.
To that end, this systematic literature review aims to provide the reader with the following:
Firstly, an overview of the post-Paris climate mitigation research landscape, in terms of the split between different mitigation approaches, the prevalence of options discussed therein, and the geographic focus and spatial scale of research.
Secondly, an assessment of the climate mitigation potential of those options per the literature reviewed, in terms of energy contribution or emissions reduction potential, and barriers faced in reaching that potential.
Traditional, “narrative” literature reviews have long been a tool for collating and summarising knowledge within a field or on a specific topic. Systematic reviews go a step further, seeking to minimise subjectivity in the review and introduce reproducibility in the methods used. To this end, they follow a strict protocol in which the research questions, search strategies, and analysis methodologies are clearly defined.
This review made use of the Preferred Reporting Items for Systematic reviews and Meta-Analyses (PRISMA) statement, consisting of a checklist and flow diagram aimed at aiding authors in improving their reporting of systematic reviews (Page et al., 2021), and enhancing replicability. Initially designed by the medical community for applications in healthcare research, the process has since been used in a wide range of areas including engineering, economics, computer science, and environmental sciences, either in its original or adapted form (e.g., Shaffril et al., 2018; Haris et al., 2020; Sorgho et al., 2020).
The process of selecting literature for inclusion in the analysis is detailed in Figure 1; Table 1. In short, the Scopus and Web of Science databases were searched, with the results gradually whittled down through a screening process, applying a set of exclusion criteria to firstly the article titles, then abstracts, and finally full texts. In cases where it was ambiguous whether an article met these criteria, lenience was shown and the article passed to the next stage. The final search of these databases was carried out on 12 December 2022.
FIGURE 1. PRISMA flowchart of the literature selection process. Original from: Page MJ, McKenzie JE, Bossuyt PM, Boutron I, Hoffmann TC, Mulrow CD, et al. The PRISMA 2020 statement: an updated guideline for reporting systematic reviews.
The search was limited to work published from 2015 onwards. This was chosen as the lower search limit for this review as the review focuses on “net-zero” carbon transitions specifically (rather than low or reduced carbon), with Scopus’ inbuilt analysis tool showing a significant increase in publications related to this term post-2015–perhaps to be expected with the Paris Agreement being adopted that same year. Searches were also restricted to those published in English and excluded articles in press or otherwise unfinalised. Restricting the literature search to English-only papers may have introduced a geographical bias to the results. However, the inclusion of non-English papers was deemed not infeasible given a lack of resources available for professional translation services.
To assess the contributions academic research is making to policy, it is helpful to understand what mitigation options have been discussed within governmental and non-governmental organisations in recent years. The literature search was therefore extended to grey literature. UK-focused literature was used as a case study to compare the options discussed within an academic context with those in an organisational context. The UK was chosen for this purpose as it was the most prevalent study area in the academic literature search, thus providing the largest source of literature from which to forward reference.
The PRISMA statement covering the use of non-database sources was followed, employing a process of citation searching of UK-focused academic literature. From this initial search, grey literature was selected for inclusion based on the same criteria applied to the academic sources (Table 1), with the additional stipulation that the work must appear more than once in the searching process. This was due to a combination of time constraints and wanting to select the most prominent documents as part of the case study.
A form was used to record the key information from each study. The data items in these forms were as follows: Author; Year; Journal; Methods; Transition Level (Supply/Demand/Removal); Transition(s) Discussed; Spatial Scope; Geographic Focus; Comments on Feasibility/Barriers/Contribution/Study Methodologies and Key Assumptions. From these data forms, the research questions could be answered.
This section provides findings on the state of the research landscape surrounding net-zero transition options, in terms of the split between different mitigation areas, the varying geographic scales and foci of the research, and the specific mitigation options discussed therein.
The academic literature was broken down into four categories based on research focus: Supply-side, Demand-side, Atmospheric Removal, and Integrated.
Supply-side research constitutes 47% of the studies reviewed, totalling 40 papers. Demand side research accounts for 24.7% of the total with 21 papers, while studies focusing on atmospheric removal (9, 10.6% of total), and those integrating two or more of these approaches (15, 17.6% of total) account for the remainder. Authorship details for these papers can be found in Supplementary Appendix SA.
The approach taken in grey literature sourced from UK-focused academic literature typically discussed transition options in an integrated approach, rather than focusing on a single supply or demand-side option. 10 of the 12 reports took this approach, leaving just one focusing on the supply-side [the Climate Change Committee’s “Hydrogen in a low-carbon economy (2018)”], and one looking at nature-based solutions [“Cutting the Climate Impact of Land Use, from the Green Alliance (2019)”].
Within the academic literature, national-level studies proved the most numerous, totalling 50 of the 85 studies. Sub-national studies were the least prevalent, totalling just eight. Typically, these studies carried out their research at the principal administrative division (e.g., an Australian state (Gurieff et al., 2021), a Chinese province (Bamisile et al., 2022) or a Japanese prefecture (Cong et al., 2022). Two chose lower divisions; Martes and Köhl (2022) focusing on the Hamburg Metropolitan Area, and Pilpola et al. (2019) the city of Helsinki. Global-level studies (15) and international studies (13) constituted the remaining papers. Note that the count totals 86, rather than the 85 papers included in this review. This is due to one study comprising both a national and sub-national assessment (Pipola et al., 2019). The predominance of national-level studies within the academic literature could be seen as beneficial in informing national-level decarbonisation policy. This is highly dependent on how those policies are formulated, however. If a bottom-up approach were to be taken, the relative lack of studies on sub-national decarbonisation represents an important knowledge gap.
Within the academic international assessments, European nations were the most prevalent, featuring in nine of the 13 studies. Similarly, national-level assessments were dominated by the UK, being the subject of 14 papers. Sub-national studies did not exhibit a predominant geographic focus. Additional details on the breakdown of geographic focus can be found in Supplementary Appendix SB.
Grey literature consisted of exclusively national-level work covering the UK. This is unsurprising given that this literature was citation searched from UK-focused academic studies, though it does suggest that national-level studies may not be considered in policies beyond the nation they originated.
Table 2 presents a breakdown of mitigation options discussed within supply-side and demand-side literature. Further discussion on each of these options follows in Section 3.2.
In additional to these two mitigation areas is the atmospheric removal of greenhouse gases. Within this portion of the literature, eight of the nine studies focused on nature-based solutions, and one on direct air capture. Four of the NBS’s papers carried their research out on a global scale, while one looked at Germany, one at Japan, and one at the United Kingdom. Authorship details for these papers can be found in Supplementary Appendix SC.
An additional table (Supplementary Appendix SD) is also available within the Supplementary Material, listing the breakdown of literature in those studies that did not focus on just one of these three areas, but instead chose to integrate two or more. Findings on potential for specific supply-side/demand-side/atmospheric removal options within these integrated studies are incorporated into the relevant subsections of Section 3.2.
Little in the way of geographic focus is found within most of the literature surrounding a given mitigation option. The exceptions to this are hydropower where three of the eight studies focus on China, and solar power, where over half of the literature places its attention on sunbelt countries. The majority of the literature focused on either Europe or Asia, with the UK and China receiving significant attention. Given the English language restriction of the literature search, this is to be expected.
Tables 3, 4 detail the potential contributions of wind and solar energy, garnered from the academic literature. These tables, and all in Section 3.2, list the contribution from the most ambitious scenario (though still plausible, per the authors) where multiple scenarios are presented, to portray the potential of a given option. Furthermore, where authors employ an established model, this is specified. Where the model is of the author’s design, this is given as n/a.
Wind shows great potential in the academic literature, exceeding 50% of electricity generation in several cases across a variety of study areas (Lugovoy et al., 2021a; Martinez-Gordon et al., 2022; Scheepers et al., 2022; Simon et al., 2022). This compares well with projections set out in the UK-focused grey literature. The CCC’s Balanced Net Zero pathway (2020) models 430.4 TWh of generation from wind in 2050 (of 748.9 TWh total) in the UK, while the National Grid’s Future Energy Scenarios (FES) report (2021) goes further, estimating between 513.9 TWh (of 723.6 TWh total) and 644.6 TWh (of 822.7 TWh total) of wind generation come 2050. At a global level, the IEA (2022a) place the share of wind power at 32% of total electricity generation under their Net Zero Emissions 2050 scenario, second only to solar power.
