- Independent Scientist, Wellington, New Zealand
Hydrogen may substitute traditional hydrocarbon fuels in propeller and jet aircraft. In the case of propeller propulsion, the use of combustion engines is preferable to fuel cells plus electric motors. The conversion efficiency from chemical to mechanical energy at the propeller is larger with fuel cells, but the propulsion system is also larger, in addition to heavier. Fuel cells have a better appeal for novel urban air mobility solutions. Hybridization of gas turbine engines is beneficial for propeller and jet propulsion. The hydrogen aircraft architecture is strongly modified to accept much larger fuel tanks, having hydrogen a larger mass-specific-energy, but a smaller volume-specific-energy, than jet fuels, stored onboard liquid or cold-cryo/compressed. Conformal tanks may reduce the total aircraft volume vs. spherical/cylindrical tanks, the same as the use of novel composite structures to improve strength and reduce the weight of tanks. With conventional designs, the maximum-take-off weight slightly reduces, but the operating-weight-empty largely increases with hydrogen, for an 8%–15% larger energy consumption per pax and nm compared to hydrocarbon fuels. Fuel cell propeller propulsion also suffers from the weight of batteries and fuel cell stack. Non-conventional designs such as Blended-Wing-Body and hybridization may help reduce energy consumption. Renewable-hydrogen-only aircraft necessitate further development of aircraft technology before full deployment by 2035, providing renewable hydrogen will be cheap and abundant at the time, and the airport infrastructure developed. The introduction of hypersonic renewable-hydrogen-only aircraft could also be possible, given the progress in hypersonic technologies and the synergy with subsonic commercial aviation.
1 Introduction
Hydrogen commercial aviation (Brewer, 1976; Pohl, and Malychev, 1997) is an emerging technology that has the potential to transform the airline industry by providing a cleaner and more sustainable alternative to traditional fossil fuels. Renewable hydrogen is a zero-emission fuel produced from renewable energy, such as solar and wind, and a feedstock of water. It can power aircraft without releasing greenhouse gases into the atmosphere. However, the use of hydrogen in commercial aviation is still in the early stages of development, and several challenges need to be addressed before it can become a viable alternative to traditional fuels. These challenges include the high cost of producing and storing hydrogen, the limited infrastructure for hydrogen refueling, and the technical challenges of designing aircraft that can operate on hydrogen fuel. Despite these challenges, several companies and organizations are actively working to develop hydrogen-powered aircraft. For example, Airbus (Airbus, 2023a; b) is currently developing concepts for zero-emission commercial aircraft, powered by hydrogen fuel cells for auxiliaries, and turboprop/turbofan combustion engines for main propulsion.
Overall, while hydrogen commercial aviation is still in the early stages of development, it has the potential to significantly reduce the carbon footprint of the airline industry and help to mitigate the impact of climate change. However, it will require significant investments in research, development, and infrastructure to become a practical and commercially viable option for airlines. By using hydrogen for propulsion in a combustion engine or a fuel cell, the only byproduct is water vapor, making it a clean and environmentally friendly alternative to traditional fossil fuels. Produced from a feedstock of water by using non-dispatchable renewable electricity, hydrogen is a renewable fuel.
Table 1 presents the properties of hydrogen compared to jet fuel.
One of the main benefits of hydrogen propulsion is its high energy density per unit mass, which means that a relatively small mass of hydrogen can provide a lot of energy. Compared to jet fuel, hydrogen requires much less fuel weight for the same energy. Because of the 2.8 times smaller lower heating value of jet fuel compared to hydrogen, the mass of jet fuel stored on board is 2.8 times larger than the mass of hydrogen (Khandelwal et al., 2013). Unfortunately, the weight advantage for the mass of fuel is only hypothetical, as hydrogen is much more difficult to store than jet fuel, requiring a much larger volume, plus cryogenic and/or pressurized tanks, which are much larger and heavier. There are indeed also challenges associated with hydrogen propulsion. One is low energy density per unit volume, which means a small mass of hydrogen still needs a substantial volume even if stored cold/cryo-pressurized. For the same energy stored on board, hydrogen requires 4 times the volume of jet fuel, if loaded liquid (Khandelwal et al., 2013). Hydrogen is also highly flammable, which presents safety concerns. One of the biggest challenges of hydrogen is the complexity of onboard hydrogen loading and storage. Additionally, there is the issue of the cost of production, storage, and distribution, however quickly reducing. The 2021 Energy Earthshot “1-1-1” seeks to reduce the cost of renewable hydrogen to $1 per 1 kg in 1 decade (Department of Energy, 2021a).
