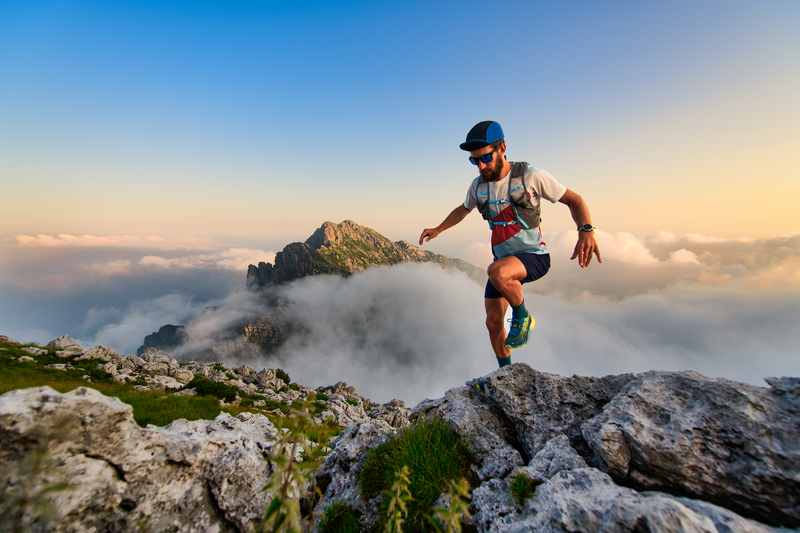
95% of researchers rate our articles as excellent or good
Learn more about the work of our research integrity team to safeguard the quality of each article we publish.
Find out more
EDITORIAL article
Front. Energy Res. , 06 March 2023
Sec. Hydrogen Storage and Production
Volume 11 - 2023 | https://doi.org/10.3389/fenrg.2023.1149688
This article is part of the Research Topic Advanced Water Splitting Technologies Development: Best Practices and Protocols View all 20 articles
Editorial on the Research Topic
Advanced water splitting technologies development: Best practices and protocols
As the level of deployment and utilization of renewable energy sources, including wind and solar, continues to rise, large-scale, long-term energy storage technologies that could accommodate weekly and seasonal energy fluctuations will play a significant role in the overall deployment of renewable energies in the future. Harnessing and storing renewable energy resources via electrochemical, photoelectrochemical, or thermochemical processes by converting renewable energy into sustainable (energy storage) fuels have the potential to meet the long-term, terawatt scale energy storage challenge. Renewable hydrogen production is the cornerstone for sustainable fuel production and deep decarbonization of multiple sectors in our society. Cost-competitive clean hydrogen provides value to applications, such as 1) in the transportation sector for fuel cell vehicles, 2) in the electric grid sector for system stability and load balancing, and 3) in the industrial sector with metal refineries, cement production, and biomass upgrading (carbon-free fertilizer production). In addition, coupling clean renewable hydrogen with the carbon and nitrogen cycles enables known and well-established thermal-chemical processes to generate renewable hydrocarbon fuels and ammonia. The Advanced Water Splitting Technologies (AWST): low temperature electrolysis (LTE), high temperature electrolysis (HTE), photoelectrochemical (PEC) and solar thermo-chemical hydrogen (STCH) provide four unique and parallel approaches to produce low cost, low greenhouse gas (GHG) emission hydrogen at scale (Figure 1). Cost competitive clean hydrogen production using these four technologies is a current high priority focus for governments and industry. In June of 2022, the U.S. Department of Energy (DOE) launched the first in a series of Earthshot Initiatives. The Hydrogen Shot, “1 1 1” aims to reduce the cost of clean hydrogen by more than 80% to one dollar per one kilogram in 1 decade ($1/kg H2). The European Green Deal and the International Energy Agency (IEA) have implemented a strong focus on green hydrogen production for a clean and secure energy future.