Solar energy is positioned as another key option in transitioning to net-zero in the academic literature. As with the literature on wind energy, numerous studies project solar supplying over half of all electricity generation in their given study areas (Handayani et al., 2022; Kanugrahan et al., 2022; Lugovoy et al., 2021b; Manjong et al., 2021), the highest share being 86% in the Majong et al. study of Cameroon in 2050. This is reflected in the IEA’s global Net Zero Emissions scenario, which apportions 37% of the world’s electricity generation to solar in 2050 (IEA, 2022a). Within UK-focused grey literature, solar makes a markedly smaller contribution than wind. The National Grid (2021) pathways place solar’s contribution between 49.5 TWh and 80.7 TWh in 2050 (of 723.6 TWh and 701.0 TWh totals, respectively), while the CCC (2020) model 83.3 TWh of generation (of 748.9 TWh total). This is largely a result of the very high offshore wind potential of the UK, with similar findings in the academic literature which focused on comparably coastal study areas [Scheepers et al., 2022 (Netherlands); Martinez-Gordon et al., 2022 (North Sea Region)].
The spatial and temporal constraints regarding wind and solar resource availability are frequently discussed in the literature (Gulagi et al., 2017; Gulagi et al., 2018; Barthelmie and Pryor, 2021; Dominkovic et al., 2016; Lugovoy et al., 2021b; Ji et al., 2022; Scheepers et al., 2022; Zhai et al., 2023). Solutions proposed to alleviate these issues include diversifying the energy system so that it is not overly reliant on one energy source (Dominkovic et al., 2016; Galvan et al., 2022; Kanugrahan et al., 2022; Pradhan et al., 2022), and deploying energy storage systems. These include battery, thermal, compressed air, hydropower, and hydrogen storage technologies (Gulagi et al., 2017; Gulagi et al., 2018; Gaeta et al., 2021; Manjong et al., 2021; Zhang Y. et al., 2022; Handayani et al., 2022; Wang et al., 2022).
Storage options come with their own set of challenges, with several papers questioning the financial and technological feasibility of rolling out these solutions at a mass scale (Handayani et al., 2022; Wang et al., 2022; Zhai et al., 2023). An alternative, seen in Lugovoy et al. (2021a) and Lugovoy et al. (2021b) in studies on China and India respectively, involves the development of a long-distance grid to provide balancing where the renewable energy supply differs between regions. The creation of long-distance grids could prove challenging in areas where they would need to span multiple countries due to the political cooperation and investment involved. Such synchronous grids have already been developed however: the Continental Europe Synchronous Area provides power to over 400 million customers across Europe and several North African countries via an AC link under the Strait of Gibraltar. Synchrony was last year expanded to Ukraine and Moldova following the 2022 Russian invasion of Ukraine (ENTSO-E, 2023).
The studies on wind and solar energy reviewed frequently emphasise the importance of reducing energy demand in conjunction to achieve net-zero targets. Rogelj et al. (2015) state this unambiguously: “returning warming to below 1.5°C by 2,100 becomes infeasible if final energy demand is not kept to very low levels.”
Three forms of hydropower are discussed in the literature: conventional dammed hydropower, run-of-river, and pumped-storage. As a renewable energy source, hydropower is more geographically constrained than wind or solar energy. This is evident in the smaller pool of academic literature in comparison to that on variable renewables and in the wide variance in potential contributions within the academic literature. For resource-rich regions, such as China’s Sichuan Province, hydropower can provide the bulk of all electricity generation (Bamisile et al., 2022; Wang et al., 2022), while the contributions remain more modest elsewhere. Financial and construction timelines are other areas that may limit hydropower expansion relative to variable renewables, as highlighted by Majong et al. (2021).
With relatively few hydropower resources, the technology does not feature heavily in the UK-focused scenarios developed by the CCC, BEIS, or National Grid within the reviewed grey literature (being grouped under “other renewables” in data tables from the CCC and National Grid).
In addition to the potential for significant contributions to energy generation and capacity (Table 5), studies highlight two key properties that make hydropower an important asset. These are the continuity of its supply, making it suitable for providing zero-carbon baseload generation, and the ability to store and rapidly dispatch energy in load-balancing applications as pumped storage hydropower (PSH).
Aghahosseini et al. (2018) assess PSH potential in designing a 100% renewable energy system for Iran, as do Gulagi et al. (2018) for India, and Wang et al. (2022) for China’s Sichuan Province. Gaeta et al. (2021) conclude that a total of 17 GW of PSH capacity could be developed in Italy by 2050 (compared to battery capacity of 28–38 GW), while Handayani et al. (2022) model PHS providing 15.8 GW of capacity (of 41.8 GW total) within the Association of Southeast Asian Nations. Galvan et al. (2022) prescribe PHS a 17% share of the energy storage mix in South America by 2050.
Dominkovic et al. (2016) demonstrate the load-balancing role of hydropower in an hourly simulation of a zero-carbon energy system for Southeast Europe. They show that dammed hydropower provides the greatest proportion of energy during evenings year-round and during summer nights, as PV generation falls at these times. The same study warns of more intermittent hydropower production in the future due to climate change reducing the accumulation of snow, a steady source of meltwater.
Academic literature on nuclear energy was limited to six studies: five looking at fission, and one at fusion. Details on potential contribution (Table 6) show nuclear to make consistently large contributions to a decarbonised energy supply.
Grey literature showed nuclear fission to be a continuing feature of the UK’s energy mix throughout the decarbonisation process. The CCC’s Balanced Net Zero pathway models 74.5 TWh of nuclear fission generation in 2050 (approximately 10% of a 748.9 TWh total), with the National Grid scenarios (2021) allocating 33.2–87.7 TWh (of 701.0–822.7 TWh totals) of generation to nuclear fission. BEIS’ Clean Growth Strategy (2017) further discusses the future of nuclear in the UK, pledging £460 million to support development.
Considering the continued reliance on nuclear fission in policy-relevant net-zero strategies–over 10% of generation in some scenarios (CCC, 2020; National Grid, 2021)–and the existing share of nuclear in the energy mix [21% in the UK (BEIS, 2017)], the limited coverage within the assessed academic literature is noteworthy. This is particularly true of academic studies focusing on the UK, which despite being the most numerous overall, only contributed a single study (Price et al., 2023).
Gi et al. (2020) provide the only study on fusion, concluding that rollout would be limited to a handful of countries where the potential of cost-efficient renewable sources was limited. As fusion remains several decades from reaching commercial viability [the IAEA (2023); UKAEA (2023) targeting 2040 for the completion of prototypes], more assumptions are made (i.e., on capital cost, annual expenses, fuel and back-end costs) versus studies on mature renewable technologies. These findings therefore come with a significant level of uncertainty, which the authors acknowledge. Further, the authors highlight barriers to fusion including the availability of lithium and waste management strategies.
Cost-competitiveness of nuclear versus renewables was further investigated by Schreyer et al. (2020), finding that Japan was alone among the industrialised economies of the EU, US, and Australia in seeing increased costs of electricity under a modelled fission phase-out by 2040. The necessity of nuclear in designing a cost-efficient net-zero energy system for Japan was not a universal finding, with Oshiro et al. (2018) arguing that BECCS would be a suitable substitute. Beyond Japan, a study of net-zero energy systems for the UK from Price et al. (2023) finds that expanding existing fission capacity is only cost-effective under scenarios where BECCS and energy storage are unavailable, expansion of European interconnectors is prohibited, and cost and construction times of nuclear are set to their most ambitious levels.
Fission comes with additional issues and limitations, with Hong et al. (2015) highlighting problems including sourcing an adequate supply of fissionable material, the management of waste, concerns over operational safety, and worries regarding nuclear proliferation. Overcoming such problems is likely to necessitate strong international cooperation, which may come with its own set of challenges. Additionally, Pilpola et al.’s (2019) techno-economic analysis of Finland finds that nuclear and wind are somewhat exclusionary of each other. The authors identify a trade-off in the levels of each, with high penetration levels of one in the energy mix precluding the other from achieving similar levels. Conversely, Hong et al. (2015) argue that utilising nuclear (over renewables) as dispatchable energy will accelerate the increase in total share held by zero-emissions technologies collectively and reduce the associated capital investment needed, while also reducing the total land area required for zero-carbon infrastructure.
Academic literature on hydrogen focused on two themes: assessments of energy supply potential, and analyses of emissions from its production. The literature framing supply potential within the context of an energy mix showed hydrogen to be a significant contributor, being a vector for a quarter of Japan’s energy supply, for example, (Ozawa et al., 2022). While several studies model hydrogen potential, they do not provide clear context on how that contribution fits into the total generation and/or capacity mix of the chosen study area (Martinez-Gordon et al., 2020; Fazeli et al., 2022; Vats and Mathur, 2022). This is a recurring issue across the academic literature, presenting difficulties in understanding the relative scale of the mitigation contribution being made. Further supply contributions outlined in the academic literature include its capability of supplanting natural gas entirely in Australia (Gurieff et al., 2021) and in decarbonising hard-to-abate sectors in China (Yang et al., 2022).