Hydrogen has advantages and disadvantages as a transportation fuel. As an advantage, it is zero emission. Hydrogen produces zero greenhouse gas emissions when burned, making it a clean alternative to fossil fuels. It is also abundant. Hydrogen is the most abundant element in the universe, making it a sustainable resource. It is renewable. When produced with renewable energy from the splitting of the water molecule, the production feedstock is the same water produced during oxidation in combustion engines or fuel cells. It is complementary to non-dispatchable renewable energy production. Production of electricity by wind and solar only occurs when there is a resource and not when it is demanded by the grid. Excess electricity may be used to produce hydrogen, which then may be used to produce the missing electricity to the grid, as well as support other uses such as transport. It has a high energy density per unit mass. Hydrogen has a very high energy density per unit mass, meaning that it can be used to store a large amount of energy with a small weight. The lower heating value (LHV) of hydrogen is about 4 times higher than that of jet fuel. The LHV of hydrogen is approximately 120 MJ/kg, while the LHV of jet fuel is about 42–45 MJ/kg. As a disadvantage, it is expensive. Producing, storing, and transporting hydrogen is more expensive than traditional hydrocarbon fuels. It needs infrastructure. A hydrogen fueling infrastructure is still in the early stages of development and is not widely available, which limits the accessibility of hydrogen vehicles. It is dangerous. Hydrogen is a highly flammable gas and can be dangerous if not handled properly, which raises concerns about its use in transportation. It is energy-intensive. The production of hydrogen from the splitting of the water molecule requires a significant amount of energy. It has a low energy density per unit volume. Under ambient conditions for pressure and temperature, hydrogen is a gas with a very low density. The resulting energy density per unit volume is only 10 kJ/L at normal atmospheric pressure and temperature, which is ∼3,130 times less than jet fuels at ∼31,300 kJ/L. When pressurized to 700 bar, it increases to ∼4,500 kJ/L, which is 7 times lower than jet fuels. Liquified at 20 K, it further increases to ∼8,500 kJ/L, which is 3.7 times lower than jet fuels. The main issue with hydrogen transport is certainly the availability of renewable hydrogen at the right environmental and economic cost. The aspect covered in this work is the storage on board in cold/cryo pressurized tanks and the changes to the aircraft to permit this storage in heavier and larger tanks also including enhanced safety features, which is the most relevant aspect from the vehicle perspective. While the combustion of hydrogen in both gas turbines and internal combustion engines certainly needs some refinement of these combustion systems, this issue is much less demanding than the onboard storage issue.
Central to the uptake of hydrogen in aviation is the ability to reach the U.S. Department of Energy’s (DOE’s) 2021 Energy Earthshot “1-1-1” seeking to reduce the cost of renewable hydrogen to $1 per 1 kg in 1 decade (Department of Energy, 2021a). This program aims to accelerate breakthroughs of more abundant, affordable, and reliable clean energy within the decade. Currently, hydrogen from renewable energy costs about $5 per kilogram. The Hydrogen Shot’s 80% reduction in cost may unlock new markets for hydrogen, including aviation.
Hydrogen is currently produced through several methods, including steam methane reforming (SMR), electrolysis of water, coal gasification, and biomass gasification. However, the most common method for large-scale hydrogen production is SMR (Boretti, and Banik, 2021). According to the 2019 International Energy Agency’s report “The Future of Hydrogen” (International Energy Agency, 2019), global hydrogen production reached 115 million tonnes per year. SMR was responsible for about 76% of that production, while electrolysis represented only about 1%.
There is a growing interest in using electrolysis to produce green hydrogen by using renewable energy sources such as wind and solar. Hydrogen as a transportation fuel makes sense only if renewable. Hydrogen is an integral part of replacing an energy system not sustainable based on fossil fuels with a renewable energy system. The production of green hydrogen is still relatively small, but it is expected to increase significantly in the coming years as the demand for sustainable fuels grows. The International Energy Agency (International Energy Agency, 2019) projected that by 2030, global green hydrogen production could reach 530 million tonnes per year, representing a significant increase compared to current levels.
The aviation industry is one of the biggest consumers of fossil fuels, and as such, it is responsible for a significant amount of greenhouse gas emissions. To reduce its carbon footprint, the aviation industry is looking to green hydrogen, produced through the electrolysis of water using renewable energy sources such as wind and solar. Several countries and organizations have announced policies related to the use of green hydrogen in the aviation industry:
European Union: In July 2021, the European Commission proposed the “Fit for 55”package (Council of the European Union, 2021). The program includes a goal to reduce aviation emissions by 90% by 2050. The package also proposes that all flights within the EU should be powered by sustainable aviation fuels, such as green hydrogen, by 2035.
United States: The Biden administration has announced a goal to reduce greenhouse gas emissions by 50%–52% below 2005 levels by 2030 (The White House, 2021). The Federal Aviation Administration (FAA) has also launched a program called “Sustainable Aviation Fuel Grand Challenge,” which aims to increase the production and use of sustainable aviation fuels, including green hydrogen (Department of Energy, 2021b).
