- 1School of Aeronautical and Mechanical Engineering, Changzhou Institute of Technology, Changzhou, China
- 2School of Automotive Engineering, Changzhou Institute of Technology, Changzhou, China
The electrocaloric effect (ECE) depends on the sudden change of the polarization field during ferroelectric phase transition near the Curie temperature. Similarly, giant ECE can be found enormously during the domain structure transition from multidomain to monodomain process in ferroelectrics. To reveal the mechanism with the effects of the electric inclusions, the ECE of PbTiO3 (PTO) ferroelectric solids under electric loads is investigated by phase-field simulation. The giant ECEs of ferroelectric materials containing three kinds of electric inclusions, namely, air, silicone oil, and water, are discussed in detail under applied electric fields. The results suggest that an unusual ultrahigh negative ECE (−9.30 K) and a large EC strength (
1 Introduction
Solid-state refrigeration of ferroelectric materials has shown its low cooling energy density and high efficiency for miniaturization of electronic components. However, the low electrocaloric effect (ECE) and the limitation of the choice of ferroelectric materials hinder the application of the large ECE. With the giant ECE (12K) in the Pb(Zr,Ti)O3 and polyvinylidene fluoride (PVDF) thin films (TFs) being obtained (Mischenko et al., 2006; Neese et al., 2008), the ferroelectric refrigeration has received more attention. After that, tremendous ECEs have been widely available in typical ferroelectrics such as PbTiO3 (PTO) (Qiu and Jiang, 2008), BaTiO3 (BTO) (Huang et al., 2018a), and lead-free K1-xNaxNbO3 (KNN) (Zhao et al., 2020) by doping elements. A large number of experiments have found that the phase transition temperature of ferroelectric materials will sharply reduce to room temperature by element doping, which will also increase the operating temperature range of large ECEs .
With the development of experimental research on the ECE, multilayer composites (Qian et al., 2021; Liu et al., 2022a) and ceramic–polymer ferroelectric nanocomposites (Lich et al., 2020; Liu Y. et al., 2022b) can integrate the performance advantages of ferroelectric materials to strengthen the ECE and EC. Recently, molecular ferroelectric ImClO4 and two-dimensional ferroelectric CuInP2S6 have shown excellent performance in electrocaloric refrigeration. Superior EC strength (
Different from ECEs actuated by ferroelectric–paraelectric phase transitions from the intrinsic factors of ion doping and material lamination, external stress–strain regulation can also significantly affect the change of the domain structure, eventually leading to large ECEs (Wang et al., 2014; Liu and Wang, 2015). The large ECEs generated by multidomain-to-monodomain transitions of PTO ferroelectrics under mismatch strains have been obtained by phase-field simulation (Hou et al., 2018). Most of the results show that in-plane polarization is produced by tensile mismatch strain, while out-of-plane polarization is affected by compressive strain. Different from the mismatch strain, the appropriate stress can also enhance the EC strength and increase the working temperature range of high EC strength (Wu et al., 2015).
Furthermore, the designed shape and structure of ferroelectrics can dramatically affect the ECEs. When the ferroelectric material contains cracks under the action of the electric field, a high electrostatic energy is generated in the cracks and the stress concentration near the tip of the cracks causes domain structure transition, thereby generating a giant ECE (Huang et al., 2021). So far, there are few research works on ferroelectric ECE with defects. In this paper, large ECEs induced by multidomain-to-monodomain transition in ferroelectrics with electrical inclusions are investigated based on the phase-field method. The main contents of the paper are arranged as follows: through the concise introduction, the phase-field model of ferroelectrics containing elliptical electric inclusion energy is given, and the unique electrical boundary conditions of the ferroelectric model containing electric inclusions are set in Section 2. The different giant ECEs formed by the ferroelectric model containing air, silicone oil, and water under the applied electric field are simulated, and the EC strength of the three models is analyzed in Section 3. Finally, the paper is summarized in Section 4.
