- 1Mineral Resources Division, Korea Institute of Geoscience and Mineral Resources (KIGAM), Daejeon, Republic of Korea
- 2School of Engineering, University of Guelph, Guelph, ON, Canada
- 3Resources Utilization Division, Korea Institute of Geoscience and Mineral Resources (KIGAM), Daejeon, Republic of Korea
Thorium is a naturally occurring radioactive element that has been identified as a potential alternative fuel for nuclear energy production. Additionally, thorium-based nuclear reactors have inherent safety features that reduce the risk of nuclear accidents and proliferation. As a result, there has been growing interest in the development of thorium-based nuclear energy as a viable alternative to fossil fuels. This paper looks at the present status of thorium nuclear fuel technology, providing an overview of thorium as a prospective natural resource for future energy, the global availability of mineral supplies, and discusses the technical, economic, and environmental factors that may influence its implementation. Potential advantages and challenges critical to further development associated with thorium-based nuclear energy are highlighted as well, and an outlook on its future prospects is provided. Thorium offers advantageous physical and chemical properties over uranium, has a higher energy density, and produces less waste, in addition to its greater natural abundance, making it to be considered a “future nuclear fuel”. There are concerns about the cost and scalability of thorium-based nuclear energy, with uncertainty around the cost to develop, build, and operate thorium reactors, as it has not yet been demonstrated in large-scale commercial reactors—although almost all current reactor types have been built and run using thorium—as it is the case with Uranium-based nuclear technology—the dominant form of nuclear energy for over half a century, having received much more investment and attention than thorium-based technology. Thorium has the potential to contribute towards a more sustainable nuclear industry, including lower lifecycle emissions and more efficient resource utilization, but for this, an acceleration of efforts to date is needed to ensure that this becomes an important climate change stabilizing wedge by the mid-21st century.
1 Introduction
1.1 Global electricity production, accessibility, and lifecycle GHG emissions
The major sources of global electricity production are crude oil followed by coal, natural gas, biofuels/waste, nuclear, and hydro (Figure 1) (British Petroleum, 2022; EMBER 2022; 2023). Despite historical gaps in access to electricity among the world’s regions and between urban and rural populations, the lack of accessibility to electricity over the globe has decreased, but in regions of greater population growth the gap is still proportionally large (International Energy Agency, 2011). The lifecycle of GHG emissions from various electricity sources is presented in Figure 2, where the highest emissions originate from lignite, coal, oil, and solar PV sectors, whereas the sectors of nuclear, hydroelectric, and wind energies are categorized as having substantially lower emissions (World Nuclear Association, 2022a). Understanding the lifecycle GHG emissions of a product or process is important for evaluating its environmental impact by quantifying the emissions according to each stage of a product or process’s lifecycle. As an example, for a car, it would include the emissions from the extraction of raw materials for the car’s components, the emissions from the manufacturing process, the emissions from transporting the car to the dealership, the emissions from the fuel used during its operation, and the emissions from the car’s disposal at the end of its life. This also makes it possible to identify areas where emissions reductions can be achieved, such as through more efficient manufacturing processes, the use of cleaner energy sources, or the development of more sustainable end-of-life disposal methods. Nuclear reprocessing is included in the lifecycle GHG emissions of nuclear energy. It consists in extracting usable nuclear materials from spent nuclear fuel, which allows for the recovery and reuse of valuable nuclear materials and reduces the amount of waste that requires disposal. This process, however, requires significant amounts of electricity and other energy inputs, which in consequence, contribute to greenhouse gas emissions. There are also environmental and safety concerns associated with the handling and storage of nuclear waste and the reprocessing of spent nuclear fuel that have to be considered.
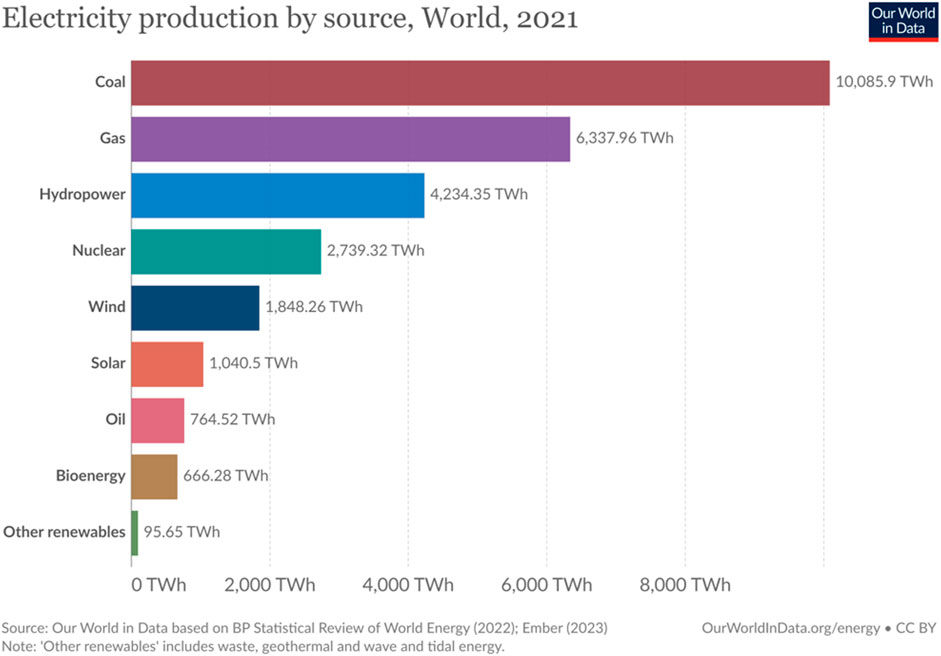
FIGURE 1. Global electricity production by various sources year 2021 (data adapted from British Petroleum (2022); Ember (2023)).