Australia: In September 2020, the Australian government announced a $1.9 billion investment in new and emerging technologies, including hydrogen (Australian Hydrogen Council, 2020). The government has also announced the development of a national hydrogen strategy, which includes the use of hydrogen in the aviation industry.
For what concerns manufacturers, as previously mentioned, Airbus has announced plans to develop a zero-emission commercial aircraft by 2035, which would be powered by green hydrogen (Airbus, 2023a). Airbus has also partnered with Air France-KLM to explore the use of hydrogen in aviation (Air France-KLM, 2021).
These are just a few examples of the policies and initiatives related to green hydrogen in the aviation industry. As the demand for sustainable aviation fuels continues to grow, more countries and organizations will likely announce similar policies in the future.
Boretti (2021) recently discussed the perspective of hydrogen fuel for current combustion engine aviation, including business jets, small regional aircraft, regional propeller aircraft, regional jet aircraft, and medium and long-range aircraft. Boretti (2022) then discussed electric propulsion with fuel cells, mostly focusing on urban air mobility, which is a novel mobility mode where uptake of hydrogen fuel cells in completely novel aircraft architectures may be easier than in propeller small regional aircraft and regional propeller aircraft. Finally, hypersonic aircraft, which are another novel mobility mode being considered, have hydrogen as the preferred fuel. The introduction of hypersonic renewable-hydrogen-only aircraft could be possible by 2035, i.e., about the same time as renewable-hydrogen conventional aviation, given the progress in hypersonic technologies and the synergy with subsonic commercial aviation (Boretti, 2023). Worth to mention, the use of renewable-hydrogen-derived ammonia could be a strong competitor for pure hydrogen, being ammonia much easier to store safely and efficiently on board, despite at the price of further complications of the combustion system and production of nitrous oxides as pollutants (Boretti, and Castelletto, 2022).
This work aims to discuss the technical challenges being addressed in designing conventional subsonic aircraft that can operate on pure hydrogen fuel.
2 Propeller propulsion systems
The equation of motion for a propeller aircraft is given by:
where T is the thrust generated by the propeller, D is the drag force, W is the weight of the aircraft, θ is the angle of climb or descent, m is the mass of the aircraft, and a is the acceleration of the aircraft. If g is the gravity acceleration, then W = mg. The thrust generated by the propeller is then expressed as:
where ηp is the propeller efficiency, ηe is the engine efficiency, ηT is the total propulsion efficiency, Pf is the fuel flow power, and v is the airspeed of the aircraft. The drag force is expressed as:
where ρ is the air density, CD is the drag coefficient, and A is a reference area such as the cross-sectional area of the aircraft. Substituting the expressions for T, D, and W, we get:
During the cruise, the terms mg·sin(θ) and ma both disappear.
Modern aeronautic propellers are designed for propulsive efficiencies around ηp = 0.8–0.9. Modern aircraft engines are also designed to be efficient, with combustion engine efficiencies of up to ηe = 0.4–0.5 for some high-performance engines.
The equation of motion for a propeller aircraft relates the thrust generated by the propeller to the drag force generated by the wings, the weight of the aircraft, and the resulting acceleration of the aircraft. It can be used to predict their speed, altitude, and range under different operating conditions.
In a propeller aircraft, the lift force is generated by the wings, and the drag force is generated by both the wings and the propeller, plus the fuselage. The lift and drag forces are related through the lift-to-drag ratio (L/D), which is a measure of the efficiency of the aircraft in converting forward motion into lift. The lift force generated by the wings can be expressed as:
where CL is the lift coefficient.
The lift-to-drag ratio is an important factor in determining the performance of the aircraft. A higher lift-to-drag ratio means that the aircraft is more efficient in generating lift and requires less power to maintain a given airspeed, resulting in better range and fuel efficiency. Conversely, a lower lift-to-drag ratio means that the aircraft requires more power to maintain a given airspeed, resulting in a shorter range and higher fuel consumption.
Comparing hydrogen with jet fuel aircraft, a larger or heavier aircraft translates into a larger power demand through the effect an increased cross-sectional area and a larger lift have on the product CDA and m.
The aircraft propeller propulsion system consists of a combustion engine used to generate thrust via the propeller. The engine converts the fuel energy into mechanical energy. The aircraft propeller propulsion system may also be electric. A fuel cell converts the fuel energy into electricity, and electricity is then converted into mechanical energy by an electric motor.
In the case of fuel cells, slightly better efficiencies of fuel chemical to mechanical at the propeller are possible, as fuel cells may have an efficiency of up to ηfc = 0.65 (Lohse-Busch, Stutenberg, Duoba, and Iliev, 2018) and the electric motor ηem = 0.95, in the best operating points, with their product at about ηe = ηfc·ηem = 0.62. This gives a total propulsion efficiency ηT of 0.50–0.56.