2 Simulation methodology
Based on the Ginzburg–Landau phase transition theory, the total energy of the system is expressed as a function of time-dependent order parameters (Huang et al., 2018b). In particular, in order to simulate the influence of electrical inclusions on the evolution of the domain structure, the electrical inclusions are simulated as dielectrics filled with air, silicone oil, and water, which have negligible elastic energy. Then, the total electrical enthalpy
where
Based on the basic principle of thermodynamics and Ginzburg–Landau equation, the mechanical equilibrium equation, electrical equilibrium equation, and phase-field dynamic equation can be expressed as follows (Huang et al., 2018a):
where
where
Based on the phenomenological theory and thermodynamic principle, through the Maxwell relationship, when the external electric field is applied, the adiabatic temperature change (
where
Figure 1 shows the overall schematic diagram of the ferroelectric plate with an electric inclusion under the applied electric field. As the phase-field model of this paper, the dimensionless sizes are set as follows: the length of the ferroelectric plate is 120, the width is 90, and the thickness is 1. The minimal thickness is set to degenerate the 3-D problem into the plane stress problem, which greatly simplifies the calculation process. The electric inclusion at the center is a flat ellipse, the long half axis of the electric inclusion is 20, and the short half axis is 1. It should be pointed out that in order to only reflect the influence of electrical inclusions on the domain structure, zero stress or strain loading is applied. The upper and lower electric potential values are set as
Figure 2 shows the mesh division of the model and the mesh densification at the crack tip, which results in the refined mesh size being much smaller than the domain wall size to ensure the correctness of the phase-field model. The material parameters of PTO in this paper are consistent with those in the previous work (Huang et al., 2018b). The dielectric constant of the medium in inclusion as air, oil, or water can be set as
The initial polarization field direction is randomly distributed and the initial value is two orders of magnitude lower than that of the simulated polarization field, which is convenient to simulate the growth and enlargement of the ferroelectric domain structure, and finally form a steady-state evolution process. The dimensionless time step of the computational simulation is taken as
3 Results and discussion
First, the ECE of the electric inclusion model containing air is simulated in this paper. Figure 3 shows the curve of the average polarization field along the y direction with the temperature under the electric loads. The results show that the polarization field increases suddenly at A1-A2 and B1-B2, resulting in negative giant ECEs, which is consistent with the previous simulation results (Hou et al., 2018). The results also show that when the temperature exceeds 500°C, ferroelectric phase-to-paraelectric phase transition occurs in ferroelectric materials containing air inclusions, and the polarization field decreases sharply, resulting in positive giant ECEs.
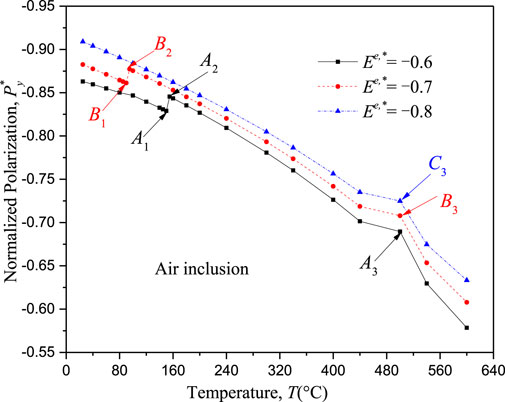
FIGURE 3. Polarization vs. temperature (P-T) curves of PTO with an air inclusion under different electric loads.