On emissions analysis, Valente et al. (2020) provide a comparison of blue and green hydrogen options. Among the renewable options, they find little difference in carbon emissions between biomass gasification and wind electrolysis, both stable around zero. With Spain as a case study, they find that grid electrolysis becomes a lower emitter than conventional steam methane reformed (SMR) hydrogen as the renewable share of the grid increases. Grid emission factors decrease from 0.514 kg CO2eq/KWh in 2020 to 0.151 CO2eq/KWh in 2050, resulting in grid hydrogen emissions falling to 7 kg CO2eq/kg H2 in 2050, versus the 10 kg CO2eq/kg H2 of SMR hydrogen. Inal, Zincir and Deniz (2022) state similar findings globally, with hydrogen via wind and solar electrolysis currently emitting far less than that produced using grid electricity.
International findings on supply and emissions (Table 7) are comparable to the UK-focused strategies of the National Grid’s FES (2021). Here, hydrogen is positioned as a necessary component across various pathways to provide security in electricity supply. Maximizing renewable energy utility by using surplus electricity during low-demand periods to produce hydrogen via electrolysis is emphasized. Sectoral demand varies based on scenario, except for aviation and shipping which is forecast to demand 80 TWh by 2050 under all net-zero pathways. This contrasts with the CCC’s “Hydrogen in a low-carbon economy” report (2018), which argues that hydrogen should be used more selectively. Their modelling suggests that the opportunity to utilise surplus electricity for hydrogen electrolysis will be more limited and warn against falling into something of a sunk-cost fallacy regarding utilising the existing gas network, versus alternative decarbonisation strategies.
As hydrogen is an emerging technology, feasibility was a prominent area of research. Gurieff et al. (2021) argue that it will be technically and economically feasible to blend 10% hydrogen into eastern Australia’s gas network by 2030, with complete replacement by 2040. They emphasise the advantages hydrogen holds over direct electrification, being its seasonal storage capacity and ability to be transported for the ammonia and steel production industries. Within the grey literature the National Grid’s FES highlight these benefits, as well as barriers including the lack of a pre-existing hydrogen market and the need to upgrade gas infrastructure. Economic feasibility is discussed in detail by Yang et al. (2022), concluding that green hydrogen will become more economically competitive than blue hydrogen only after 2030 in China. Fazeli, Beck and Stocks (2022) further emphasise the cost barriers to the proliferation of green hydrogen.
Discussion of bioenergy in the literature includes its application both with and without point-source carbon capture technology. Two key advantages of bioenergy with carbon capture and storage (BECCS) were stressed. Firstly, its ability to be utilised as a dispatchable energy source in place of fossil-fuels, and secondly, its potential to provide net-negative emissions. Table 8 details potential contributions from bioenergy, in terms of both energy supply and negative emissions. Unless stated otherwise within the table, these contributions come from BECCS plants.
These supply contributions are highly variable, and dependent on assumptions of future land use, investment, research and development, and sustainability (regarding energy crop competition with food crops, and the conservation/growth of forests and peatland). Several studies (Manjong et al., 2021; Handayani et al., 2022; Kanugrahan et al., 2022; Ozawa et al., 2022) place bioenergy supply below 5% of their total energy mix, while others (Cossutta et al., 2021; Bamisile et al., 2022; Scheepers et al., 2022) identify much more significant contributions. Within UK-focused grey literature, contributions tend to be at the more reserved end of those found in academic literature. The CCC’s Balanced Net Zero Pathway (2020) models 22.4 TWh (of 748.9 TWh total) generation from BECCS in 2050, supported by 0.7 million hectares of perennial energy crops (up from 0.01 Mha in 2019). The level of deployment in this scenario is dictated by the residual emissions that need to be offset to reach net-zero, rather than seeking to maximise negative emissions potential. The National Grid FES (2021) model 35.9–53.4 TWh (of 701.0–822.7 TWh totals), using the CCC’s land use assumptions. Only passing comments are made on BECCS in BEIS’ Clean Growth Strategy (2017), with bioenergy mostly being discussed in heating and transportation applications.
Studies have tried to quantify the negative emissions potential of BECCS. Alcalde et al. (2018) model BECCS as part of a package of technologies to achieve carbon neutrality in Scotland, with removals reaching −8.81 MtCO2/yr, when 0.2 Mha (of Scotland’s 1.96 Mha of suitable land) are assigned to energy crops. This is in the context of 41.89 MtCO2 total emissions in 2014. Cossutta et al. (2021) model UK-wide negative emissions from BECCS at −30.7 MtCO2e/year in 2050, while Kato and Kurosawa (2021) simulate −120 MtCO2/yr in Japan in 2050, where upper limits on biomass availability set to 1500 PJ/yr. Negative emissions from BECCS are in many cases an essential component of national net-zero strategies. The Kato and Kurosawa study finds no feasible solution for Japan to reach net-zero targets without the deployment of BECCS or another NET. In the grey literature, the National Grid’s FES states that without BECCS, “meeting net zero [in the UK] will be very challenging.” The CCC’s Sixth Carbon Budget (2020) also emphasises negative emissions potential, the budget’s Balanced Pathway modelling removals of 53 MtCO2/yr by 2050. While limited in number, several of the studies in the preceding subsections do provide a pathway to reach net-zero without the use of bioenergy (e.g., Solomon et al., 2018; Lugovoy et al., 2021b; Pradhan et al., 2022).
Geothermal featured in four studies (Table 9). While these contributions are relatively small, the utility of geothermal in increasing the diversity and security of an energy system is highlighted (Aghahosseini et al., 2018). Furthermore, Dominkovic et al. (2016) emphasise the importance of the direct application of geothermal heat in successfully decarbonising the heating sector in their study of a zero-carbon energy system for Southeast Europe.
Within the UK-focusing grey literature, contributions from geothermal were not explicitly quantified, being grouped under “other renewables,” as with hydropower. Much like hydropower, geothermal is a geographically constrained renewable, which may partially explain the limited coverage it receives in both academic and grey literature.
Five papers assessed the mitigation potential of behavioural transitions (Table 10). These options encompass a broad range of scenarios and overlap with specific demand-side sectors, such as transport and buildings. Where overlap exists, approaches are classed as behavioural transitions when the focus is placed on actions taken by individuals or organisations to reduce their CO2 emissions, as opposed to policy options or technological transitions designed to reduce energy demand. Brand et al. (2020), for example, discuss both behavioural transitions in transport (changes in travel patterns, occupancy levels, travel mode) which are detailed here, and transport-specific policy options (phase-out of fossil-fuel vehicles) which are covered in the transport-specific subsection.
Literature emphasised that technological options alone will not suffice in reaching net-zero by 2050. Costa et al. (2021) conclude that behavioural transitions in combination with technological changes can help realise net-zero goals in Europe by 2040, whereas relying on technology alone delays that to 2050. Several authors argued that an over-reliance on technology would limit the co-benefits of decarbonisation. Brand et al. (2020) highlight this in improved road safety and transport equality from the uptake of shared and multi-modal mobility, Costa et al. emphasise the reduced pressure on natural resources, and Garvey et al. (2021) the public health benefits of low-carbon dietary transitions. Multiple studies (Koide et al., 2021; Wiest et al., 2022) also addressed the feasibility of bringing about such change. The former showed that the needed uptake of behavioural changes to meet net-zero ranged from 62% to 87% of the population in a study covering 52 Japanese cities, while the later concede that it is unrealistic for all European citizens to adopt their proposed lifestyle changes.
The relative lack of academic literature on behavioural transitions is surprising for several reasons. Firstly, the five papers reviewed demonstrate sizeable mitigation potential. Secondly, behavioural transitions encompass a plethora of avenues for research to pursue. Thirdly, behavioural transitions feature prominently in the UK-focusing grey literature. The National Grid FES (2021) build one of their three net-zero pathways around changes in consumer behaviour, for example,. The CCC’s Sixth Carbon Budget (2020) positions behavioural transitions as a central feature in their Widespread Engagement scenario, and to varying degrees in all other net-zero scenarios. The Clean Growth Strategy (BEIS, 2017) targets behaviour change in reducing emissions from transport and buildings, and CREDS (2021) hold it as a key component in reducing energy demand in the UK’s transition to net-zero.
The lack of academic literature on behavioural transitions may partially be a result of research not being captured by the search terms, which prescribe reference to “zero-carbon” or “net-zero” in the title, abstract, or keywords of an article. These were selected as the framing of this review places mitigation options within the context of their contribution towards net-zero. While unlikely to be zero-carbon when considered alone, behavioural options such as dietary change can play a role in reaching net-zero but would not be captured in this search unless explicitly highlighted in one of the search fields.