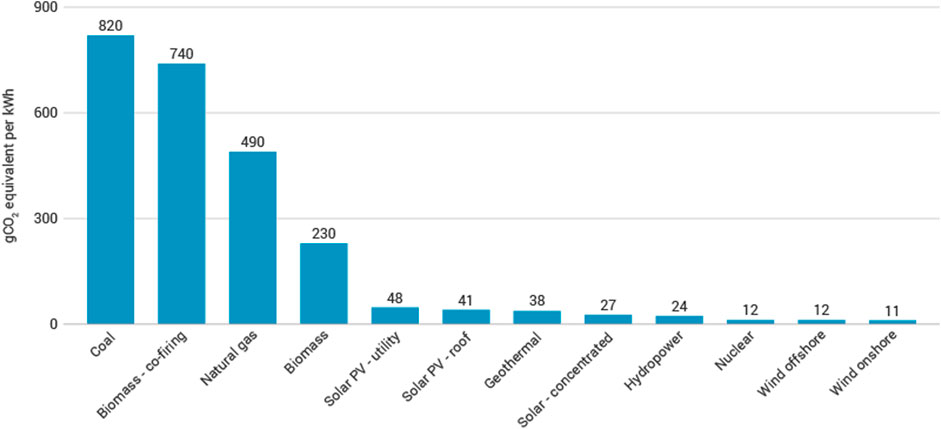
FIGURE 2. Average life-cycle CO2 equivalent emissions (data adapted from World Nuclear Association (2022a)).
Those values are in accordance with the comprehensive study of (Lenzen, 2008), who proposed that lifecycle emissions for LWR (light water reactor) and HWR (heavy water reactor) to be 10–130 g CO2-e/kWhel (average 65 g CO2-e/kWhel). These values compare favorably to those of fossil technologies (600–1,200 g CO2-e/kWhel) and solar photovoltaic or solar thermal power (90 g CO2-e/kWhel), though they are measurably higher than for wind turbines and hydroelectricity (15–25 g CO2-e/kWhel). Nevertheless, nuclear energy is an important component of the transition to more sustainable energy (Office of Nuclear Energy, 2021), especially in the case of next-generation reactors and fuels (Ho et al, 2019), and thus can potentially become a critical climate stabilization wedge (Haque et al, 2021).
1.2 Global nuclear reactors by 2030
Countries like China and India, where energy demands have rapidly grown and are expected to continue growing, plan to build a large number of nuclear reactors by the year 2030 to fulfill future demand. It is expected that by the year 2040, the amount of nuclear reactors in the world is expected to reach 622—considering 123 reactors closing by then, and 308 coming online—from 437 in 2021, achieving an electricity generation of 871 GWe by 2050, up from 389.5 GWe in 2021 (Figure 3) (International Atomic Energy Agency, 2023; World Nuclear Association, 2022b; World Nuclear Association, 2023). It is important to point out the situation in France, where half of its 56 reactors are about 40 years old, and many are unusually down for maintenance—by September 2022, no fewer than 25 reactors were out of action: ten for routine maintenance, the rest for corrosion analysis or repairs (The Economist, 2022). These trends dictate that the future need for nuclear fuel will be substantially higher compared to the present situation, but this is cause for concern given geopolitical instabilities and the environmental impact that follows new mining activity. These are key factors to foresee the greater thorium requirement for future needs (Loiseaux et al, 2022).
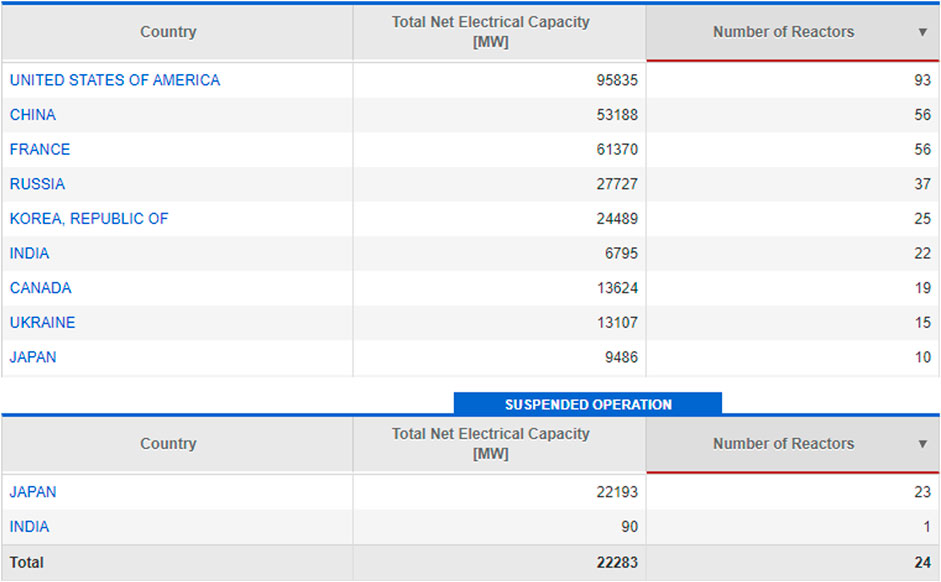
FIGURE 3. Top 10 countries with the most nuclear reactors (data adapted from International Atomic Energy Agency (2023)).