Electric motors have an outstanding power density, for both unit mass and volume. Another advantage of electric motors is the availability of high torque from zero speed, and generally higher efficiencies at every load and speed, which are however relevant to transient rather than cruise operation. While electric motors are very compact, fuel cells by themselves are already much larger and heavier than an internal combustion engine. This translates into a larger and heavier propulsion system because of the fuel cells. In electric propulsion, it is convenient to couple to a fuel cell stack a battery, to buffer the operation of the fuel cell which can be made much smaller and operate more efficiently over a restricted range of loads.
One advantage of the fuel cell propulsion system is that it can be coupled to battery energy storage. This is helpful especially during transients, such as take-off, as the electric motor may draw electricity at the same time from the battery and the fuel cell, thus delivering instantaneously more power than the power of the fuel cell. The battery, which is recharged from the power grid, may also be recharged in-flight, if needed, by demanding from the fuel cell more power than the power to the electric motor, storing the difference in the battery. The fuel cell propulsion system is a better fit for urban air mobility but could be beneficial also for regional propeller aircraft. Fuel cells operate at much higher efficiencies than combustion engines, even if converting the fuel’s chemical energy into electrical energy rather than mechanical energy. The technology of fuel cells is being improved, for higher efficiencies and reduced weight and volume of a stack (Fan et al., 2021). Similarly being improved is battery technology (Li et al., 2021). This may eventually result in fuel cell electric propulsion systems becoming preferable to combustion engine systems to drive propellers, which is a compromise between energy conversion efficiency, and weight and volume energy demand by the aircraft.
3 Jet propulsion systems
The turbojet engine is a type of air-breathing jet engine, which generates thrust by accelerating a stream of air through a nozzle using the energy from burning fuel. The equation of motion for a turbojet aircraft can be derived using the same principles as for a propeller aircraft but with some modifications to account for the different types of engines. In a turbojet aircraft, the thrust is written as:
where ṁ is the mass flow rate of air through the engine, vj is the exhaust jet velocity, v0 is the free-stream air velocity, pj is the pressure in the exhaust jet, p0 is the free-stream air pressure, and S is the area of the engine nozzle. If we take T from (2), where ηp is now the propulsive efficiency of the jet engine, and ηe the thermal efficiency of the gas turbine engine, then also for jet propulsion the propulsion equation is given by (4). This is the equation of motion for a turbojet aircraft to be used to predict its speed, altitude, and range under different operating conditions. The comparison of jet fuel and hydrogen aircraft is made through their impact on m, CD, and A.
As shown by (Epstein, 2014; National Academies of Sciences, Engineering, and Medicine, 2016), engine efficiency ηe ranges from mid 0.4 to high 0.5, while propulsive efficiency ηp ranges from 0.5 to 0.7, for overall efficiency ηT from mid 0.2 to 0.4. Turbojet has the lowest efficiencies, then a low by-pass ratio, and finally a high by-pass ratio.
In addition to the lift and drag forces, a jet aircraft also experiences a thrust force generated by its engines. The thrust force is generally much greater than the drag force, which allows the aircraft to maintain a high airspeed and altitude. The thrust-to-weight ratio (T/W) is another important factor in determining the performance of the aircraft, as it relates the thrust generated by the engines to the weight of the aircraft. A higher thrust-to-weight ratio means that the aircraft is more capable of accelerating and climbing, while a lower thrust-to-weight ratio means that the aircraft may struggle to maintain altitude or climb at a sufficient rate.
4 Hydrogen aircraft design
While relatively accurate novel designs have been proposed for hypersonic and urban air mobility applications, which are complete novelties, not too much has been done so far in the direction of designing more conventional aircraft to work on hydrogen. Over this century, the most important manufacturers have proposed mostly preliminary studies, which are often artist impressions more than detailed engineering studies (Boretti, 2021).
One of the first hydrogen aircraft concepts was proposed by (Brewer, and Morris, 1976). Aircrafts capable of carrying 130 pax over 1,500 nm, 200 pax over 3,000 nm, and 400 pax over 5,000 nm radius missions were designed. The first hydrogen aircraft built and operated was the alternative fuel test plane Tu-155 of 1988. First, the aircraft was operated with liquid hydrogen and then modified to work on liquid natural gas (LNG). Only one of the three jet engines was fueled with hydrogen.
