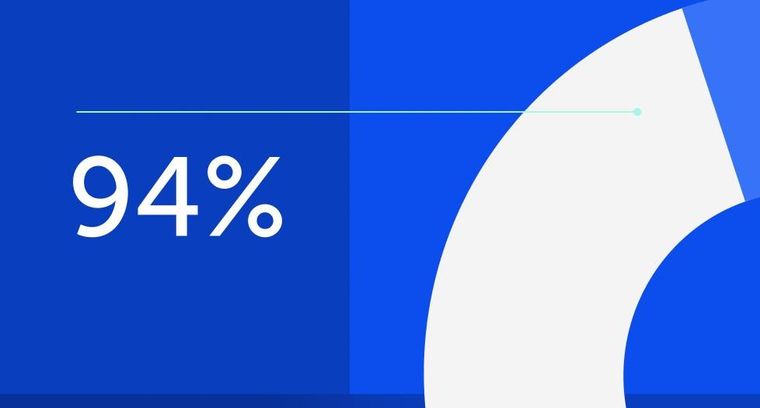
94% of researchers rate our articles as excellent or good
Learn more about the work of our research integrity team to safeguard the quality of each article we publish.
Find out more
EDITORIAL article
Front. Energy Res., 11 July 2023
Sec. Hydrogen Storage and Production
Volume 11 - 2023 | https://doi.org/10.3389/fenrg.2023.1242619
This article is part of the Research TopicMicrobiology of Underground Hydrogen StorageView all 5 articles
Editorial on the Research Topic
Microbiology of underground hydrogen storage
As the energy demand of the growing world population rises, we are challenged with transforming our energy system from fossil fuel towards renewable. Hydrogen (H2), as a universal energy carrier, has been proposed to be a front-runner during this energy transition. It can be used to de-carbonize industries that cannot use electricity directly like steel production, chemical production, aviation or heavy transport, and also be stored to overcome periods with low production of renewable energy (no wind and/or Sun) (Adam et al., 2020; Wang et al., 2022; Razzaq et al., 2023; Xuan et al., 2023). Massive amounts of H2 need to be stored to provide mid- to long-term or seasonal storage for industries or even cities. Underground geological formations offer these required volumes in additions to the advantages of improved safety, low operational costs, and already existing infrastructure. Different types of underground hydrogen storage (UHS) can be used or considered depending on the storage period and demand. For seasonal storage, porous media structures like deep aquifers or depleted oil/gas reservoirs provide the necessary large volumes. Furthermore, artificial salt caverns and hard rock caverns can help to stabilize short-to mid-term energy demands. All these subsurface environments have in common that they are not devoid of life but harbor diverse microbial communities that can thrive under harsh conditions such as, oxygen-free, high temperature, high salinity, and/or high pressure.
The aim of this Research Topic is to draw attention to possible microbially induced challenges in the storage of hydrogen in the subsurface. What influence can microbiological processes have on the storage of hydrogen? What consequences can occur, what products can arise (e.g., toxic or corrosive H2S), how can the risk be reduced, and how can we model, simulate in the laboratory, or predict the potential risks?
The aspect of microbiology is often not considered when planning underground hydrogen storage. However, many different microorganisms (both Bacteria and Archaea) can be present which is highlighted by all of the contributions of this Research Topic (Liu et al.; Schwab et al.; Strobel et al.; Tremosa et al.). Hydrogen is a universal energy carrier also for microbes and can be used as an electron donor by various microbial groups. In addition to a potential loss of the hydrogen (and thus economic loss), biological processes can also cause other potentially hazardous/costly/environmentally important consequences like H2S formation, CH4 formation, biological plugging, corrosion and can cause various geochemical reactions. The three microbial metabolisms of biggest concern are sulfate reduction, methanogenesis and acetogenesis.
Theoretically, the mentioned processes can be present in any storage site, but the complexity of biology does not yet allow a generally valid conclusion. It is rather the case that seemingly identical conditions and similar microbial communities can lead to completely different reactions for reasons not yet understood.
In the study of Liu et al., the hydrogen consumption by a halophilic sulfate-reducing microorganisms was studied in a pressurized microfluidic chip mimicking the porous media structure. The H2 consumption and the potential effects caused by biofilm formation in porous rock, which can hinder hydrogen recovery, are considered. The microbial strain consumed the H2 and this decreased the overall amount and also disconnected H2 bubbles, which will lead to a less efficient recovery. They also observed that the wettability of the pore rock was altered by the microbes, which is an important chemo-physical parameter within reservoir engineering. The wettability changes were probably caused by the attached cells or by biofilm formation. However, clear evidence for bio-plugging was not seen but this result could look very different when using a different strain or a microbial community. The results show that under perfect conditions, sulphate reducers can consume significant amounts of H2. More studies looking at different strains are necessary and whether the observed rates will also be possible in the field.
In contrast to the previous study, Schwab et al. used mixed communities enriched from hypersaline gas storage sites to perform microcosm experiments under H2 atmosphere to test the effect of varying salt concentration and carbon sources. At regularly scheduled intervals, the cell numbers, acetate, lactate and sulfate concentrations, and the gas composition were monitored and after the experiment the composition of the microbial community was assessed by 16S rRNA gene-based amplicon sequencing. They showed a strong impairment of microbial activity with increasing salinity, including reduced sulfate reduction, leading to extremely slow rates. At low to moderate salinity concentrations, the addition of various carbon sources showed increased sulfate reduction. Without carbon sources, homoacetogenic activity at high salinity apparently facilitated sulfate reduction. In conclusion, they highlight that the effects of different acetate concentrations on sulfate-reducing activity in hypersaline systems will be a key issue for further research.