2 Uranium versus thorium
Until now, the nuclear energy field has mainly depended upon uranium resources. Six key factors project the future needs for alternative nuclear fuel, these are: global energy resources, global electricity situation, global non-accessibility of electricity, lifecycle of greenhouse gas emissions, global non-renewable energy resources, and nuclear reactor technology condition (tied to estimations for future needs) (Warner and Heath, 2012). Thorium (232Th) is a fertile radioactive element, i.e., can capture a neutron to become 233Th, which through double beta decay becomes fissile 233U, a nuclear fuel (Banerjee et al, 2011). It is available on Earth’s crust at 3 to 4 times more than uranium (Schaffer, 2013), and is thus an important resource for future energy (Salehuddin et al, 2019). The global reserves of uranium and thorium are presented in Figure 4 (World Nuclear Association, 2020b; World Nuclear Association, 2022b). The top five nations for uranium reserves are Australia, Kazakhstan, Canada, Russia, and Namibia (accounting for 67% of world reserves, i.e., two-thirds), whereas the top five countries for thorium reserves are India, Brazil, Australia, USA, and Egypt (accounting for 47% of world reserves) (Figure 4). Notably, thorium reserves are more widespread than those for uranium, which geopolitically can be beneficial but can also signify a lower number of large concentrated reserves and hence challenges for economic exploitation. Some factors put thorium in a privileged position: its contribution to the burning of excess plutonium, less generation of long-lived waste, higher burn-ups and temperatures capability, and sustainability, considering uranium reserves [Lung and Gremm, 1998].
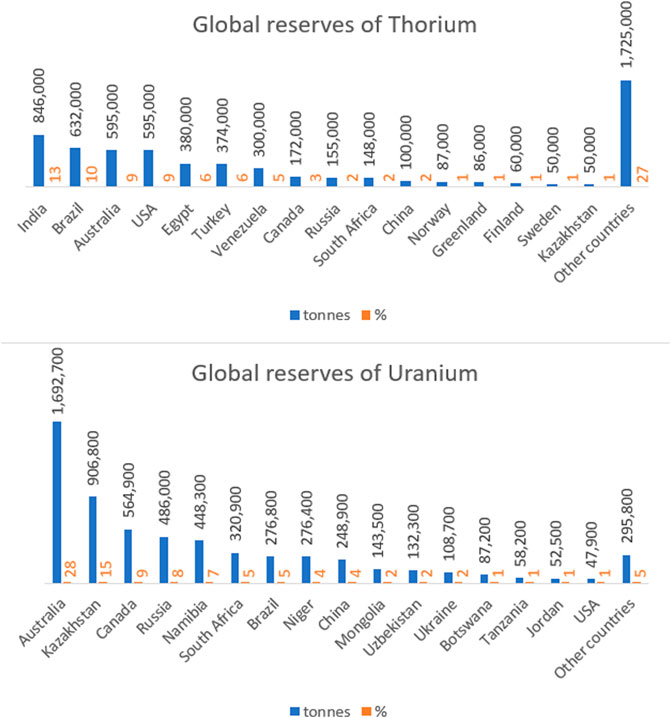
FIGURE 4. Global reserves of uranium (data adapted from World Nuclear Association (2022c)) and thorium (data adapted from World Nuclear Association (2020a)).
Global nuclear energy consumption has been estimated in terms of million tonnes oil equivalent for seven regions of the world: North America, South/Central America, the Commonwealth of Independent States (CIS) in Eurasia, Middle East, Africa, and Asia Pacific (Figure 5) (British Petroleum, 2019). It is clear that nuclear power has been concentrated in regions that have large population densities and high-income countries in combination with reduced access to fossil and renewable energy. This makes for a clear contrast between North America and Europe versus South/Central America, Middle East, and Africa. The CIS and Asia Pacific regions have complex factors that place them between these two extremes. Overall, worldwide nuclear power generation has slightly increased, with the construction of new power plants in China. In lieu, there has been reactors shutdown in Europe. A critical factor that will determine how this balance shifts in coming decades is the availability of uranium reserves, which are expected to be depleted leading to a shift toward thorium resources for future generations. Another factor is pricing pressures on electricity supply. Electricity costs for various energy sources are presented in Figure 6. With the need for carbon capture and sequestration factored in, it has been estimated that the emissions abatement cost for nuclear power is between USD 10.4 and USD 15.7 per tonne CO2(eq) lower than for coal-fired and gas-fired power plants, respectively (Mendoza España and Bromley, 2019). Uranium, however less satisfactory regarding energy production, is, as a general rule, cheaper. Thorium-uranium fuel is expensive, having a break-even cost with uranium that would only be reached if the natural uranium price more than doubled (Serfontein, 2014). It has to be noted, however, that there are strategic cases, like India and other countries in which thorium is plentiful compared to uranium. [Lung and Gremm, 1998] In addition, the revenue generated from electricity sales combined with the reactor downtime refueling cost shows it can be advantageous - a 14.8% savings if compared in equivalent time frames (three thorium-based x four uranium cycles) (Du Toit, 2014). (Ashley 2014) presented normalized fuel cycle costs of 0.77¢/kWh for a Uranium-fueled European Pressurized Reactor (EPR) as a baseline comparison against Thorium-Uranium fueled reactors: another EPR (0.90/kWh), an Advanced Heavy Water Reactor (AHWR, 0.99¢/kWh), and a General Atomics’ Gas-Turbine Modular Helium Reactor (GT-MHR, 2.37¢/kWh).