In LTE, an electrochemical process produces hydrogen gas from water. H2 is produced at the cathode and O2 at the anode electrochemically under a voltage bias, typically operating between 50 and 80°C. In commercial systems, either a membrane or a porous fabric-like separator separates the cathode from the anode. Among various LTE configurations, three types of LTE systems include alkaline, proton exchange membrane (PEM) based, and hydroxide exchange membrane based water electrolysis. These configurations are the most utilized systems. Alkaline and PEM electrolyzers have been deployed commercially at multi-megawatt scale (thousands to tens of thousands of metric tons per year H2 production capacity for the largest projects) while hydroxide membrane electrolyzers are mostly pre-commercial.
The operating principle of HTE is similar to LTE. The HTE cells include a cathode for water reduction and hydrogen production, an anode for oxygen generation, and a solid ceramic electrolyte for selective transport of oxygen or proton ions at elevated temperatures of 400–850°C. HTE systems operate at low voltages of ≤1.3 V and current densities of 1–1.5 A cm-2 and can achieve 90%–95% stack electrical efficiency. Current SOEC technology is pre-commercial; the largest demonstrated scale for HTE is 0.72 MW projected to increase to a 2.6 MW demo plant in 2023, which corresponds to a generation rate of ∼ 525 metric tons per year.
While LTE and HTE use renewable electrons from solar, wind, and/or hydroelectric power (or carbon free electrons from nuclear) to generate clean hydrogen, PEC and STCH produce clean H2 directly from sunlight. PEC water-splitting integrates light absorption, photo-generated carrier transport, electrocatalysis, ionic transport, and product separation in an integrated photoelectrochemical device for hydrogen generation, (water plus sunshine-in and renewable-hydrogen-out solar panel). In a more in-depth perspective: PEC devices often operate at much lower current densities compared with LTE or HTE to match the solar flux, while high current density PEC devices with an operating current density close to 1 A cm-2 have been demonstrated in conjunction with solar concentrators. A portfolio of PEC devices have achieved a solar to hydrogen conversion efficiency of >10% with an overall device stability ranging from tens of hours to hundreds of hours. For the moment, PEC devices have only been demonstrated at a laboratory scale <<1 kg/day H2.
In contrast, solar thermochemical hydrogen (STCH) cycles use the heat from sunlight to produce hydrogen and oxygen from water. A popular STCH pathway uses two-step redox active metal oxide (MOx) thermochemical cycles to produce H2 and O2 sequentially in two different chemical reactions. STCH has been demonstrated at −1 kg/day. In the two-step cycle, a redox-active MOx is first heated, generally using concentrated solar radiation, to temperatures typically exceeding 1500 K and often close to 1800 K at a low partial pressure of oxygen (pO2), at which point the material becomes reduced to a more O-poor metal oxide. In the second step, the reduced metal oxide cools to a temperature where re-oxidation is favorable when exposed to superheated H2O vapor (aka steam), which leads to water splitting and regeneration of the original MOx. Off-stoichiometric metal oxides form and fill oxygen vacancies during the thermal reduction and water splitting (or re-oxidation) steps, respectively, without undergoing major structural bulk phase transitions, thus promoting faster kinetics, cyclability, and durability.
It is important to understand that LTE, HTE, PEC, and STCH water splitting technologies have different technical readiness levels and face different technical challenges. However, a common and absolutely vital need in each and all of these (and many other) basic research to commercial development efforts, is a concerted effort to come together and produce a path for the most trust-worthy, reliable, and reproducible results. This coordination will lead ultimately to the most rapid development of these life-changing beneficial technologies.
In this light, Benchmarking including the developing and documenting the best practice procedures and protocols are vital for creating an advanced R&D foundation for the broader research community in all four pathways and, potentially, in the R&D community in general. The development of standard protocols that integrate and harmonize independently funded work across the world is vital for accelerating the materials development as well as for the commercialization of each technology. This statement is particularly true given the enormous new interest in clean or green hydrogen. There exists at this moment a critical need for consistency in testing protocols, reference materials, and standard testing cells within and across all four technological pathways. To present a clear perspective, standard protocol development and the use of reference materials and cells for testing at the materials level, component level, and device level have proved to be critical in the development of similar technologies including fuel cells, advanced batteries, etc. Standard protocols, when successfully established and utilized within and across communities and technologies, can significantly reduce inconsistencies in results from different research groups due to different and varying testing procedures. The incorporation and adherence to a set of standardized protocols enables true cross comparison among newly discovered or developed materials and components. Standard protocols can also quantitatively gauge the progress made in each technology and are a vital part of developing an overall roadmap designed to achieve high level $/kg H2 goals that are desperately needed.