In order to reveal the mechanism of large ECE through microstructure evolution, the detailed domain structures of PTO with an air inclusion are given in Figure 4 (a1)—(d1), respectively. Under zero electric loads, the domain structure is in a head-to-tail state at different temperatures, which can greatly reduce the depolarization energy, as shown in Figure 4 (a1) and (c1). Figures 4 (b1) and (d1) exhibit the domain transition from the multidomain to monodomain state along the direction of the electric field (
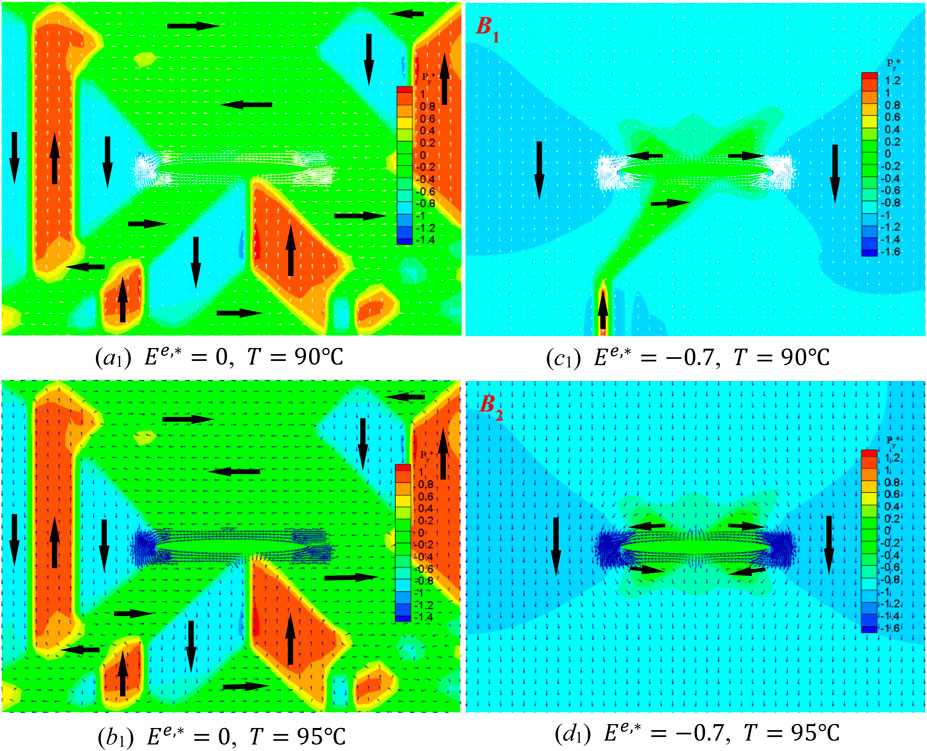
FIGURE 4. Domain structures of PTO under different electric loads near the transition temperature, which correspond to the points
Figure 5 indicates that when the applied electric field changes, the average adiabatic temperature change has the largest negative ECE near the ambient temperature field (
As we know, the air inclusion is apt to dielectric breakdown and produces a discharge phenomenon under high applied electric loads. Therefore, the inclusion is filled with silicone oil or water, which can effectively protect the ferroelectric material and improve the tolerance of the applied electric field strength. Figure 6 shows the temperature dependence of the polarization component P2 of PTO with oil and water inclusions under different electric loads. The results show that when the electric field is applied, the polarization field of the model containing silicone oil inclusion increases abruptly at C1-C2 and the water-inclusion model changes abruptly at D1-D2, all of which produce negative giant ECEs. Interestingly, the temperature for the giant ECE of the water-inclusion model is 50°C, which effectively controls the temperature around the room temperature, which is convenient for experimental and engineering applications.