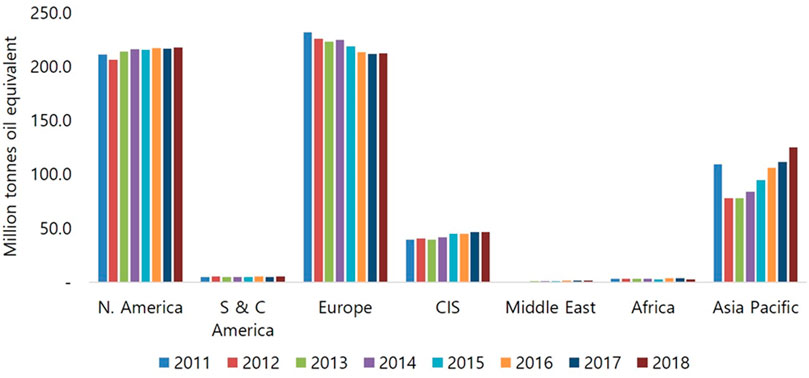
FIGURE 5. Global nuclear energy consumption in million tones oil equivalent; data estimated by BP statistical survey (data adapted from British Petroleum (2019)) (N. America, North America, USA, Canada, Mexico; Total CIS, Azerbaijan, Kazakhstan, Russian Federation, Turkmenistan, Uzbekistan, Other CIS; S & C America, South and Central America).
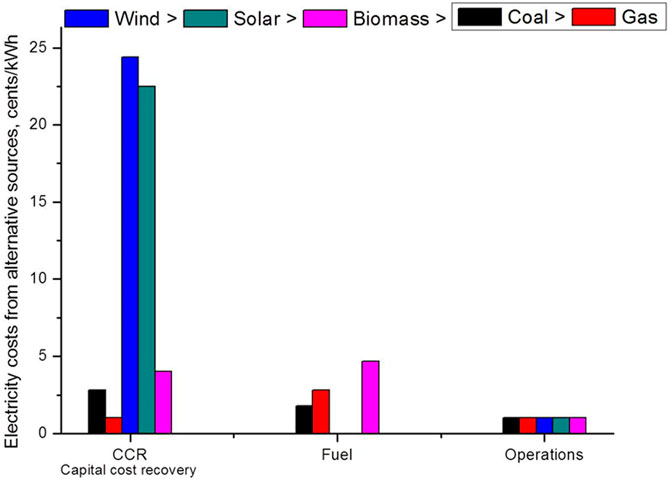
FIGURE 6. Electricity costs from various energy sources (data adapted from Hargraves (2012)).
There are several advantages of using thorium as a nuclear fuel compared to uranium. Thorium oxide has a higher melting point (3300ᴼC) compared to uranium oxide (2865ᴼC), and thorium oxide has better thermal conductivity, a lower rate of fission gas release (despite higher fission gas production per fission event), good radiation resistance, and dimensional stability up to 38 MWd/kg (Banerjee et al, 2011; György and Czifrus, 2017). UO2 easily oxidizes to U3O8 and UO3, but ThO2 is relatively inert, hence, thorium oxide is much more stable than uranium oxide, and ThO2-based fuels are expected to have better in-pile performance than UO2 and UO2-based mixed oxide (International Atomic Energy Agency, 2005; Bell et al, 2019); (Hania, 2012) also mentions that considering the focus on the burning of Pu in LWRs or HWRs, further irradiation testing of (Th,Pu)O2 in the 6%–10% Pu concentration range to high plutonium burnups is warranted. Fabrication procedures for thorium mixed oxides are analogous to that of UO2 and (U,Pu)O2, with the main technical challenges lying in the reprocessing steps, and subsequent (shielded) refabrication. After reprocessing, Th228 (t1/2 = 1.9 years) is found, making the output radioactive for decades, so blending the reprocessed uranium oxide with fresh ThO2 and considering the spent thoria as waste is suggested.
The formation of U232 via (n,2n) reactions with 232Th, 233Pa, and 233U, makes a great proliferation-resistance impact (International Atomic Energy Agency, 2012). The half-life of U232 is 68.9 +-0.4 years [Tuli, 2011] and the daughter products have a very short half-life, with some products, e.g., 212Bi and 208Tl, emitting strong gamma radiations. From these considerations, it can be said that Th-based fuels can be used as an intrinsic proliferation-resistant barrier to highly enriched uranium (HEU) and weapons-grade plutonium (WPu) (International Atomic Energy Agency, 2005); (Kazimi, 2003) estimated that Th-based fuels could cost ±10% when compared to conventional U-based fuels, with the biggest uncertainty being the cost of 235U-rich seed, the cost of the fuel assemblies (which need to be designed to withstand greater exposure to heat and radiation in the core of the reactor), and the potential future savings due to a reduction in spent fuel amounts needed to be stored. HWR has a more flexible neutron economy compared to LWR, as a result of lower neutron absorption by Deuterium, in addition to presenting the necessity for online refueling, while also having a thermal neutron spectrum, which is an attractive characteristic for the thorium fuel cycle (Ault et al, 2018).