Westenberger (2003) reporting on the Cryoplane project, proposed preliminary ideas about conventional and innovative liquid H2 aircraft configurations, from business jets to regional, from medium to long-range applications, all subsonic, propeller, or jet. While practical configurations were available for all categories of airliners, there was no standard configuration for all categories of airliners. The Max Take Off Weight (MTOW) was smaller, especially for long-range aircraft vs. the jet fuel version (15% smaller). In other cases, there were much smaller or even small penalties in MTOW. The Operating Weight Empty (OWE) generally increased by about 20%–25% vs. the jet fuel versions. Particularly negative was the Very Large Long Range Aircraft, which had an unrealistic 48% large OWE. The specific energy consumption generally increased by 8%–15%, because of the more wetted area, and the higher mean flight weight. The much larger specific energy consumption of the Very Large Long Range Aircraft was unrealistic, the same as those of the business jet, which were sub-optimal. The strongly oscillating values of dMTOW, dOWE, or dE per pax and nm were indeed an indication of the suboptimal definition of the hydrogen aircraft in the exercise, without too much consistency in between results. Opposite to what is suggested in Table 2, large long-range aircraft, are expected to permit much closer to jet fuel energy per pax and nm.
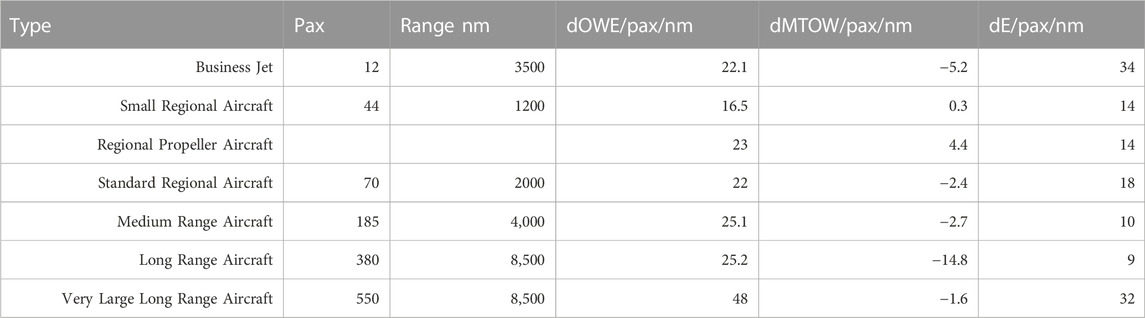
TABLE 2. Pax, range, and difference compared to traditional jet fuel of Max Take Off Weight (MTOW), Operating Weight Empty (OWE), and energy (E) per pax and nm of different hydrogen aircraft concepts from Westenberger (2003).
Westenberger (2003) concluded that the advantages of alternative unconventional configurations such as twin boom or Blended-Wing-Body were not obvious. However, especially the Blended-Wing-Body design may help to better accommodate the hydrogen fuel tanks in addition to reducing the wetted perimeter, and providing advantages in large long-range aircraft.
Different ZeroE concept aircraft have been proposed more recently by Airbus (Airbus, 2023b). They are a 100 pax 1,000 nm traditional turboprop aircraft, fitted with two hybrid turboprop engines, and a blended wing body, or a traditional turbofan aircraft, 200 pax 2,000 nm, fitted with two hybrid turbofan engines. Modified gas turbine engines to work on hydrogen power the aircraft. Liquid hydrogen is said to be used as fuel for combustion with oxygen. In addition, hydrogen fuel cells create electrical power that complements the gas turbine, resulting in a highly efficient hybrid-electric propulsion system. Blended Wing Body aircrafts offer the best opportunity to locate the large and heavy fuel tanks needed to store hydrogen.
All three designs have issues (Scholz, 2020). The turbofan and the turboprop have their LH2 tank in the back resulting in an unbalanced aircraft during flight, with the center of gravity moving as the fuel is consumed. The Blended-Wing-Body design without a vertical tail(s) is not advisable. The Blended-Wing-Body design may be interesting only for very large aircraft. By increasing the wingspan n times, the surface area (and hence the wing lift) will increase by n2 times, and the volume (mass of the entire aircraft) will increase n3 times. With an efficient aerodynamic layout, a flying wing is longitudinally statically unstable.
As commented by (Scholz, 2020), one major issue in the Cryoplane project was the balancing of the center of gravity of the aircraft due to the large and heavy fuel tanks for the liquid hydrogen. One assumption was to use liquid H2 in cylindrical or spherical tanks.
The use of cold/cryo pressurized storage (Ahluwalia, Hua, Peng, Lasher, McKenney, Sinha, and Gardiner, 2010), conformal tanks with graphene composite structure (Ioaircraft, 2021), and non-conventional designs, especially Blended-Wing-Body, could be the avenue of more efficient aircraft’s designs. According to (Liebeck, 2004), a Blended-Wing-Body long-range aircraft for 450 to 800 pax could achieve energy savings per pax and nm above 20%.