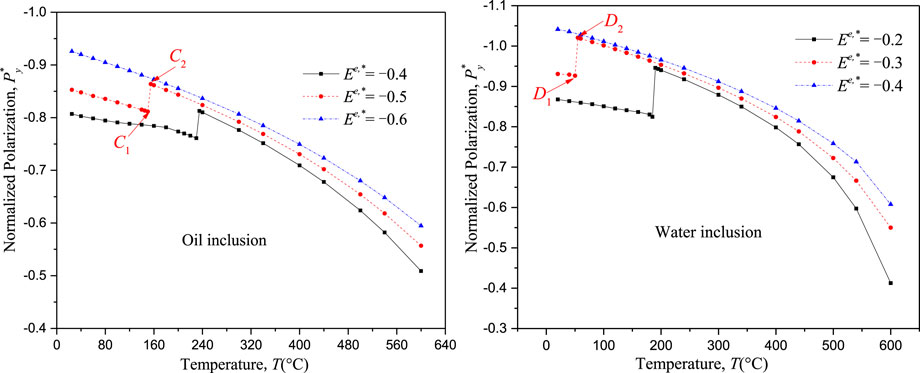
FIGURE 6. Comparison of the P-T curves of PTO with oil and water inclusions under different electric loads.
The detailed domain structures of PTO with oil and water inclusions at C1-C2 and D1-D2 to explain the mechanism of the giant ECE are shown in Figures 7, 8, respectively. The evolution of the microstructure reveals that the multidomain-to-monodomain transition is the result of energy minimization of the whole system under the coupling multifield, including the electric inclusion with high electrostatic energy, which explains the macroscopic behavior of the P-T curve in Figure 6 from the microscopic mechanism.
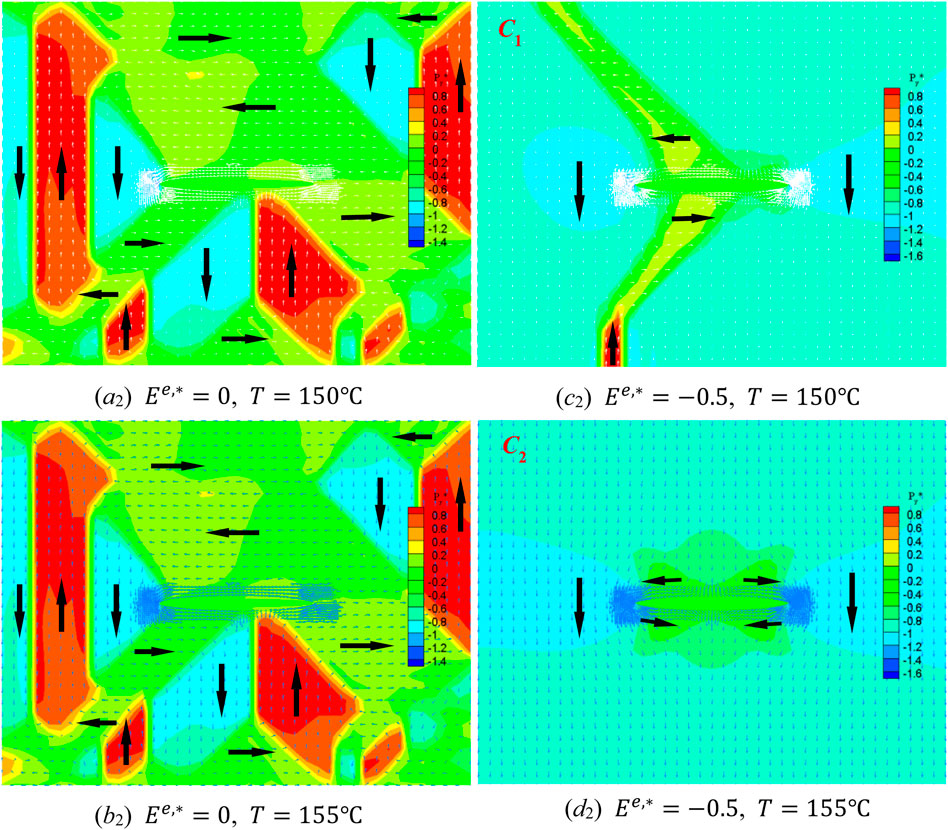
FIGURE 7. Domain structures of PTO under different electric loads near the transition temperature, which correspond to the points
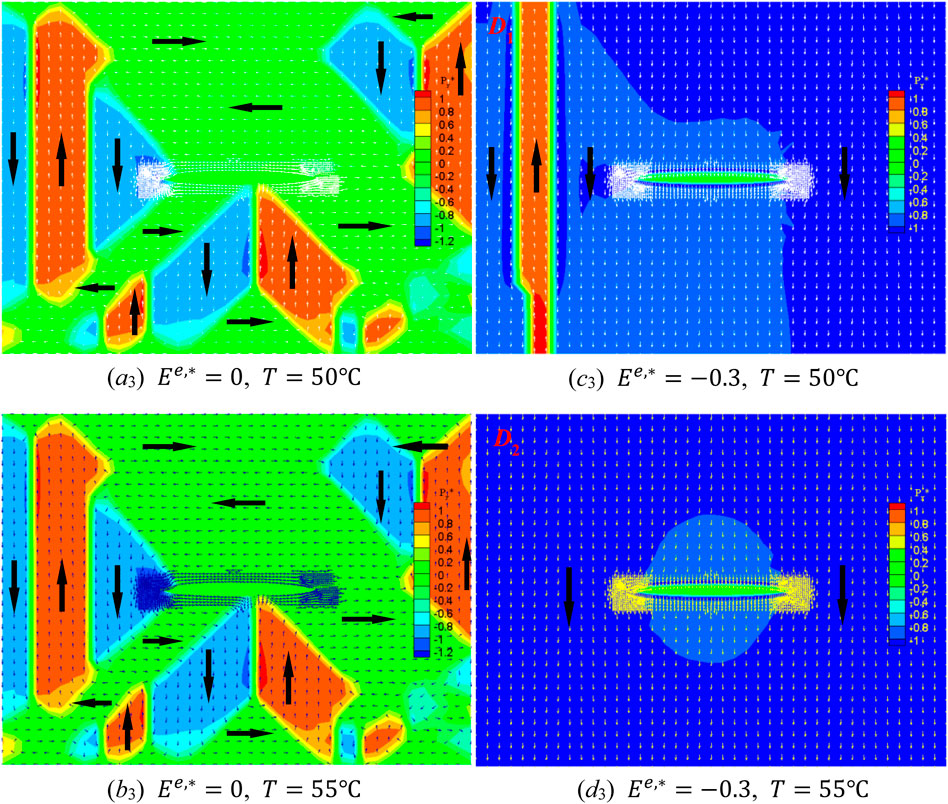
FIGURE 8. Domain structures of PTO under different electric loads near the transition temperature, which correspond to the points
The adiabatic temperature change under different electric loads is shown in Figure 9 for the oil-inclusion and water-inclusion models. As shown in Figure 9, the peak value of negative ATC of the water-inclusion model at 50°C with