3 Thorium as future nuclear fuel
Thorium has some advantageous physical and chemical properties over uranium and that can make a reason to use thorium as a ‘future nuclear fuel’ (International Atomic Energy Agency, 2005), which has been discussed previously. Thorium is considerably more abundant than uranium in the Earth’s crust, so the use of thorium in power reactors has been considered since the start of nuclear energy in the 1950s (Kazimi, 2003). Thorium is said to be ‘fertile’ rather than ‘fissile’, as a quantity of Th232 transforms into U233 by absorbing neutrons within a reactor (Alexander et al, 2020). The breeding of U233 from thorium is much more efficient than the breeding of Pu239 from U238 because of less production of non-fissile isotopes, which plays an important role in assessing the breeding capability of a nuclear reactor design, as a measurement of the potential for converting non-fissile material into fissile material through the use of neutron irradiation. The International Atomic Energy Agency (2005) mentions the absorption cross-section for thermal neutrons of Th232 (7.4 barns) is nearly three times that of U238 (2.7 barns), resulting in a higher conversion (to U233) with Th232 than with U238 (to Pu239). The high neutron yield per absorption ratio can be identified by looking at the fission-to-capture ratio of about 10 for U233, which is only about 2.5 for Pu239 (Hania, 2012). However, it is important to point out that the theoretical physics breeding is different from the actual engineering breeding–which includes reprocessing losses and large uncertainties in nuclear data of thorium fuel cycle -, meaning the effective spectrum averaged cross sections data would depend on the type of reactor design and the associated neutron energy spectrum. Ganesan (2016) mentions under-prediction for the production of U232, given although it is possible to apply nuclear data for the cross-section of isotopes of the thorium fuel cycle to traces of thorium in natural uranium rods, it is not for the irradiation of bulk thorium rods used for power flattening in their PHWRs. There are programs, such as the IAEA CRP, that took place between the years of 2010–2014, on improving nuclear data of prompt fission neutron spectra. Gunsing (2016) references the EXFOR database, with experimental nuclear reaction data fed from the world by the International Network of Nuclear Reaction Data Centers (NRDC), as well as the contributions from the Neutron Time-of-Flight Facility (n_TOF) at CERN, measuring and recording records data, and developing instrumental, detection and beam imaging techniques, having measured fission cross sections of Th232, U233, U238, among others, through different fission detectors. Moreover, thorium compounds, such as silicon-doped cubic ternary thorium phosphides (ThSixP1-x, where x = 0, 0.25, 0.5, 0.75, 1), from Generation-IV nuclear breeder—theoretical—systems, have unique chemical and physical properties such as high melting point, density thermal conductivity, and oxidation resistance, being prospective fuel materials for cleaner and safer nuclear energy (Bell et al, 2019; Siddique et al, 2021). A reactor is defined as a breeder if it produces more fissile material than it consumes - i.e., even with expected neutron losses, more nuclear fuel can be made from the additional neutrons than fissioned. In theory, mastering nuclear fission thorium breeder cycle reactors could increase the amount of fissile material by over 100x (Dittmar, 2018).
Combinations of thorium and highly enriched uranium have been tested in gas or water-cooled reactors, and several countries such as India, Germany, the UK, France, Japan, Russia, Canada, Brazil, and China have tested the use thorium-based fuels for their reactors (Jiyang et al, 2004; Şahin et al, 2004; Bell et al, 2019; Mendoza España and Bromley, 2019), while some countries like Türkiye are considering it (Demirbaş, 2005). However, the existence of natural resources, the cost of exploiting those resources, a country’s international trade balance position, and its economic growth policies, the profitability of the electrical power and nuclear industries are important factors that may help or prevent the adoption of thorium-based nuclear fuel technology, making technical development critical to overcoming economic challenges (Mendoza España and Bromley, 2019). For example, the excellent neutron economy in Canada deuterium uranium (CANDU) reactors potentially enables the implementation of thorium fuel cycles in these systems as a strategy to conserve uranium fuel resources and as an alternative to fast breeder reactors (FBR) (Şahin et al, 2004). An FBR typically uses uranium and/or plutonium as fuel and liquid sodium as a coolant, having the potential to produce electricity more sustainably and efficiently than conventional nuclear reactors. However, due to the large availability in its territory, India has been over essential microstructural, mechanical and. thermophysical properties of thorium-uranium alloys, showing great interest in Thorium-based metallic fuel applications to LMFBRs (Ramanna, 1986), and its excellent safety features—more specifically, (Th, Pu)O2 containing from 20% to 80% PuO2 (Lainetti, 2017).
3.1 Global thorium resources
Hans M.T. Esmrak found an unknown black mineral on the island of Lovo, Norway, and sent it to Swedish chemist Jons Jakob Berzelius, who identified that mineral as a new element and named it Thorium after the Nordic God Thor, in the year 1828; after that, the mineral was described as thorium-silicate thorite (International Atomic Energy Agency, 2019). Major natural occurrence of thorium is an oxide (thorianite), silicate (thorite), and more than 60 minerals with thorium in oxides, hydroxides, silicates, phosphates (monazite), carbonates (rare) (Clarke et al, 2000). The most common source of thorium is a rare earth phosphate mineral, monazite (Salehuddin et al, 2019). However, the recovery of thorium from these minerals is challenging due to the co-existence of thorium, uranium, and rare earth elements (REE), calling for the development of new solid extraction techniques that have high selectivity and capacity (Xiong et al, 2020). India is the only country today extracting thorium from monazite which has been announced (International Atomic Energy Agency, 2019). Another concern is the presence of naturally occurring impurities in thorium-based fuels, but studies have found that the effect of impurities at their typical levels has a limited impact on fuel performance (exit burnup and radiotoxicity), thus not necessitating further refinement (Alexander et al, 2020).
3.2 Major thorium deposits
Major deposit types of thorium are: placer (∼2.2 million t Th, ∼35%), carbonatite (∼1.8 million t Th, ∼29%), vein type (∼1.5 million t Th, ∼25%), alkaline rocks (∼0.6 million t Th, ∼9%), unknown types (∼0.1 million t Th, ∼2%) (International Atomic Energy Agency, 2019). Carbonatite, peralkaline rocks, and associated veins are characteristic of silica under-saturated magmatic provinces. Thorium is mined in conjunction with uranium, thus the estimates of reserves for thorium are made based on uranium content. Thorium is typically found in the minerals thorite, thorianite, and monazite, however, monazite is the only mineral from which thorium is currently mined. A maximum of these monazite deposits are found in placer deposits in India and the United States. In addition to the presence of thorium in primary minerals, Chadirji-Martinez et al, (2022) point to the presence of Th as a substitution in REE sites and anhydrite, and such substitutions have implications for physical and chemical recovery in terms of extraction strategies needed depending on the speciation split of Th in the ores. With limited dedicated experimental research on thorium recovery from minerals, geochemical modeling is a powerful tool that can be used to screen potential extraction, recovery, and purification processes (Khalidy and Santos, 2021). Examples of thorium geochemical modeling efforts include studies on determining the speciation of co-existing thorium and uranium in natural waters from a contaminant perspective (Lofts et al, 2015), and in assessing the stability and mobility of thorium in radioactive waste disposal, storage, and site remediation (Hummel, 2005).