Through this collaborative Benchmarking, Protocol, Best Practices, and Road mapping project, an improved efficiency, reliability, and most effective pathway towards solving serious future energy issues has taken form and is being implemented. Critical for this effort was bringing the four technologies together to effectively facilitate cross-cutting opportunities. Low TRL technologies with a current emphasis on materials level development can learn scale up strategies, balance of system designs, and methods of estimating cost from higher TRL technologies, while high TRL technologies with the current emphasis on the system level development can benefit from fundamental materials knowledge gained in low TRL technologies.
To provide a specific example of the benefits and success in these efforts; the standard protocols that benchmark membrane properties, such as water content, gas permeability, and ion exchange capacity in LTE, can be adapted by the PEC community to include additional considerations on stability under illumination and conductivity for cations and anions other than proton or hydroxide for near-neutral pH operations. Similarly, HTE is learning from LTE about cell and system scale-up, testing on comparable cell and device levels, and corresponding testing protocols.
At the same time, through this widening of communications and support, expertise in PEC has opened new possibilities for LTE. For example, earth abundant electro-catalytic materials development in the PEC community is largely translatable to LTE and can in principle provide options for non-platinum group metal (PGM) catalysts for incorporation in LTE. There is a lot of similarities in materials requirements in HTE and STCH. Examples of common R&D interests in system level considerations for PEC and STCH include sunlight spectrum utilization, overall solar to hydrogen conversion efficiency, operational conditions, and constraints of each system under sunlight, can cross-fertilize advances in both technologies. Cross-communication, collaboration, and unilateral support of R&D advancements towards a clean energy future is vital for all our futures. This project and this publication platform provide an opportunity to collaborate and collectively improve the community’s R&D efforts to ensure that we are all working most effectively towards a sustainable clean energy future.
Research Topic in Frontiers in Energy: This on-line, peer reviewed journal, provides open access to rapidly developing standardized testing protocols for the AWST R&D community. In this issue, authors offer a collection of standard protocols in the fields of LTE, HTE, PEC, and STCH advanced water-splitting technologies for clean hydrogen production. To track and report on progress and to set global priorities for advanced water splitting hydrogen research, this issue is a step intended to address the need to gain consistency within and across individual technology pathways so that researchers can reliably evaluate and compare the potential for each pathway. In addition, time is not our friend, and the lack of consistency and agreed upon benchmarks, protocols, standards, or roadmaps creates a large activation barrier for entry, for communicating to decision makers, and for general outreach.
In the interest of lowering the barriers and disseminating best practices in characterizing and benchmarking advanced water splitting materials, creating a foundation in accelerated materials, device, and systems research, development and deployment for the broader research community, this research topic asked the community for articles that describe comparisons, materials screening, characterization protocols, benchmarks, techno-economics, system analyses, and roadmaps for any and all advanced water splitting pathways, in which the primary energy is renewable (or at least carbon-free).
Current LTE protocols focus on ex-situ material screening methods as a first step in any new exploration to ensure that minimum criteria are met before investing additional time and resources in more complex tests. Some of these measurements are very technique dependent and require careful methods to obtain accurate results, such as catalyst activity via rotating disk electrodes. Other property measurements include ion exchange capacity, and oxidative stability of anion exchange membranes, and physical properties of porous transport layers. A final protocol addresses standard teardown of full cells for analysis. These protocols form a framework to build from for in cell component testing and durability.
PEC currently has five topical protocol reports that include benchmarking solar-to-hydrogen conversion efficiency of photoelectrodes, measurements of ion exchange membranes for solar fuel applications, comprehensive evaluation of optical, electrical, photoelectrochemical and spectroscopic properties of protective coatings, incident photo-to-current efficiency measurements and long term photoelectrode stability measurement protocols for PEC water splitting.
Presented HTE protocols focus on methods of solid oxide cell materials properties’ characterization, such as measuring sample density, determining oxide electrical properties, separating the contributions of different charge carriers to the total conductivity as well as describing effective ways of solid oxide cell sealing, operating, and leak testing. In addition, one of the protocols compares several different steam generators to ensure stable and reliable steam supply to HTE, which is vital to ensure a uniform hydrogen production rate and to report degradations accurately.