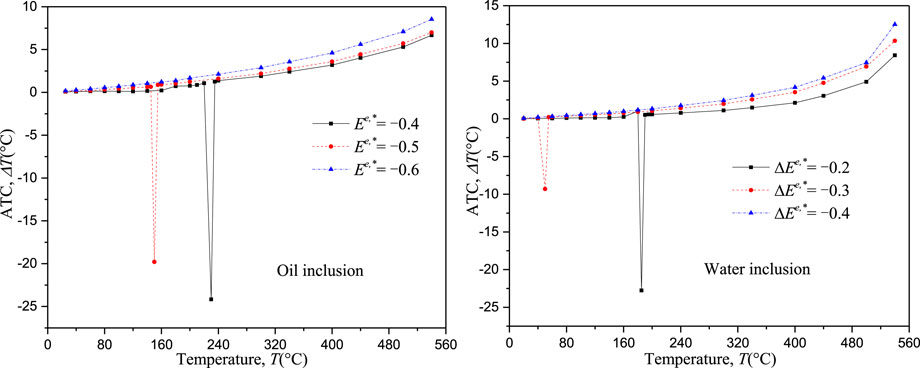
FIGURE 9. Comparison of
4 Concluding remarks
In summary, the ECE of ferroelectric solids with different electric inclusions under electric loads is studied using a phase-field method. Numerical simulations show that the electric inclusion models containing air and silicone oil produce high electrostatic energy under applied electric field, and negative large ECEs are produced, but the domain transition temperature is much higher than room temperature. Because the dielectric constant of water and PTO are on the same order of magnitude, there is no electric field concentration inside the water inclusion, which only affects the distribution of the polarization field of the model as a crack; thus, a ferroelectric solid with a water inclusion under relatively lower applied electric field
Data availability statement
The original contributions presented in the study are included in the article/Supplementary Material. Further inquiries can be directed to the corresponding authors.