There are some known cases of microbially induced conversion during H2 storage trials, e.g., Ketzin (Germany), Beynes saline aquifer (France), underground Sun storage project by RAG Austria AG (Austria) or in Lobodice town gas storage (Czech Republic). The latter showed the unintentional conversion of about 10%–20% of stored H2 into methane which is typical sign of activity by methanogenic archaea.
In the study of Tremosa et al., based on this case of damage, it was examined whether three already existing models can simulate the loss of H2 accurately. They included values/data of different parameters collected before the damage and evaluate which of the models simulate the observed situation best and what are the weak points of other models. They demonstrated the importance of considering the effects of microbial kinetics on aqueous redox reactions with hydrogen as an electron donor to properly simulate hydrogen reactivity. Testing the various ways to account for methanogenesis, sulfate reduction, and acetogenesis using different reactive models showed that an appropriate level of hydrogen reactivity can only be captured if kinetics controls these reactions. Calibration of kinetic rates and work on transferring redox reaction rates from the aqueous laboratory to the reservoir scale needs to be further developed. Furthermore, observational data from other hydrogen reservoirs are needed to better constrain hydrogen reactivity and its relationship to ambient conditions.
The increasing cases of subsurface hydrogen conversion by methanogens gave rise to a novel concept, the so-called bio-methanation process, where green H2 and captured CO2 are introduced into the subsurface and converted by methanogenic archaea into carbon-neutral CH4. Focusing on this, Strobel et al. carried out laboratory experiments combined the results with modeling. With the focus on a methanogenic archaeon, data on growth kinetics, hydrogen conversion rates, changes in carbon concentration and gas concentration changes in the liquid or gas phase are considered in batch or chemostat experiments. They could culture the microbe and saw repeated H2 conversion over time. They could successfully use their experimental data and built a first model as a basis to be used for predicting bio-methanation in future trials. The authors of the study, also draw attention to the weaknesses of their own study (e.g., changes in gas compositions could not be measured and therefore yield coefficient cannot be calculated, no diffusion coefficient was considered, the reactor was running without porous material). They highlight that more studies with an interdisciplinary background are needed to design meaningful modeling approaches, which will help to understand and predict microbial activity in the subsurface.
All authors listed have made a substantial, direct, and intellectual contribution to the work and approved it for publication.
The authors declare that the research was conducted in the absence of any commercial or financial relationships that could be construed as a potential conflict of interest.
All claims expressed in this article are solely those of the authors and do not necessarily represent those of their affiliated organizations, or those of the publisher, the editors and the reviewers. Any product that may be evaluated in this article, or claim that may be made by its manufacturer, is not guaranteed or endorsed by the publisher.
Adam, N., Schlicht, S., Han, Y., Bechelany, M., Bachmann, J., and Perner, M. (2020). Metagenomics meets electrochemistry: utilizing the huge catalytic potential from the uncultured microbial majority for energy-storage. Front. Bioeng. Biotechnol. 8
Razzaq, I., Amjad, M., Qamar, A., Asim, M., Ishfaq, K., Razzaq, A., et al. (2023). Reduction in energy consumption and CO2 emissions by retrofitting an existing building to a net zero energy building for the implementation of SDGs 7 and 13. Front. Environ. Sci. 10
Xuan, J., Chen, Z., Zheng, J., Zhang, Z., and Shi, Y. (2023). Optimal planning of hybrid electric-hydrogen energy storage systems via multi-objective particle swarm optimization. Front. Environ. Sci. 10
Keywords: biodeterioration and biodegradation, hydrogen storage, modelling, case studies, microbial simulation, anaerobic pathways, geology
Citation: Dopffel N, An-Stepec BA, de Rezende JR, Sousa DZ and Koerdt A (2023) Editorial: Microbiology of underground hydrogen storage. Front. Energy Res. 11:1242619. doi: 10.3389/fenrg.2023.1242619
Received: 19 June 2023; Accepted: 30 June 2023;
Published: 11 July 2023.
Edited and reviewed by:
Uwe Schröder, University of Greifswald, GermanyCopyright © 2023 Dopffel, An-Stepec, de Rezende, Sousa and Koerdt. This is an open-access article distributed under the terms of the Creative Commons Attribution License (CC BY). The use, distribution or reproduction in other forums is permitted, provided the original author(s) and the copyright owner(s) are credited and that the original publication in this journal is cited, in accordance with accepted academic practice. No use, distribution or reproduction is permitted which does not comply with these terms.
*Correspondence: Andrea Koerdt, QW5kcmVhLktvZXJkdEBiYW0uZGU=
Disclaimer: All claims expressed in this article are solely those of the authors and do not necessarily represent those of their affiliated organizations, or those of the publisher, the editors and the reviewers. Any product that may be evaluated in this article or claim that may be made by its manufacturer is not guaranteed or endorsed by the publisher.
Research integrity at Frontiers
Learn more about the work of our research integrity team to safeguard the quality of each article we publish.