Two papers focused on public transport, one on personal transport, and two on a combination of both. The emissions reduction potential in this selection of literature was significant. In the United Kingdom, Brand et al. (2020) model tailpipe CO2 reductions from personal vehicles of up to 100% under scenarios phasing-out the sale of conventional fossil-fuel vehicles, Logan et al. (2020a) achieve 91% CO2 emission reductions in the bus fleet through switching to electric and hydrogen buses, and Logan et al. (2020b) an 89% reduction in rail through adopting electric trains. These studies make several important assumptions regarding feasibility. Brand et al.’s higher ambition scenarios require increasingly efficient batteries and charging infrastructure, and much-improved market conditions and consumer awareness. The Logan et al. studies require increasingly ambitious supply-side decarbonisation to reach their upper emission reduction levels from electric alternatives and accelerated technological development to meet demand for hydrogen options.
In other areas of the world, these reductions remain significant though less substantial–Bataille et al. (2020) identify potential reductions in passenger transport energy demand of between 14% (Peru) and 62% (Costa Rica) in Latin America, and Bu et al. (2021) reductions of 42% in China. While useful in understanding the degree of sectoral-level decarbonisation, these reductions are often not placed within the broader context of the total emissions reduction necessary to meet net-zero overall in their study area. This presents an obstacle to understanding how significant these reductions are at a broader scale.
Grey literature positions transport as a key area for carbon abatement. The Clean Growth Strategy (BEIS, 2017) apportions 24% of the UK’s emissions to this sector, or 113 MtCO2e/year, per the National Grid (2021). The CCC’s Sixth Carbon Budget reduces these emissions to 1 MtCO2e/year by 2050, primarily through the adoption of zero-emission vehicles, aided by demand reduction. Reducing transport demand is a component of CREDS’ 2021 report on the role of demand reduction in achieving net-zero in the UK, with their modelling producing a reduction of 68% by 2050.
Given this ambition, it is noteworthy that this area received relatively little attention. As with the subsection on behavioural transitions, this may be a result of the search terms used. Use of the phrasing “zero-emissions” is commonplace with vehicles, for example, and would not be captured by the search terms if the title, abstract or keywords of the article did not place them within the context of reaching “zero-carbon” or “net-zero.”
Some common barriers to transport decarbonisation were identified in the literature listed in Table 11. Despite their potential, many papers noted the difficulties that may be encountered in increasing EV uptake. These include constraints stemming from the availability of materials, the reach of the charging network, storage capacity of the vehicle battery, and affordability. This is particularly true of less wealthy areas of the world, as Bu et al. (2021) show in a study of various regions in China, and Bataille et al.’s (2020) study in South America. Several papers also acknowledged the timescale for the roll-out of zero-carbon vehicles and thus stressed the continued importance of reducing emissions from conventional vehicles.
Academic literature highlighted the built environment’s significant share of total energy demand (Table 12), with Roach and Ugursal (2021) estimating demand from the residential sector alone at 17% of Canada’s total, for example,. Contributions were either described in terms of emission reductions or energy demand reductions. Built environment emission reduction potential was significant, reaching 94% in Allen et al.’s (2022) study on Australia and 84% in Li et al.’s (2022) study on the UK. Findings on energy demand reduction were also promising, with falls of between 60% and 65% possible in China, Germany and Japan (Hu and Qui, 2019; Shimoda et al., 2021).
TABLE 12. Contribution from the decarbonisation of the built environment across assessed literature.
These academic findings on emission reduction potential, within the UK and elsewhere, track well with assessments made in the UK-focusing grey literature. The CCC’s Sixth Carbon Budget (2020) show buildings to be the UK’s third biggest emitter, ahead of electricity supply, agriculture and land use, and waste and F-gases. In contrast with the academic literature, their pathways show that it is possible to reduce emissions from residential buildings to near-zero (0.04 MtCO2e) by 2050, at the latest. This is achieved through the use of hydrogen for heat and behavioural changes in occupants, in addition to the retrofit and efficiency improvements assessed in the Li et al. (2022) study. The National Grid FES (2021) echo this, their pathway reducing residential emissions to 1 MtCO2e in 2050. Further, CREDS’ demand reduction report (2021) models total energy demand reductions of up to 52% in residential buildings and 48% in non-domestic buildings, driven by retrofit, digitalisation, and behavioural change. These energy demand reductions are comparable to the findings from Hu and Qui (2019) and Shimoda et al. (2021) for China, Germany, and Japan.
While significant contributions can be made from improvements in this sector, barriers exist in implementing them. This is particularly true in warmer climates, where buildings must maintain thermal comfort while contending with intense summer temperatures, recurrent heatwaves, and an exacerbated urban heat island effect (IEA, 2018). Attia et al. (2017) discuss these challenges in the geographic context of southern Europe, finding that current practices and designs are failing to incorporate new materials and concepts, resulting in many residential and commercial buildings experiencing summer conditions well beyond even the most tolerant comfort range. The authors point to an over-reliance on steady-state simulations in building design and construction, neglecting to employ laboratory and field measurements, or real-world performance data.
As building energy codes vary substantially between countries, studies projecting energy demand based upon them are likely to exhibit considerable variance depending on the study area. In investigating this, Hu and Qui (2019) perform a comparison between the building energy codes and policies of the United States, China, and Germany. They find that while China’s regulations are much less stringent than those of the United States or Germany, the United States has the greatest energy intensity. This was due to different energy use operational schedules, driven by user behaviour and cultural contexts (chiefly, differing thermal comfort ranges). Germany, with its short operational schedules similar to China and stringent energy codes akin to the United States, exhibits the highest potential for achieving the transformation of its housing stock into net-zero energy buildings (NZEBs). These findings suggest that strengthening regulations governing NZEBs, particularly with elements promoting behavioural change, can enhance the contributions of the built environment towards achieving net-zero goals.
The pool of academic literature focused on the industrial sector’s transition to net-zero was limited to the studies detailed in Table 13. Garvey et al. (2022) and Zhang S. et al. (2022) assess the steel and iron industries of the UK and China respectively, modelling high levels of emissions reduction, principally through electrification of the processes involved. Ren et al. (2023) and Watari et al. (2022) focus on the cement and concrete sectors, with less reduction potential being found on the demand-side of these processes. Demand-side measures here revolve around reducing demand for the product (be that concrete, cement, iron, or steel) or decreasing the energy and/or carbon intensity of the production process. None of the studies achieve net-zero solely through these measures, as several industrial processes emit CO2 directly as a by-product of raw materials used (Kuramochi et al., 2012), highlighting the varied requirements for CCS technology to attain net-zero.
Industry is a high-pollution sector in the UK, accounting for 13% of emissions in 2020 (Grid, 2021). It is also one of the most difficult sectors to decarbonise, as evident in the grey literature. Amongst CREDS’ scenarios for demand reduction (2021), industrial energy consumption falls by just 26% under the most ambitious 2050 pathway (by comparison, total energy consumption falls by 52%). The CCC’s Balanced Net Zero Pathway for the manufacturing and construction sector reduces emissions from 62.3 MtCO2e in 2020 to 2.81 MtCO2e, with the main abatement sources being the use of hydrogen, electrification, and CCS deployment. The need for CCS to reduce direct emissions from industrial processes is also identified in BEIS’ Clean Growth Strategy (2017), which shows it to be the largest source of industrial carbon abatement. These findings track well with those of the academic literature.
As with transport and behavioural transitions, the lack of academic coverage of this area is surprising given the scale of the challenge in reaching industrial net-zero. Like those sections, this may be a result of the explicit net-zero framing of this review. In many cases, industrial decarbonisation studies that exclude CCS are unlikely to achieve net-zero. While their findings may still be relevant to reaching that goal, they may not be accounted for unless framed within net-zero terminology.
The potential contributions from nature-based solutions in achieving net-zero are shown in Table 14. The global assessments (Dooley et al., 2022; Matthews et al., 2022) found significant potential, with NBS’ capable of sequestering approximately 2 and 3 years’ worth of global annual CO2 emissions (2020 levels) by 2050 and 2100 respectively–though in the Matthews et al. study, this is returned to the atmosphere in the latter half of the century. In the UK, Yumashev et al. (2022) estimate that a year’s worth of 2020-level CO2e could be sequestered by 2100, with Bradfer-Lawrence et al. (2022) going further, estimating 3 years’ worth of CO2e sequestration by the end of the century.
Nature-based solutions play an important role in the UK-focusing grey literature. The CCC’s Balanced Net Zero Pathway estimates removals of 38.5 MtCO2/yr in 2050 to mitigate the significant residual emissions from aviation and agriculture. Similarly, the National Grid’s FES (2021) model removals of 33 MtCO2/yr in 2050 in their Leading the Way net-zero scenario. The Green Alliance also show significant mitigation potential from NBS’, building on the assumptions of the CCC’s “high biomass/natural peatland” scenario in their report “Cutting the climate impact of land use” (2019). An increase in sequestration of 90%, from 13.4 MtCO2e/yr in 2016 to 25.4 MtCO2e/year in 2030, is modelled, through a combination of increased woodland planting and agroforestry, salt marsh restoration, and enhanced soil sequestration. This is supplemented by a 4.8 MtCO2e/year reduction in emissions from peatland and a 10.4 MtCO2e/year cut in agricultural emissions. The Clean Growth Strategy (BEIS, 2017) sets out several policy proposals to facilitate investment in natural capital to support this expansion of nature-based solutions.