While not too much has been done in prototyping and testing of combustion engines propeller or jet propulsion aircraft, regarding fuel cells electric propeller propulsion, more flying demonstrators have been built and tested. The first demonstrator hydrogen fuel cell electric propeller aircraft was built by Boeing in 2008 (Boeing, 2008), a modified two-seat Dimona motor-glider. A small step forward is the latest Zeroavia hydrogen fuel cell electric demonstrator aircraft of 2020 (Zeroavia, 2020). ZeroAvia retrofitted a small Piper M-350 to work with electric propulsion powered by hydrogen fuel cells. Others such as Cummins (Cummins, 2021) have proposed small hydrogen fuel cell electric propeller demonstration aircraft. In addition, Airbus is developing a fuel cell electric propeller engine (Airbus, 2022) that could be used in a larger 100-pax 1,000 nm commercial aircraft.
It must be mentioned as hybridization may come in many different ways. For example, the NASA NX-3 Blended-Wing-Body propeller aircraft (Kim et al., 2013; Liou et al., 2016) uses gas turbines to drive an electric generator producing the electricity then used in the electric motors driving the propellers.
Thus, hydrogen propulsion is less efficient than propulsion with jet fuels, primarily for the increased mass and volume of the aircraft for the same pax and nm, with better design of the aircrafts likely to deliver much closer to today’s jet fuels aircraft energy economy by 2035. The three areas of development are primarily the aircraft concept, and then hybridization, and further improvement, of gas turbine engines for both propeller and jet propulsion applications, plus fuel cell electric propeller propulsion in the case of small regional aircraft.
5 Discussion
Renewable hydrogen aviation has the potential to be a cleaner and more sustainable alternative to traditional fossil aviation fuel. Hydrogen is a clean-burning fuel that produces only water and heat as byproducts, making it a very attractive option for reducing carbon emissions and improving air quality. Renewable hydrogen obtained from the splitting of the water molecule by using non-dispatchable renewables such as wind and solar is also fully renewable. Hydrogen produced from renewable sources is a more sustainable and environmentally-friendly fuel option than current hydrocarbon fossil fuels. However, there are also some challenges associated with the adoption of hydrogen aviation, starting from the high cost of producing, transporting, and storing hydrogen, as well as the need for significant infrastructure upgrades to support the widespread use of hydrogen in the aviation industry. Specific to aircraft design, hydrogen poses challenges as it requires larger and heavier fuel tanks to store the energy needed for a given mission, and this largely impacts the aircraft design. Less challenging is then the further specific development of the combustion systems to deal with hydrogen rather than traditional jet fuels. An emerging technology for propeller rather than jet propulsion aircraft is the use of fuel cells, batteries, and electric motors, which provides higher energy conversion efficiencies, albeit at the cost of the larger and heavier fuel cell stacks compared to combustion engines. Despite these challenges, many companies and governments are investing in research and development to explore the potential of hydrogen aviation and to overcome these obstacles in the future.
Hydrogen may be used to propel aircraft, either conventional business, regional, and medium/long range aircraft as in use today, and urban air mobility or hypersonic aircraft that are novel modes of air transport. The first and foremost issue is the ability to produce cheap and abundant renewable hydrogen, which may be possible by 2030 if the Hydrogen Shot “1-1-1” target (Department of Energy, 2021a) is met, and to make this hydrogen available at the airports for fueling the aircraft. Given this is achieved, then the design of aircraft also needs changes. The use of hydrogen, which is more difficult to be stored than jet fuel, translates into similar Max Take Off Weight, but a larger Operating Weight Empty, and ultimately an 8%–15% larger energy per pax and nm, by converting existing designs to use the hydrogen stored liquid in cylindrical or spherical tanks. The energy penalty could be reduced by better designing the novel aircraft, adopting cold/cryo pressurized storage, lighter and stronger composite fuel tanks, conformal to the available space, introducing non-conventional designs for the aircraft such as Blended-Wing-Body, and further refining the combustion engine technologies, with electrically assisted gas turbines, and more specific designs for hydrogen of the combustion system. Given the progress in fuel cell technologies and batteries, fuel cell electric propeller propulsion could be competitive for regional aircraft. Providing renewable hydrogen will be cheap and abundant by 2030, and the airport infrastructure will be developed, traditional commercial aviation could be converted to hydrogen starting in 2035. At that time, the introduction of hypersonic renewable-hydrogen-only aircraft could also be possible, given the progress in hypersonic technologies and the synergy with subsonic commercial aviation. Similar could be the case of personal air mobility, which is another novel mode of air transport being considered, where fuel cell electric propeller propulsion is promising.
Data availability statement
The original contributions presented in the study are included in the article/Supplementary Material, further inquiries can be directed to the corresponding author.
Author contributions
The author reviewed the literature and wrote the manuscript. The author confirms being the sole contributor of this work and has approved it for publication. All authors contributed to the article and approved the submitted version.
Conflict of interest
The author declares that the research was conducted in the absence of any commercial or financial relationships that could be construed as a potential conflict of interest.