3.2.1 Europe and Türkiye
Main deposits of thorium in Europe are found in Greenland, Finland, Norway, and Türkiye, though as of 2019 no mining for thorium is undertaken in Europe. This may change in the future as on the 24th of October 2013 the Greenland Parliament lifted a moratorium on mining radioactive elements (International Atomic Energy Agency, 2019). The type of deposits found in Europe are: vein deposits, peralkaline intrusions, carbonatite, volcanic rock of acidic to alkaline composition, and placers (International Atomic Energy Agency, 2019). Of the ∼1 Mt thorium in Europe and Türkiye, only about 10% is estimated to be possibly economically available, assuming by-product recovery, but under current market conditions (as of 2019), production of thorium in Europe is not expected.
3.2.2 Americas
Canada has reported 44 000 t Th of inferred resources that are estimated to be recoverable at less than USD 80/kg Th, and an additional prognosticated resource of 128 000 t Th [OECD-NEA, 2010]. Most of the thorium resources are associated with uranium, either in the Blind River-Elliot Lake area or in pegmatite or carbonatites, and no mining activities presently exist to recover these resources (International Atomic Energy Agency, 2019).
In the United States, thorium resources primarily occur in veins, with an estimated 113 000 t Th inferred and ∼234 000 t Th prognosticated (Van Gosen et al, 2009). The co-occurrence of thorium with REE can make the former a valuable by-product if the latter is mined in the near future. Carbonatites and alkaline intrusions possess limited amounts of thorium and thus are not currently considered economically potential sources unless associated as by-products of primary mining (International Atomic Energy Agency, 2019). One example is the exploration of rare earth oxides (REO) in the carbonatite of Mountain Pass, where 29 Mt of carbonatite ore (bastnaesite mineral) containing ∼9% of total REO and 0.02%–0.04% Th. Greater economic potential is seen in placers, from which Th-minerals (namely, monazite) can be more readily recovered if demand for nuclear fuel arises. As it stands, no active production of thorium exists in the United States.
No definite data exists for thorium resources in Mexico, Central America, and the Caribbean, and likewise no current mining or processing operations (International Atomic Energy Agency, 2019).
In South America, with the exception of Brazil, no thorium has been extracted, but the total amount of extracted thorium is limited (International Atomic Energy Agency, 2019). Total resources are estimated at ∼1 Mt of thorium, though there are uncertainties and extraction is expected to be only possible as a by-product of mining activities. For example, in Araxa, where niobium ore is mined and ferroniobium is produced, thorium ends up in the slag and is disposed of at a rate of ∼1,000 t Th per year. Moreover, an extraction process for thorium from slag has not been developed, which would be needed before it could be used as a nuclear fuel.
3.2.3 Africa
With few exceptions, knowledge on thorium deposits in Africa is restricted to previous and active mining projects, mainly for uranium and for placers (South Africa), or to occurrences in other placers of probably very limited to nil economic relevance. Only a few countries, mainly South Africa, have resources in deposits readily available for extraction, such as the Steenkampskraal deposit, in case demand would develop (International Atomic Energy Agency, 2019). However, only a portion as low as 5% of the total resources, contained in placers, veins, carbonatites, and granites, is estimated to be recoverable as a by-product of other mining operations. In addition, thorium resources in addition to the estimated total occur in various countries.
3.2.4 Asia
Placer deposits in India contain thorium in the form of monazite (Dhana Raju et al, 2001). Manavalakurichi deposit in Tamil Nadu has been estimated to have ∼125,000 t of monazite containing ∼9,900 t of thorium. The state of Andhra Pradesh is considered the largest deposit of monazite in terms of tonnages, but at concentrations much lower than in Tamil Nadu (International Atomic Energy Agency, 2019). Brahmagiri in the state of Odisha is the location of a new commercially explored deposit, containing 610,000 t of monazite at a mean concentration of 0.06 wt% monazite. Monazite content in the Teri sands, which are red sands in inland regions of India, ranges from 0.01 to 3 wt% and Teri sands contain 130,000 t Th of India’s thorium resources (Balachandran, 2011). India’s thorium resources can be categorized state-wise as: Andhra Pradesh ∼36%, Tamil Nadu ∼20%, Odisha ∼17%, Kerala ∼14%, West Bengal ∼11%, and Bihar ∼2%. Total estimation of 846,477 t thorium in India, of which ∼493,000 t (∼58%) are identified resources, and the majority of which are in beach placers (∼68%), with an additional ∼353,000 t inferred and also primarily located in beach placers (International Atomic Energy Agency, 2019). Thorium recovery from the ash content of Indian coal has been identified as an additional potential source. Thorium has been commercialized as a nuclear fuel in India due to its resources far exceeding those of uranium in the country. Thorium extraction from monazite is performed by chemical processing, during which REE content is also recovered. Once extracted, thorium is converted into compounds used in nuclear reactors.