Though the contributions from NBS’ are often sizeable, academic literature stresses that they do not nullify the need for drastic emissions reductions. Cong et al.’s (2022) study of NBS’ in Japan’s Fukuoka Prefecture, for example, finds achieving net-zero to be impossible without additional efforts on emissions reduction. Both Costa et al. (2021) and Matthews et al. (2022) argue that carbon removal via NBS’ is only comparable to emissions reductions when that carbon is permanently sequestered, which is often not guaranteed.
Trade-offs between carbon sequestration and the co-benefits of NBS’ are also highlighted, with Martes and Köhl (2022) noting that their maximum-sequestration scenario would result in the disruption of ecosystem services provided by forests. The trade-off between reforestation and the need to meet growing food demand is also highlighted. As with many of the other mitigation options reviewed, cost presents another barrier–Dooley et al. (2022) estimates a potential tripling of investment in NBS’ necessary by 2030, while Costa et al. (2021) state that only around 50% of the mitigation potential of existing agriculture-related solutions are cost-effective today.
Direct air capture (DAC) is a negative emissions technology which captures CO2 directly from ambient air, rather than at point sources (such as in the bioenergy sector, as discussed in Section 3.2.1). This technology is in an early stage of development, with this reflected in the more limited number of studies on its potential, versus established mitigation options such as wind and solar energy (Alcadle et al., 2018). Nonetheless, those studies that are available suggest it to be capable of meaningful contributions (Table 14).
The two Japanese studies show varying levels of capture potential, with Kato and Kurosawa (2021) estimating −130 MtCO2/yr in 2070, and Ozawa et al. (2022) approximately −203 MtCO2/yr in 2050. This compares against Japan’s total emissions of 1,150 MtCO2 in 2020, representing a significant reduction. The Kato and Kurosawa study achieves these reductions in scenarios which do not employ BECCS–where BECCS is available, these reductions are approximately −35 MtCO2/yr, with assumptions on energy consumption from DAC set to 5.25 GJ/tCO2 abated within the TIMES-Japan model. By comparison, the Ozawa et al. study uses a slightly higher energy consumption assumption of 5.97 GJ/tCO2, within the related AIST-MARKAL model. Wohland et al. (2018) meanwhile model a 300 GW capacity DAC system capable of capturing 500 MtCO2/yr in Europe, which compares to EU total emissions of 2,730 MtCO2 in 2021.
Within the UK-focused grey literature, the National Grid’s FES (2021) is much more conservative regarding the deployment of DAC than those assessments made within academic literature. Their Leading the Way pathways models removals of 15 MtCO2/yr in 2050, while it does not feature at all in their other two net-zero pathways. Removals are constrained further still in the CCC’s Balanced Net Zero Pathway, with just 5 MtCO2/yr in 2050. In BEIS’ Clean Growth Strategy, DAC receives just a single passing mention. While the study area is different to those in the academic literature, these differences in potential contribution remain noteworthy due to their size alone.
As to be expected with a technology in its infancy, there currently exist barriers to its wider deployment. Alcalde et al. (2018) note the high energy requirements involved with deploying DAC, these ranging from 1 to 13 GJ/tCO2 abated–emphasising the need to decarbonise and expand the energy supply to power DAC technologies. Cost-competitiveness is highlighted by Becattini et al. (2021), who find that DAC abatement costs are expected to be significantly higher than those of point source capture NETs. The authors also stress the unknowns surrounding the technology, such as the upper limits of its emission mitigation potential, due to the early stage of development it is in. These barriers offer an explanation for the notable difference in contribution DAC makes between the academic and grey literature. The CCC’s Balanced Net Zero Pathway accounts for the need to overcome these barriers and demonstrate DAC at scale, before beginning to scale-up the technology in the 2040s. The final level of deployment is also dictated by the scale of residual emissions which need to be offset. The academic papers all take different approaches which account for their higher findings on potential from technology, either looking at maximising the theoretical contribution from DAC, modelling DAC deployment to bridge a significantly larger residual emissions gap, while often not explicitly considering the timeline needed to demonstrate and scale-up DAC.
This systematic review sought to unpack the literature on zero-carbon transitions to answer two key questions.
• Firstly, what were the most prevalent options discussed within zero-carbon literature, and how do the geographic scales and focus of research vary between those different options?
• Secondly, what insights can be drawn from the literature on the feasibility, barriers, and potential contributions of these options towards meeting net-zero?
Regarding the former, the analysis demonstrates that the balance of literature favours supply-side research. Studies on demand-side mitigation totalled just half that of those focusing on the supply-side. Research on solar and wind energy led the way in supply-side literature, with the relative lack of nuclear coverage noteworthy given the 10% contribution it currently makes to global electricity generation (IEA, 2022a, p. 294). This is perhaps a reflection of a belief that nuclear will play a lesser role in the future, considering the gradual decrease in total share the technology has held over the past decade, compared to the expansion of renewables (IEA, 2022b).
Within demand-side literature, research on the built environment was by far the most prevalent, with studies on behavioural change, transport transitions, and industrial decarbonisation totalling between just four and five papers apiece. This may in part be a result of the framing of literature in this space. Net-zero terminology (i.e., “net-zero energy buildings” and related nomenclature) is particularly commonplace within the context of decarbonising the built environment (Sartori et al., 2012; Voss et al., 2013), which may have lent itself to returning more results in the literature search in comparison to other demand-side options.
In terms of the geographic scale of the research, the current pool of literature was dominated by national-level studies (50 of 85), with sub-national studies the least common, accounting for just eight academic papers. Within these eight, just two selected study areas at a level below the principal administrative division, such as a city or metropolitan area. Very little in the way of a geographic focus was found in the mitigation options assessed, with the exceptions to this being solar–where over half of the literature focused on sunbelt countries–and hydropower–with China featuring in four of eight studies.
Given the above, it can be concluded that two key areas would benefit from further research–that focusing on demand-side mitigation, and that carrying research out at a sub-national scale, particularly at a local administrative level.
Concerning the second question, the academic literature typically positioned solar and wind energy as the principal components of a decarbonised energy system. This finding was common across a wide range of study areas from across the world and compared well to those from the UK-focusing grey literature, which found the two combined to account for the vast majority of the UK’s energy supply come 2050. Findings on the next most prevalent option within the supply-side academic literature–bioenergy–showed some interesting differences with the grey literature, which was comparatively much more reserved regarding potential contributions. This appears to largely be a result of the grey literature limiting the deployment of bioenergy with CCS to offset residual emissions in their scenarios, whereas much of the academic literature seeks to maximise the potential contribution from the technology. This discrepancy in contribution between academic and grey literature was also identified in part of the work on hydrogen. Nuclear proved another area where differences in the academic and grey literature emerged. Within academia, relatively little engagement with nuclear was identified, featuring in just six studies. In the grey literature however, nuclear power was still relied upon to provide approximately 10% of the UK’s energy supply in 2050, and significant investments in this area were pledged.
Findings on potential contributions within the demand-side academic literature were generally comparable to those within the UK-focused grey literature. The takeaway from this portion of the work was that while the contributions from demand-side decarbonisation were consistently significant, in both academic and grey literature, they receive very little coverage within the academic pool of literature compared to options on the supply-side. Transport, for example, is assessed to account for nearly a quarter of the UK’s emissions, with literature finding substantial reductions in this sector possible. Despite the clear importance of this sector, just four of the 85 academic studies specifically focused on it. This finding applies broadly to the research on demand-side behavioural transitions and industrial decarbonisation additionally, representing a potential gap in academia’s contribution towards net-zero policy.
This paper focused on literature explicitly framed within the context of the “transition” (and related terms, see Table 1) to zero carbon. As such, it should be stressed that the quantification of climate mitigation potential of the assessed options pertains only to the body of literature which discusses them within this framing. The results discussed in this paper do not provide a comprehensive synthesis of all literature discussing the climate mitigation potential of any given option, as much does so outside of this framing.
Providing an analysis of the climate mitigation potential of the options identified within this body of literature was one of the key objectives of this research. This was performed through a quantification of the potential contribution to reaching net-zero described in each study, either through the amount of energy supplied, the level of demand reduced, or the quantity of carbon sequestered. As noted in the discussion, in many instances there were significant differences in the assessments of potential contribution in the literature on a given mitigation option. This invites further research and analysis of the models used and assumptions made by studies in this area, and how these factors may influence results.
Pursuing these research gaps in the literature will help reach a better understanding of the role climate change mitigation options will play in the transition to zero-carbon, and further ensure that policymakers receive the data necessary to chart a course to a zero-carbon future.