Publisher’s note
All claims expressed in this article are solely those of the authors and do not necessarily represent those of their affiliated organizations, or those of the publisher, the editors and the reviewers. Any product that may be evaluated in this article, or claim that may be made by its manufacturer, is not guaranteed or endorsed by the publisher.
References
Ahluwalia, R. K., Hua, T. Q., Peng, J. K., Lasher, S., McKenney, K., Sinha, J., et al. (2010). Technical assessment of cryo-compressed hydrogen storage tank systems for automotive applications. Int. J. hydrogen energy 35 (9), 4171–4184. doi:10.1016/j.ijhydene.2010.02.074
Air France-KLM (2021). Air France-KLM, Total, Groupe ADP and Airbus have joined forces to carry out the first long-haul flight powered by Sustainable Aviation Fuel (SAF) (1) produced in France. Available at: www.airfranceklm.com/en/newsroom/air-france-klm-total-groupe-adp-and-airbus-join-forces-decarbonize-air-transportation-and (Accessed May 18, 2021).
Airbus (2022). Airbus reveals hydrogen-powered zero-emission engine. Available at: www.airbus.com/en/newsroom/press-releases/2022-11-airbus-reveals-hydrogen-powered-zero-emission-engine (Accessed November 30, 2022).
Airbus (2023a). Hydrogen. An important pathway to our decarbonisation ambition. Available at: www.airbus.com/en/innovation/zero-emission-journey/hydrogen (Accessed September 28, 2022).
Airbus (2023b). Zeroe: Towards the world’s first hydrogen-powered commercial aircraft. Available at: www.airbus.com/en/innovation/zero-emission-journey/hydrogen/zeroe (Accessed September 8, 2022).
Australian Hydrogen Council (2020). Australian Hydrogen Council welcomes Federal Government’s $1.9b investment in new energy technologies. Available at: https://h2council.com.au/wp-content/uploads/2022/10/201917-AHC-welcomes-Federal-Govt-1.9b-investment-in-new-energy-technologies.pdf (Accessed September 17, 2020).
Boeing (2008). Boeing successfully flies fuel cell-powered airplane. Available at:www.boeing.com/aboutus/environment/environmental_report/_inc/flash-2-1-2.html (Accessed April 3, 2008).
Boretti, A. (2022). A net-zero future needs hydrogen not less than batteries, especially for urban air mobility. Int. J. Hydrogen Energy 47 (69), 29956–29958. doi:10.1016/j.ijhydene.2022.06.279
Boretti, A., and Banik, B. K. (2021). Advances in hydrogen production from natural gas reforming. Adv. Energy Sustain. Res. 2 (11), 2100097. doi:10.1002/aesr.202100097
Boretti, A., and Castelletto, S. (2022). NH3 prospects in combustion engines and fuel cells for commercial aviation by 2030. ACS Energy Lett. 7 (8), 2557–2564. doi:10.1021/acsenergylett.2c00994
Boretti, A. (2023). How close is a hydrogen hypersonic commercial aircraft? Int. J. Hydrogen Energy 48 (28), 10684–10691. doi:10.1016/j.ijhydene.2022.11.303
Boretti, A. (2021). Perspectives of hydrogen aviation. Adv. Aircr. Spacecr. Sci. Int. J. 8 (3), 199–211. doi:10.12989/aas.2021.8.3.199
Brewer, G. D. (1976). Aviation usage of liquid hydrogen fuel—Prospects and problems. Int. J. Hydrogen Energy 1 (1), 65–88. doi:10.1016/0360-3199(76)90011-2
Brewer, G. D., and Morris, R. E. (1976). Study of LH2 fueled subsonic passenger transport aircraft. Lockheed, NASA CR-144935. Available at: https://ntrs.nasa.gov/citations/19760012056 (Accessed January 1, 1976).
Council of the European Union (2021). Fit for 55. Available at:https://www.consilium.europa.eu/en/policies/green-deal/fit-for-55-the-eu-plan-for-a-green-transition/(Accessed July 17, 2021).
Cummins (2021). Cummins Hydrogen power takes flight. Available at: www.cummins.com/news/2021/04/15/cummins-hydrogen-power-takes-flight (Accessed April 15, 2021).
Department of Energy (2021a). Hydrogen shot. Available at: https://www.energy.gov/eere/fuelcells/hydrogen-shot (Accessed September 8, 2022).
Department of Energy (2021b). Sustainable aviation fuel Grand challenge. Available at: https://www.energy.gov/eere/bioenergy/sustainable-aviation-fuel-grand-challenge (Accessed September 8, 2022).