Author contributions
CH: formal analysis, methodology, writing–original draft, and writing–review and editing. XW: conceptualization and writing–review and editing. JZ: funding acquisition and writing–review and editing.
Funding
The authors declare financial support was received for the research, authorship, and/or publication of this article. The projects were supported by the National Natural Science Foundation of China (Grant No. 62273061), the Natural Science Foundation of the Jiangsu Higher Education Institutions of China (22KJA580001), and Qing Lan Project of Jiangsu Province of China (Grant No. 31120222003).
Conflict of interest
The authors declare that the research was conducted in the absence of any commercial or financial relationships that could be construed as a potential conflict of interest.
Publisher’s note
All claims expressed in this article are solely those of the authors and do not necessarily represent those of their affiliated organizations, or those of the publisher, the editors, and the reviewers. Any product that may be evaluated in this article, or claim that may be made by its manufacturer, is not guaranteed or endorsed by the publisher.
References
Hou, X., Wu, H., Li, H., Chen, H., and Wang, J. (2018). Giant negative electrocaloric effect induced by domain transition in the strained ferroelectric thin film. J. Phys. Condens Matter. 30 (46), 465401. doi:10.1088/1361-648X/aae602
Huang, C., Yang, H. B., and Gao, C. F. (2018b). Giant electrocaloric effect in a cracked ferroelectrics. J. Appl. Phys. 123 (15), 154102. doi:10.1063/1.5004203
Huang, C., Yin, M. S., Qi, X., and Guo, H. (2021). Torsion of circular shaft with elliptical inclusions or cracks. Trans. Nanjing Univ. Aeronautics Astronautics 38 (1), 96–105. doi:10.16356/j.1005⁃1120.2021.01.009
Huang, Y. H., Wang, J. J., Yang, T. N., Wu, Y. J., Chen, X. M., and Chen, L. Q. (2018a). A thermodynamic potential, energy storage performances, and electrocaloric effects of Ba1-xSrxTiO3 single crystals. Appl. Phys. Lett. 112 (10), 102901. doi:10.1063/1.5020515
Li, W. R., Jafri, H. M., Zhang, C., Zhang, Y. J., Zhang, H. B., Huang, H. B., et al. (2020). The strong electrocaloric effect in molecular ferroelectric ImClO4 with ultrahigh electrocaloric strength. J. Mat. Chem. A 8 (32), 16189–16194. doi:10.1039/d0ta05154c
Lich, L. V., Vu, N. L., Ha, M. T., Bui, T. Q., Le, V. T., Nguyen, T. G., et al. (2020). Enhancement of electrocaloric effect in compositionally graded ferroelectric nanowires. J. Appl. Phys. 127 (21), 214103. doi:10.1063/1.5145040
Liu, M., and Wang, J. (2015). Giant electrocaloric effect in ferroelectric nanotubes near room temperature. Sci. Rep. 5, 7728. doi:10.1038/srep07728
Liu, Y. Q., Liu, J. F., Pan, H., Cheng, X. X., Hong, Z. J., Xu, B., et al. (2022a). Phase-field simulations of tunable polar topologies in lead-free ferroelectric/paraelectric multilayers with ultrahigh energy-storage performance. Adv. Mat. 34, 2108772. doi:10.1002/adma.202108772