AS: Writing–original draft. SM: Writing–review and editing. BP: Writing–review and editing. RW: Writing–review and editing.
The author(s) declare financial support was received for the research, authorship, and/or publication of this article. ESRC funded Centre for Climate Change and Social Transformations (CAST), Grant number ES/S012257/1.
The authors declare that the research was conducted in the absence of any commercial or financial relationships that could be construed as a potential conflict of interest.
All claims expressed in this article are solely those of the authors and do not necessarily represent those of their affiliated organizations, or those of the publisher, the editors and the reviewers. Any product that may be evaluated in this article, or claim that may be made by its manufacturer, is not guaranteed or endorsed by the publisher.
The Supplementary Material for this article can be found online at: https://www.frontiersin.org/articles/10.3389/fenrg.2023.1268270/full#supplementary-material
Aghahosseini, A., Bogdanov, D., Ghorbani, N., and Breyer, C. (2018). Analysis of 100% renewable energy for Iran in 2030: integrating solar PV, wind energy and storage. Int. J. Environ. Sci. Technol. 15 (1), 17–36. doi:10.1007/s13762-017-1373-4
Alcalde, J., Smith, P., Haszeldine, R. S., and Bond, C. E. (2018). The potential for implementation of negative emission technologies in Scotland. Int. J. Greenh. Gas Control 76, 85–91. doi:10.1016/j.ijggc.2018.06.021
Allen, C., Oldfield, P., Teh, S. H., Wiedmann, T., Langdon, S., Yu, M., et al. (2022). Modelling ambitious climate mitigation pathways for Australia’s built environment. Sustain. Cities Soc. 77, 103554. doi:10.1016/j.scs.2021.103554
Attia, S., Eleftheriou, P., Xeni, F., Morlot, R., Ménézo, C., Kostopoulos, V., et al. (2017). Overview and future challenges of nearly zero energy buildings (nZEB) design in Southern Europe. Energy Build. 155, 439–458. doi:10.1016/j.enbuild.2017.09.043
Bamisile, O., Wang, X., Adun, H., Joseph Ejiyi, C., Obiora, S., Huang, Q., et al. (2022). A 2030 and 2050 feasible/sustainable decarbonization perusal for China’s sichuan province: a deep carbon neutrality analysis and EnergyPLAN, 261. Energy Conversion and Management. doi:10.1016/j.enconman.2022.115605
Barthelmie, R. J., and Pryor, S. C. (2021). Climate change mitigation potential of wind energy. Climate 9 (9), 136. doi:10.3390/cli9090136
Bataille, C., Waisman, H., Briand, Y., Svensson, J., Vogt-Schilb, A., Jaramillo, M., et al. (2020). Net-zero deep decarbonization pathways in Latin America: challenges and opportunities. Energy Strategy Rev. 30, 100510. doi:10.1016/j.esr.2020.100510
Becattini, V., Gabrielli, P., and Mazzotti, M. (2021). Role of carbon capture, storage, and utilization to enable a Net-Zero-CO2-emissions aviation sector. Industrial Eng. Chem. Res. 60 (18), 6848–6862. doi:10.1021/acs.iecr.0c05392
BEIS (2021). Net zero strategy: build back greener. Available at: https://www.gov.uk/government/publications/net-zero-strategy.
BP Sustainability Report 2020 (2021). Reimagining energy for people and our planet, 1–97. Available at: https://www.bp.com/content/dam/bp/business-sites/en/global/corporate/pdfs/sustainability/group-reports/bp-sustainability-report-2020.pdf.
Bradfer-Lawrence, T., Finch, T., Bradbury, R. B., Buchanan, G. M., Midgley, A., and Field, R. H. (2022). The potential contribution of terrestrial nature-based solutions to a national ‘net zero’ climate target. J. Appl. Ecol. 58 (11), 2349–2360. doi:10.1111/1365-2664.14003
Brand, C., Anable, J., Ketsopoulou, I., and Watson, J. (2020). Road to zero or road to nowhere? Disrupting transport and energy in a zero carbon world. Energy Policy 139, 111334. doi:10.1016/j.enpol.2020.111334
Bu, C., Cui, X., Li, R., Li, J., Zhang, Y., Wang, C., et al. (2021). Achieving net-zero emissions in China’s passenger transport sector through regionally tailored mitigation strategies. Appl. Energy 284, 116265. doi:10.1016/j.apenergy.2020.116265
Cong, R., Fujiyama, A., and Matsumoto, T. (2022). Carbon sink quantification aids for achieving the zero-emission goal: a case study in Japan. Energy Rep. 8, 8–17. doi:10.1016/j.egyr.2022.06.102
Cossutta, M., Foo, D. C. Y., and Tan, R. R. (2021). Carbon emission pinch analysis (CEPA) for planning the decarbonization of the UK power sector. Sustain. Prod. Consum. 25, 259–270. doi:10.1016/j.spc.2020.08.013
Costa, L., Moreau, V., Thurm, B., Yu, W., Clora, F., Baudry, G., et al. (2021). The decarbonisation of Europe powered by lifestyle changes. Environ. Res. Lett. 16 (4), 044057. doi:10.1088/1748-9326/abe890
Creutzig, F., Roy, J., Lamb, W. F., Azevedo, I. M., Bruine de Bruin, W., Dalkmann, H., et al. (2018). Towards demand-side solutions for mitigating climate change. Nat. Clim. Change 8 (4), 260–263. doi:10.1038/s41558-018-0121-1
Dominković, D. F., Bačeković, I., Ćosić, B., Krajačić, G., Pukšec, T., Duić, N., et al. (2016). Zero carbon energy system of South east Europe in 2050. Appl. Energy 184, 1517–1528. doi:10.1016/j.apenergy.2016.03.046
Dooley, K., Nicholls, Z., and Meinshausen, M. (2022). Carbon removals from nature restoration are no substitute for steep emission reductions. One Earth 5 (7), 812–824. doi:10.1016/j.oneear.2022.06.002
ENTSO-E (2023). One year after the successful synchronisation of the Continental European power system with the Ukrainian and Moldovan power systems, the European TSOs together with Ukrenergo have confirmed the basis for an essential and effective cooperation contributing to the security of the interconnected power system. https://www.entsoe.eu/news/2023/03/16/synchronisation-anniversary/.
European Commission (2020). Long-term low greenhouse gas emission development strategy of the European Union and its Member States. European Commission, 1–7. Available at: http://www4.unfccc.int/submissions/INDC/Published Documents/Latvia/1/LV-03-06-EU INDC.pdf.
Fazeli, R., Beck, F. J., and Stocks, M. (2022). Recognizing the role of uncertainties in the transition to renewable hydrogen. Int. J. Hydrogen Energy 47 (65), 27896–27910. doi:10.1016/j.ijhydene.2022.06.122
Gaeta, M., Businge, C. N., and Gelmini, A. (2021). Achieving net zero emissions in Italy by 2050: challenges and opportunities. Energies 15 (1), 46. doi:10.3390/en15010046
Galván, A., Haas, J., Moreno-Leiva, S., Osorio-Aravena, J. C., Nowak, W., Palma-Benke, R., et al. (2022). Exporting sunshine: planning South America’s electricity transition with green hydrogen. Appl. Energy 325, 119569. doi:10.1016/j.apenergy.2022.119569
Garvey, A., Norman, J. B., and Barrett, J. (2022). Technology and material efficiency scenarios for net zero emissions in the UK steel sector. J. Clean. Prod. 333, 130216. doi:10.1016/j.jclepro.2021.130216
Garvey, A., Norman, J. B., Owen, A., and Barrett, J. (2021). Towards net zero nutrition: the contribution of demand-side change to mitigating UK food emissions. J. Clean. Prod. 290, 125672. doi:10.1016/j.jclepro.2020.125672
Gi, K., Sano, F., Akimoto, K., Hiwatari, R., and Tobita, K. (2020). Potential contribution of fusion power generation to low-carbon development under the Paris Agreement and associated uncertainties. ENERGY STRATEGY Rev. 27, 100432. doi:10.1016/j.esr.2019.100432
Green Alliance (2019). Cutting the climate impact of land use, what role can land use play in climate change mitigation?