There is an estimation of thorium resources in the Asian Region of the former Union of Soviet Socialist Republics (∼1500000 t), China (>100,000 t), and Thailand (∼10,000 t); total resources for Asia are estimated to be more than 2500000 t of thorium (International Atomic Energy Agency, 2019). Quantification of the thorium resources in Asia is incomplete due to low commercial interest and information withholding due to strategic economic reasons. However, based on the developments in India, thorium can be exploited as a commercial commodity, especially when it becomes a by-product of REE production, which is in high demand for green technologies (Odimba and Santos, 2021).
3.2.5 Australia
The latest evaluations show resources containing 386,800 t Th (65%) in heavy mineral sands (placers), 125,000 t Th (21%) in vein-type deposits, 50,900 t Th in alkaline complexes, and 30,500 t Th in carbonatites. Monazite content varies regionally between 0.2 wt% in the fossil shoreline type deposits to 3.0 wt% or more in the fine-grained fossil offshore deposits (Geoscience Australia, 2012). Commercially active mining operations are recovering titanium and zirconium minerals (ilmenite, leucoxene, rutile, and zircon), while Th- and REE-bearing monazite is not recovered (i.e., accumulating in tailings).
4 Discussion
We are, at present in the third decade of the 21st century, waiting to see when factors will align to see global dissemination of the thorium nuclear fuel cycle. Jordan et al, (2015), a few years ago, summarized the main uncertainties that have slowed this factor alignment: (i) competing proposals for reactor designs; (ii) fuel configurations; and (iii) reprocessing options. As a result of these uncertainties, which will be played out by social, political, and economic interests, the certainty that we can end with is that the crustal abundance of thorium is a simpler question than the complex matter of usable availability.
Thorium is a future prospective nuclear fuel based on major considerations such as resource availability globally, economic evaluations that consider it as both a primary and secondary resource associated with other valuable mining products, environmental protection in terms of its relatively low short- and long-term carbon and environmental footprints, and its association with the mining of natural resources needed for green technologies such as REE, and the safeguarding of future generations due to the lower potential for proliferation of fissile materials (Kakodkar, 2006). Carbon emissions are threatening future generations as well and are a major driver in thinking about meeting future societal needs with an alternate energy source. At the same time, with society having negative opinions on nuclear power plants, especially as a consequence of recent events in Japan (Fukushima incident) and Ukraine (military threats), it is critical that future studies also consider the lesser-known health benefits resulting from the interlink between human lives and nuclear energy such as air pollution and birth weight (Shellenberger, 2017). Other studies have considered the health of mine workers in the pursuit of thorium resource exploitation; Lindsay et al, (2022) investigated the health hazards at the Steenkampskraal mine in the Western Cape Province in South Africa, where high natural thorium concentrations lead to high thoron activity concentrations. The study concludes that while thoron concentrations cannot be fully eliminated by mine ventilation, it should be possible to significantly reduce thoron progeny so that radiation should be an avoidable hazard with adequate engineered protections. On top of this, thorium natural resources are more abundant in Earth’s crust than uranium (Figure 4), so a mindset change towards thorium exploration is all but expected.
Nuclear energy has a vast scope to fulfill human needs, but there is a clear need to design nuclear power plants that are properly planned and that utilize high standards of safety precautions. The low to negligible extent of air pollution from well-operated nuclear power plants can support a carbon-free society. After the Fukushima accident in 2011, Japan decided to close nuclear power plants and replaced them to some extent with coal power plants, jeopardizing carbon emissions reduction goals. The country’s nuclear generation dropped to zero by September 2013 for almost 2 years, which jeopardized carbon emissions reduction goals—as they were replaced mostly with coal and natural gas power plants, as shown in Figure 7. The reactors have been being resumed slowly through the years. By September 2020, Japan had 33 operable nuclear reactors with a total installed net generating capacity of about 32 GW, down from 54 reactors with 47 GW of capacity before the Fukushima accident in 2011 [Ministry of Economy, 2023, Trade and Industry–Agency for Natural Resources and Energy, 2023]. The government’s stated aim is for nuclear power to provide 20%–22% of electricity by 2030 (World Nuclear Association, 2023). Looking back in history, the carbon emissions from the top twenty nations from 1960 are compared to the same group in 2018 (Figure 8) (Global Carbon Atlas, 2021). Cumulative carbon emissions have risen from 9.104 Gt CO2 in 1960 to 35.331 Mt CO2 in 2018 (Figure 9) (Global Carbon Atlas, 2021), an enrichment of 3.88 times which is coupled with a global population expected to reach 9 billion in the coming years (Lam, 2013). This has called and propelled the high-tech and fourth industrial revolution era that has accompanied marked lifestyle changes in society already in the last two decades. Such changes illustrate that despite the nuclear power industry seemingly being slow in development in recent years, progress can rapidly pick up and thorium can play a leading or important role (Hargraves, 2012). Moreover, Hargraves (2012) delves into the early days of nuclear power development to exemplify how the first version of a technology (the uranium fuel cycle with LWR developed in the first half of the 20th century) can be far from the technical ideality when economic and political factors take precedence. What has changed since then is the climate emergency (Santos and Bakhshoode, 2021).
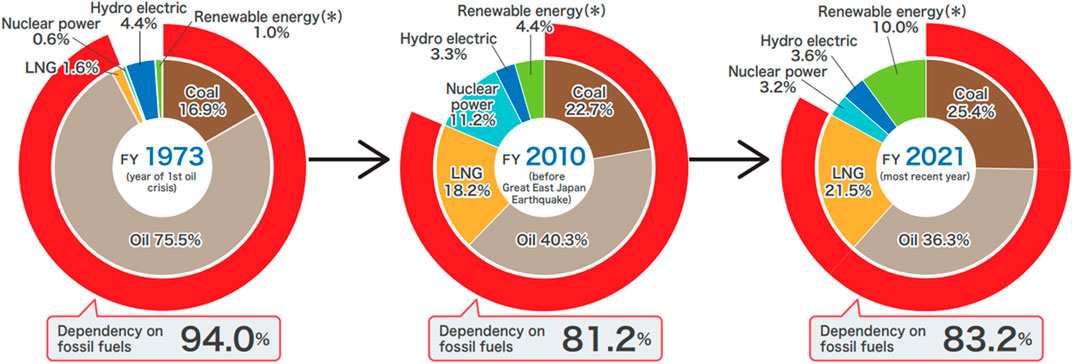
FIGURE 7. Sources of energy in Japan, 1973-2010-2021 (data adapted from Ministry of Economy, Trade and Industry–Agency for Natural Resources and Energy (2023)).