Epstein, A. (2014). Aeropropulsion for commercial aviation in the twenty-first century and research directions needed. AIAA J. 52 (5), 901–911. doi:10.2514/1.J052713
Fan, L., Tu, Z., and Chan, S. H. (2021). Recent development of hydrogen and fuel cell technologies: A review. Energy Rep. 7, 8421–8446. doi:10.1016/j.egyr.2021.08.003
International Energy Agency (IEA) (2019). The future of hydrogen. Available at: https://www.iea.org/reports/the-future-of-hydrogen (Accessed September 8, 2022).
Ioaircraft (2021). Conforming tanks. Available at: https://www.ioaircraft.com/technologies/conforming-tanks.php (Accessed August 17, 2021).
Khandelwal, B., Karakurt, A., Sekaran, P. R., Sethi, V., and Singh, R. (2013). Hydrogen powered aircraft: The future of air transport. Prog. Aerosp. Sci. 60, 45–59. doi:10.1016/j.paerosci.2012.12.002
Kim, H. D., Felder, J. L., Tong, M., and Armstrong, M. (2013). Revolutionary aeropropulsion concept for sustainable aviation: Turboelectric distributed propulsion. Available at: https://ntrs.nasa.gov/api/citations/20140002510/downloads/20140002510.pdf (Accessed September 8, 2022).
Li, C., Wang, Z. Y., He, Z. J., Li, Y. J., Mao, J., Dai, K. H., et al. (2021). An advance review of solid-state battery: Challenges, progress and prospects. Sustain. Mater. Technol. 29, e00297. doi:10.1016/j.susmat.2021.e00297
Liebeck, R. H. (2004). Design of the blended wing body subsonic transport. J. Aircr. 41 (1), 10–25. doi:10.2514/1.9084
Liou, M. S., Kim, H., and Liou, M. F. (2016). Challenges and progress in aerodynamic design of hybrid wingbody aircraft with embedded engines. Available at: https://ntrs.nasa.gov/api/citations/20160007898/downloads/20160007898.pdf (Accessed June 1, 2016).
Lohse-Busch, H., Stutenberg, K., Duoba, M., and Iliev, S. (2018). Technology assessment of a fuel cell vehicle: 2017 toyota mirai (No. ANL/ESD-18/12). Argonne, IL: Argonne National Lab ANL.
National Academies of Sciences, Engineering, and Medicine (2016). “Commercial aircraft propulsion and energy systems research: Reducing global carbon emissions,” in Chapter 3 aircraft gas turbine engines (NAS, Washington DC, USA: The National Academies Press).
Pohl, H. W., and Malychev, V. V. (1997). Hydrogen in future civil aviation. Int. J. hydrogen energy 22 (10-11), 1061–1069. doi:10.1016/S0360-3199(95)00140-9
Scholz, D. (2020). Design of hydrogen passenger aircraft: How much" zero-emission" is possible? Reposit. Available at: https://www.haw-hamburg.de/bitstream/20.500.12738/10024/1/text_2020_11_19_HydrogenAircraft.pdf (Accessed September 8, 2022).
The White House (2021). Fact sheet: President biden sets 2030 greenhouse gas pollution reduction target aimed at creating good-paying union jobs and securing U.S. Leadership on clean energy technologies. Available at: https://www.whitehouse.gov/briefing-room/statements-releases/2021/04/22/fact-sheet-president-biden-sets-2030-greenhouse-gas-pollution-reduction-target-aimed-at-creating-good-paying-union-jobs-and-securing-u-s-leadership-on-clean-energy-technologies/(Accessed April 22, 2021).
Westenberger, A. (2003). Cryoplane – hydrogen aircraft. H2 expo at hamburg. Available at: https://www.h2hh.de/downloads/Westenberger.pdf (Accessed September 8, 2022).
Zero Avia (2020). ZeroAvia completes world first hydrogen-electric passenger plane flight. Available at: https://www.zeroavia.com/press-release-25-09-2020 (Accessed September 25, 2020).
Keywords: hydrogen, fuel cells, gas turbine, propeller propulsion, jet propulsion, subsonic aviation
Citation: Boretti A (2023) Progress of hydrogen subsonic commercial aircraft. Front. Energy Res. 11:1195033. doi: 10.3389/fenrg.2023.1195033
Received: 27 March 2023; Accepted: 03 May 2023;
Published: 16 May 2023.
Edited by:
IMR Fattah, University of Technology Sydney, AustraliaReviewed by:
Wei Zhu, Wuhan University, ChinaS. M. Ashrafur Rahman, Queensland University of Technology, Australia
Copyright © 2023 Boretti. This is an open-access article distributed under the terms of the Creative Commons Attribution License (CC BY). The use, distribution or reproduction in other forums is permitted, provided the original author(s) and the copyright owner(s) are credited and that the original publication in this journal is cited, in accordance with accepted academic practice. No use, distribution or reproduction is permitted which does not comply with these terms.
*Correspondence: Alberto Boretti, YS5hLmJvcmV0dGlAZ21haWwuY29t