STCH protocols in this issue include one paper on performance indicators (“Performance Indicators for Benchmarking Solar Thermochemical Fuel Processes and Reactors.”) The solar reactor for operationalizing STCH is the key component and the performance of that reactor can be the deciding factor in assessing technical and economic feasibility. Important indicators discussed in this paper are conversion, selectivity, efficiency, and stability. The issue also includes a paper on determining the off-stoichiometry with temperature and partial pressure of oxygen (“A Thermogravimetric Temperature-Programmed Thermal Redox Protocol for Rapid Screening of Metal Oxides for Solar Thermochemical Hydrogen Production,”) The authors show that temperature-programmed thermal reduction can provide a simple thermogravimetric analysis-based single-run experiment that measures the redox behavior of a specimen under thermal reduction and reoxidation conditions relevant to STCH. Lastly, the issue includes a paper on synchrotron-base characterization (“Synchrotron-based techniques for characterizing STCH water-splitting materials.”) Synchrotron radiation is a powerful tool for characterizing STCH materials. X-ray absorption spectroscopy can identify those cations that are redox active and the extent of reduction in quenched conditions.
In summary, this issue contains nineteen articles and ninety different authors. Additional protocols are still in development and will be published later. We look forward to extensive international use of the protocols presented in this Research Topic and new ones as they become available. We also highly encourage participation by the wider community in improving and giving feedback on the current protocols and the continued creation of advanced protocols for AWST materials, component, and systems research and development.
KA, OM, ES, and CX contributed to the manuscript equally.
Authors MC, KA, and GR are employed by Nel Hydrogen. KJG was employed by H2 Technology Consulting.
The remaining authors declares that the research was conducted in the absence of any commercial or financial relationships that could be construed as a potential conflict of interest.
All claims expressed in this article are solely those of the authors and do not necessarily represent those of their affiliated organizations, or those of the publisher, the editors and the reviewers. Any product that may be evaluated in this article, or claim that may be made by its manufacturer, is not guaranteed or endorsed by the publisher.
Keywords: hydrogen, water splitting, benchmarking, low temperature electrolysis, high temperature electrolysis, photoelectrochemical, solar thermochemical water splitting, protocol
Citation: Bulfin B, Carmo M, Van de Krol R, Mougin J, Ayers K, Gross KJ, Marina OA, Roberts GM, Stechel EB and Xiang C (2023) Editorial: Advanced water splitting technologies development: Best practices and protocols. Front. Energy Res. 11:1149688. doi: 10.3389/fenrg.2023.1149688
Received: 22 January 2023; Accepted: 14 February 2023;
Published: 06 March 2023.
Edited and reviewed by:
Fenglong Wang, Shandong University, ChinaCopyright © 2023 Bulfin, Carmo, Van de Krol, Mougin, Ayers, Gross, Marina, Roberts, Stechel and Xiang. This is an open-access article distributed under the terms of the Creative Commons Attribution License (CC BY). The use, distribution or reproduction in other forums is permitted, provided the original author(s) and the copyright owner(s) are credited and that the original publication in this journal is cited, in accordance with accepted academic practice. No use, distribution or reproduction is permitted which does not comply with these terms.
*Correspondence: Kathy Ayers, a2F5ZXJzQG5lbGh5ZHJvZ2VuLmNvbQ==; Olga A. Marina, T2xnYS5NYXJpbmFAcG5ubC5nb3Y=; Ellen B. Stechel, RWxsZW4uU3RlY2hlbEBhc3UuZWR1; Chengxiang Xiang, Y3h4QGNhbHRlY2guZWR1
Disclaimer: All claims expressed in this article are solely those of the authors and do not necessarily represent those of their affiliated organizations, or those of the publisher, the editors and the reviewers. Any product that may be evaluated in this article or claim that may be made by its manufacturer is not guaranteed or endorsed by the publisher.
Research integrity at Frontiers
Learn more about the work of our research integrity team to safeguard the quality of each article we publish.