Gulagi, A., Bogdanov, D., and Breyer, C. (2018). The role of storage technologies in energy transition pathways towards achieving a fully sustainable energy system for India. J. Energy Storage 17, 525–539. doi:10.1016/j.est.2017.11.012
Gulagi, A., Choudhary, P., Bogdanov, D., and Breyer, C. (2017). Electricity system based on 100% renewable energy for India and SAARC. PLoS ONE 12 (7), e0180611. doi:10.1371/journal.pone.0180611
Gurieff, N., Moghtaderi, B., Daiyan, R., and Amal, R. (2021). Gas transition: renewable hydrogen’s future in eastern Australia’s energy networks. Energies 14 (13), 3968. doi:10.3390/en14133968
Handayani, K., Anugrah, P., Goembira, F., Overland, I., Suryadi, B., and Swandaru, A. (2022). Moving beyond the NDCs: ASEAN pathways to a net-zero emissions power sector in 2050. Appl. Energy 311, 118580. doi:10.1016/j.apenergy.2022.118580
Haris, S. M., Mustafa, F. B., and Raja Ariffin, R. N. (2020). Systematic literature review of climate change governance activities of environmental nongovernmental organizations in Southeast Asia. Environ. Manag. 66 (5), 816–825. doi:10.1007/s00267-020-01355-9
Hong, S., Bradshaw, C. J. A., and Brook, B. W. (2015). Global zero-carbon energy pathways using viable mixes of nuclear and renewables. Appl. Energy 143, 451–459. doi:10.1016/j.apenergy.2015.01.006
Hu, M., and Qiu, Y. (2019). A comparison of building energy codes and policies in the USA, Germany, and China: progress toward the net-zero building goal in three countries. Clean Technol. Environ. Policy 21 (2), 291–305. doi:10.1007/s10098-018-1636-x
IAEA (2023). Fusion – frequently asked questions. Available at: https://www.iaea.org/topics/energy/fusion/faqs.
IEA (2021a). Direct air capture. Paris: IEA. Available at: https://www.iea.org/reports/direct-air-capture.
IEA (2022b). Global electricity generation mix, 2010-2020. Paris: IEA. Available at: https://www.iea.org/data-and-statistics/charts/global-electricity-generation-mix-2010-2020.
IEA (2020). Global EV outlook 2020. Paris: IEA. Available at: https://www.iea.org/reports/global-ev-outlook-2020.
IEA (2021b). Net zero by 2050. Paris: IEA. Available at: https://www.iea.org/reports/net-zero-by-2050.
IEA (2018). The future of cooling. Paris: IEA. Available at: https://www.iea.org/reports/the-future-of-cooling.
IEA (2022a). World energy outlook 2022. Paris: IEA. Available at: https://www.iea.org/reports/world-energy-outlook-2022.
Inal, O. B., Zincir, B., and Deniz, C. (2022). Investigation on the decarbonization of shipping: an approach to hydrogen and ammonia. Int. J. Hydrogen Energy 47 (45), 19888–19900. doi:10.1016/j.ijhydene.2022.01.189
IPCC (2022). Climate change 2022 - impacts, adaptation and vulnerability - summary for policymakers.
IPCC (2018). Mitigation pathways compatible with 1.5°C in the context of sustainable development. Glob. Warming 1.5°C, 93–174. doi:10.1017/9781009157940.004
Ji, L., Wu, Y., Sun, L., Zhao, X., Wang, X., Xie, Y., et al. (2022). Solar photovoltaics can help China fulfill a net-zero electricity system by 2050 even facing climate change risks. Resour. Conservation Recycl. 186, 106596. doi:10.1016/j.resconrec.2022.106596
Kanugrahan, S. P., Hakam, D. F., and Nugraha, H. (2022). Techno-economic analysis of Indonesia power generation expansion to achieve economic sustainability and net zero carbon 2050. Sustain. Switz. 14 (15), 9038. doi:10.3390/su14159038
Kato, E., and Kurosawa, A. (2021). Role of negative emissions technologies (NETs) and innovative technologies in transition of Japan’s energy systems toward net-zero CO2 emissions. Sustain. Sci. 16 (2), 463–475. doi:10.1007/s11625-021-00908-z
Kobashi, T., Yoshida, T., Yamagata, Y., Naito, K., Pfenninger, S., Say, K., et al. (2020a). On the potential of “Photovoltaics + Electric vehicles” for deep decarbonization of Kyoto’s power systems: techno-economic-social considerations. Appl. ENERGY 275, 115419. doi:10.1016/j.apenergy.2020.115419
Koide, R., Kojima, S., Nansai, K., Lettenmeier, M., Asakawa, K., Liu, C., et al. (2021). Exploring carbon footprint reduction pathways through urban lifestyle changes: a practical approach applied to Japanese cities. Environ. Res. Lett. 16 (8), 084001. doi:10.1088/1748-9326/ac0e64
Kourgiozou, V., Commin, A., Dowson, M., Rovas, D., and Mumovic, D. (2021). Scalable pathways to net zero carbon in the UK higher education sector: a systematic review of smart energy systems in university campuses. Renew. Sustain. Energy Rev. 147 (5), 111234. doi:10.1016/j.rser.2021.111234
Kuramochi, T., Ramírez, A., Turkenburg, W., and Faaij, A. (2012). Comparative assessment of CO2 capture technologies for carbon-intensive industrial processes. Prog. energy Combust. Sci. 38 (1), 87–112. doi:10.1016/j.pecs.2011.05.001
Li, X., Arbabi, H., Bennett, G., Oreszczyn, T., and Densley Tingley, D. (2022). Net zero by 2050: investigating carbon-budget compliant retrofit measures for the English housing stock. Renew. Sustain. Energy Rev. 161, 112384. doi:10.1016/j.rser.2022.112384
Logan, K. G., Nelson, J. D., and Hastings, A. (2020a). Electric and hydrogen buses: shifting from conventionally fuelled cars in the UK, 85. Transportation Research Part D: Transport and Environment. doi:10.1016/j.trd.2020.102350
Logan, K. G., Nelson, J. D., McLellan, B. C., and Hastings, A. (2020b). Electric and hydrogen rail: potential contribution to net zero in the UK, 87. Transportation Research Part D: Transport and Environment. doi:10.1016/j.trd.2020.102523
Lugovoy, O., Gao, S., Gao, J., and Jiang, K. (2021a). Feasibility study of China’s electric power sector transition to zero emissions by 2050. Energy Econ. 96, 105176. doi:10.1016/j.eneco.2021.105176
Lugovoy, O., Jyothiprakash, V., Chatterjee, S., Sharma, S., Mukherjee, A., Das, A., et al. (2021b). Towards a zero-carbon electricity system for India in 2050: ideea model-based scenarios integrating wind and solar complementarity and geospatial endowments. Energies 14 (21), 7063. doi:10.3390/en14217063
Manjong, N. B., Oyewo, A. S., and Breyer, C. (2021). Setting the pace for a sustainable energy transition in central africa: the case of Cameroon. IEEE Access 9, 145435–145458. doi:10.1109/ACCESS.2021.3121000
Martes, L., and Köhl, M. (2022). Improving the contribution of forests to carbon neutrality under different policies—a case study from the Hamburg metropolitan area. Sustain. Switz. 14 (4), 2088. doi:10.3390/su14042088
Martin, A., Agnoletti, M. F., and Brangier, E. (2020). Users in the design of hydrogen energy systems: a systematic review. Int. J. Hydrogen Energy 45 (21), 11889–11900. doi:10.1016/j.ijhydene.2020.02.163
Martínez-Gordón, R., Sánchez-Diéguez, M., Fattahi, A., Morales-España, G., Sijm, J., and Faaij, A. (2022). Modelling a highly decarbonised North Sea energy system in 2050: a multinational approach. Adv. Appl. Energy 5, 100080. doi:10.1016/j.adapen.2021.100080
Matthews, H. D., Zickfeld, K., Dickau, M., MacIsaac, A. J., Mathesius, S., Nzotungicimpaye, C.-M. M., et al. (2022). Temporary nature-based carbon removal can lower peak warming in a well-below 2 °C scenario. Commun. Earth Environ. 3 (1), 65. doi:10.1038/s43247-022-00391-z
Oshiro, K., Masui, T., and Kainuma, M. (2018). Transformation of Japan’s energy system to attain net-zero emission by 2050. Carbon Manag. 9 (5), 493–501. doi:10.1080/17583004.2017.1396842
Ozawa, A., Tsani, T., and Kudoh, Y. (2022). Japan’s pathways to achieve carbon neutrality by 2050 – scenario analysis using an energy modeling methodology. Renew. Sustain. Energy Rev. 169, 112943. doi:10.1016/j.rser.2022.112943
Page, M. J., McKenzie, J. E., Bossuyt, P. M., Boutron, I., Hoffmann, T. C., Mulrow, C. D., et al. (2021). The PRISMA 2020 statement: an updated guideline for reporting systematic reviews. Syst. Rev. 10 (1), 89–11. doi:10.1186/s13643-021-01626-4
Pamucar, D., Deveci, M., Canıtez, F., Paksoy, T., and Lukovac, V. (2021). A novel methodology for prioritizing zero-carbon measures for sustainable transport. Sustain. Prod. Consum. 27, 1093–1112. doi:10.1016/j.spc.2021.02.016