Liu, Y., Yang, T. N., Zhang, B., Williams, T., Lin, Y. T., Li, L., et al. (2022b). Structural insight in the interfacial effect in ferroelectric polymer nanocomposites. Adv. Mat. 34 (7), 2109926. doi:10.1002/adma.202109926
Mischenko, A. S., Zhang, Q., Scott, J. F., Whatmore, R. W., and Mathur, N. D. (2006). Giant electrocaloric effect in thin-film PbZr0.95Ti0.05O3. Science 311 (5765), 1270–1271. doi:10.1126/science.1123811
Neese, B., Chu, B. J., Lu, S. G., Wang, Y., Furman, E., and Zhang, Q. M. (2008). Large electrocaloric effect in ferroelectric polymers near room temperature. Science 321 (5890), 821–823. doi:10.1126/science.1159655
Qian, X. S., Han, D. L., Zheng, L. R., Chen, J., Tyagi, M., Li, Q., et al. (2021). High-entropy polymer produces a giant electrocaloric effect at low fields. Nature 600, 664–669. doi:10.1038/s41586-021-04189-5
Qiu, J. H., and Jiang, Q. (2008). Effect of electric field on electrocaloric effect in Pb(Zr1−xTix)O3 solid solution. Phys. Lett. A 372 (48), 7191–7195. doi:10.1016/j.physleta.2008.10.042
Si, M. W., Saha, A. K., Liao, P. Y., Gao, S. J., Neumayer, S. M., Jian, J., et al. (2019). Room-temperature electrocaloric effect in layered ferroelectric CuInP2S6 for solid-state refrigeration. ACS Nano 13 (8), 8760–8765. doi:10.1021/acsnano.9b01491
Wang, J., and Kamlah, M. (2009). Three-dimensional finite element modeling of polarization switching in a ferroelectric single domain with an impermeable notch. Smart Mat. Struct. 18, 104008. doi:10.1088/0964-1726/18/10/104008
Wang, J., Liu, M., Zhang, Y., Shimada, T., Shi, S. Q., and Kitamura, T. (2014). Large electrocaloric effect induced by the multi-domain to mono-domain transition in ferroelectrics. J. Appl. Phys. 115 (16), 164102. doi:10.1063/1.4873112
Wu, H. H., Zhu, J., and Zhang, T. Y. (2015). Pseudo-first-order phase transition for ultrahigh positive/negative electrocaloric effects in perovskite ferroelectrics. Nano Energy 16, 419–427. doi:10.1016/j.nanoen.2015.06.030
Keywords: ferroelectrics, electrocaloric effect, phase-field simulation, multidomain-to-monodomain transition, electrical inclusion
Citation: Huang C, Wang X and Zhao J (2023) Large electrocaloric effects induced by multidomain-to-monodomain transition in ferroelectrics with electrical inclusions. Front. Energy Res. 11:1257567. doi: 10.3389/fenrg.2023.1257567
Received: 12 July 2023; Accepted: 14 August 2023;
Published: 28 August 2023.
Edited by:
Haopeng Song, Nanjing University of Aeronautics and Astronautics, ChinaReviewed by:
Quanquan Yang, Huaiyin Institute of Technology, ChinaYan Shi, Nanjing University of Aeronautics and Astronautics, China
Copyright © 2023 Huang, Wang and Zhao. This is an open-access article distributed under the terms of the Creative Commons Attribution License (CC BY). The use, distribution or reproduction in other forums is permitted, provided the original author(s) and the copyright owner(s) are credited and that the original publication in this journal is cited, in accordance with accepted academic practice. No use, distribution or reproduction is permitted which does not comply with these terms.
*Correspondence: Cheng Huang, aHVhbmdjaGVuZ0BjenUuY24=; Jinbo Zhao, emhhb2piQGN6dS5jbg==