Pilpola, S., Arabzadeh, V., Mikkola, J., and Lund, P. D. (2019). Analyzing national and local pathways to carbon-neutrality from technology, emissions, and resilience PerspectivesCase of Finland. Energies 12 (5), 949. doi:10.3390/en12050949
Pradhan, B. B., Chaichaloempreecha, A., Chunark, P., Rajbhandari, S., Pita, P., and Limmeechokchai, B. (2022). Energy system transformation for attainability of net zero emissions in Thailand. Int. J. Sustain. Energy Plan. Manag. 35, 27–44. doi:10.54337/ijsepm.7116
Price, J., Keppo, I., and Dodds, P. E. (2023). The role of new nuclear power in the UK’s net-zero emissions energy system. ENERGY 262, 125450. doi:10.1016/j.energy.2022.125450
Ren, M., Ma, T., Fang, C., Liu, X. R., Guo, C. Y., Zhang, S. L., et al. (2023). Negative emission technology is key to decarbonizing China’s cement industry, 329. APPLIED ENERGY. doi:10.1016/j.apenergy.2022.120254
Roach, P. E., and Ugursal, V. I. (2021). Development and techno-economic evaluation of pathways to new construction net-zero energy houses in Nova Scotia, Canada. Trans. Can. Soc. Mech. Eng. 45 (2), 221–232. doi:10.1139/tcsme-2019-0247
Rogelj, J., Luderer, G., Pietzcker, R. C., Kriegler, E., Schaeffer, M., Krey, V., et al. (2015). Energy system transformations for limiting end-of-century warming to below 1.5 °C. Nat. Clim. CHANGE, 5(6), 519-+. 527. doi:10.1038/nclimate2572
Sartori, I., Napolitano, A., and Voss, K. (2012). Net zero energy buildings: a consistent definition framework. Energy Build. 48, 220–232. doi:10.1016/j.enbuild.2012.01.032
Scheepers, M., Palacios, S. G., Jegu, E., Nogueira, L. P., Rutten, L., van Stralen, J., et al. (2022). Towards a climate-neutral energy system in The Netherlands. Renew. Sustain. Energy Rev. 158, 112097. doi:10.1016/j.rser.2022.112097
Schreyer, F., Luderer, G., Rodrigues, R., Pietzcker, R. C., Baumstark, L., Sugiyama, M., et al. (2020). Common but differentiated leadership: strategies and challenges for carbon neutrality by 2050 across industrialized economies. Environ. Res. Lett. 15 (11), 114016. doi:10.1088/1748-9326/abb852
Shaffril, H. A. M., Krauss, S. E., and Samsuddin, S. F. (2018). A systematic review on Asian’s farmers’ adaptation practices towards climate change. Sci. Total Environ. 644, 683–695. doi:10.1016/j.scitotenv.2018.06.349
Shimoda, Y., Sugiyama, M., Nishimoto, R., and Momonoki, T. (2021). Evaluating decarbonization scenarios and energy management requirement for the residential sector in Japan through bottom-up simulations of energy end-use demand in 2050. Appl. Energy 303, 117510. doi:10.1016/j.apenergy.2021.117510
Simon, S., Xiao, M. Z., Harpprecht, C., Sasanpour, S., Gardian, H., and Pregger, T. (2022). A pathway for the German energy sector compatible with a 1.5 °C carbon budget. Sustain. Switz. 14 (2), 1025. doi:10.3390/su14021025
Solomon, A. A., Bogdanov, D., and Breyer, C. (2018). Solar driven net zero emission electricity supply with negligible carbon cost: israel as a case study for sun belt countries. Energy 155, 87–104. doi:10.1016/j.energy.2018.05.014
Sorgho, R., Quiñonez, C. A. M., Louis, R., Winkler, V., Dambach, P., Sauerborn, R., et al. (2020). Climate change policies in 16 west african countries A systematic review of adaptation with a focus on agriculture food security and nutrition international journal of environmental research and public health.
Toma, A. R., Olteanu, M. V., Gheorghe, C. M., and Dumitrescu, A. (2019). Promoting H ousehold E nergy C onservation through F eedback on E nergy C onsumption I nformation Project Submitted to.
UKAEA (2023). Spherical tokamak for energy production. Available at: https://step.ukaea.uk/.
Valente, A., Iribarren, D., and Dufour, J. (2020). Prospective carbon footprint comparison of hydrogen options, 728. Science of the Total Environment. doi:10.1016/j.scitotenv.2020.138212
Vats, G., and Mathur, R. (2022). A net-zero emissions energy system in India by 2050: an exploration. J. Clean. Prod. 352, 131417. doi:10.1016/j.jclepro.2022.131417
Voss, K., Musall, E., Sartori, I., and Lollini, R. (2013). Nearly zero, net zero, and plus energy buildings–theory, terminology, tools, and examples. Transition Renew. energy Syst., 875–889. doi:10.1002/9783527673872.ch41
Wang, X., Bamisile, O., Chen, S., Xu, X., Luo, S., Huang, Q., et al. (2022). Decarbonization of China’s electricity systems with hydropower penetration and pumped-hydro storage: comparing the policies with a techno-economic analysis. Renew. Energy 196, 65–83. doi:10.1016/j.renene.2022.06.080
Watari, T., Cao, Z., Hata, S., and Nansai, K. (2022). Efficient use of cement and concrete to reduce reliance on supply-side technologies for net-zero emissions. Nat. Commun. 13 (1), 4158. doi:10.1038/s41467-022-31806-2
Wiest, F., Gamarra Scavone, M. G., Newell, M. T., Otto, I. M., and Ringsmuth, A. K. (2022). Scaling up ecovillagers’ lifestyles can help to decarbonise Europe. Sustain. Switz. 14 (20), 13611. doi:10.3390/su142013611
Wohland, J., Witthaut, D., and Schleussner, C. F. (2018). Negative emission potential of direct air capture powered by renewable excess electricity in Europe. EARTHS FUTURE 6 (10), 1380–1384. doi:10.1029/2018EF000954
Xiao, M., Simon, S., and Pregger, T. (2019). Energy system transitions in the eastern coastal metropolitan regions of China—The role of regional policy plans. Energies 12 (3), 389. doi:10.3390/en12030389
Yang, X., Nielsen, C. P., Song, S., and McElroy, M. B. (2022). Breaking the hard-to-abate bottleneck in China’s path to carbon neutrality with clean hydrogen. Nat. Energy 7 (10), 955–965. doi:10.1038/s41560-022-01114-6
Yumashev, D., Janes-Bassett, V., Redhead, J. W., Rowe, E. C., and Davies, J. (2022). Terrestrial carbon sequestration under future climate, nutrient and land use change and management scenarios: a national-scale UK case study. Environ. Res. Lett. 17 (11), 114054. doi:10.1088/1748-9326/aca037
Zhai, H. B., Gu, B. H., Zhu, K. W., and Huang, C. (2023). Feasibility analysis of achieving net-zero emissions in China?s power sector before 2050 based on ideal available pathways. Environ. IMPACT Assess. Rev. 98, 106948. doi:10.1016/j.eiar.2022.106948
Zhang, S., Yi, B., Guo, F., and Zhu, P. (2022b). Exploring selected pathways to low and zero CO2 emissions in China’s iron and steel industry and their impacts on resources and energy. J. Clean. Prod. 340, 130813. doi:10.1016/j.jclepro.2022.130813
Keywords: climate change, climate mitigation, zero carbon, net-zero, systematic review, transitions, scenarios, pathways
Citation: Shaw A, Mander S, Parkes B and Wood R (2023) Zero carbon transitions: a systematic review of the research landscape and climate mitigation potential. Front. Energy Res. 11:1268270. doi: 10.3389/fenrg.2023.1268270
Received: 27 July 2023; Accepted: 04 September 2023;
Published: 19 September 2023.
Edited by:
Francesco Liberato Cappiello, University of Naples Federico II, ItalyReviewed by:
Luca Cimmino, University of Naples Federico II, ItalyCopyright © 2023 Shaw, Mander, Parkes and Wood. This is an open-access article distributed under the terms of the Creative Commons Attribution License (CC BY). The use, distribution or reproduction in other forums is permitted, provided the original author(s) and the copyright owner(s) are credited and that the original publication in this journal is cited, in accordance with accepted academic practice. No use, distribution or reproduction is permitted which does not comply with these terms.
*Correspondence: Alexander Shaw, YWxleGFuZGVyLnNoYXctMkBtYW5jaGVzdGVyLmFjLnVr
Disclaimer: All claims expressed in this article are solely those of the authors and do not necessarily represent those of their affiliated organizations, or those of the publisher, the editors and the reviewers. Any product that may be evaluated in this article or claim that may be made by its manufacturer is not guaranteed or endorsed by the publisher.
Research integrity at Frontiers
Learn more about the work of our research integrity team to safeguard the quality of each article we publish.