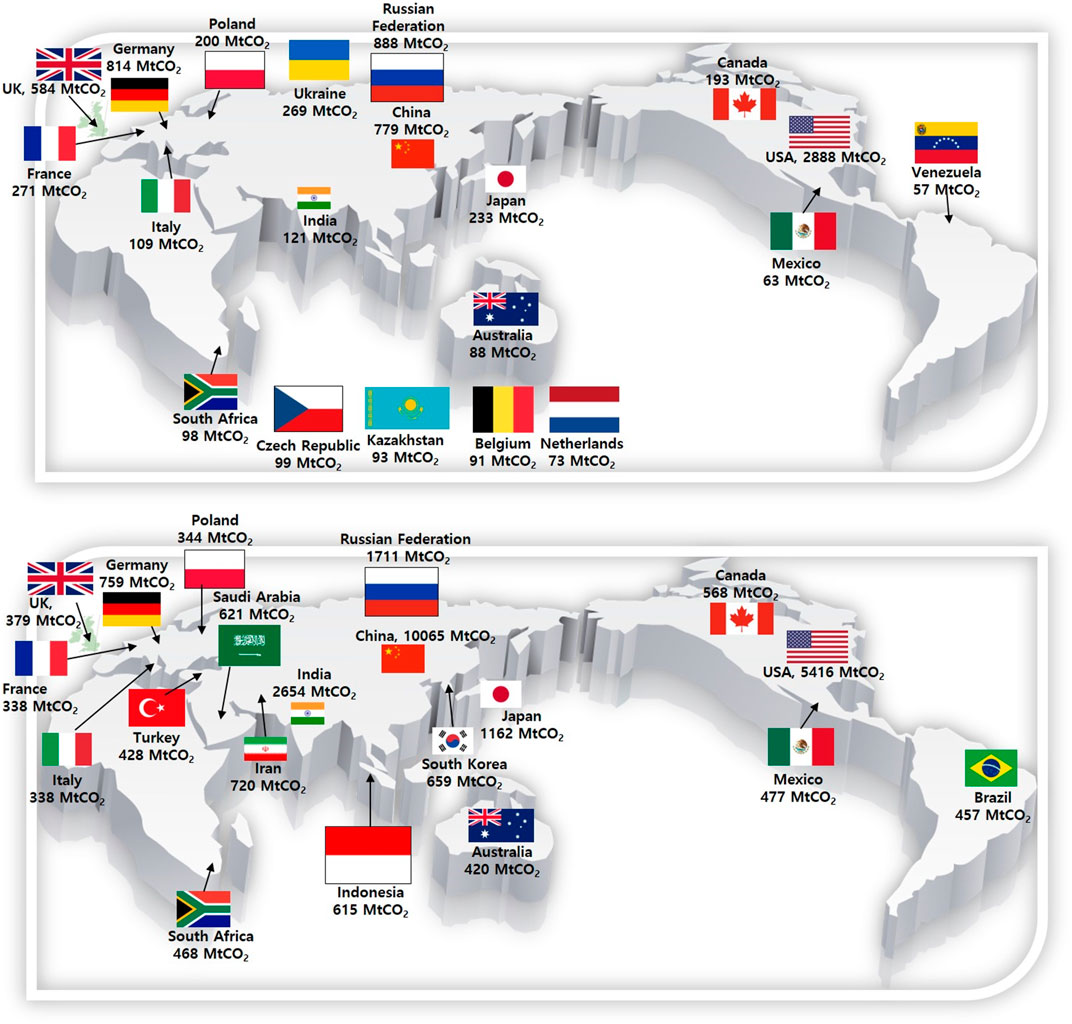
FIGURE 8. Comparison of carbon emissions of planet Earth in years 1960 (top) and 2018 (bottom) (data adapted from Global Carbon Atlas (2021)).
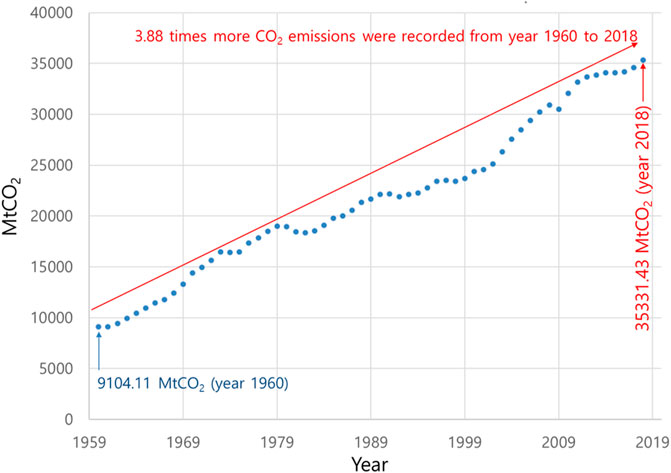
FIGURE 9. Total carbon emissions on planet Earth from 1960 to 2018 (data adapted from Global Carbon Atlas (2021)).
Most nations operating nuclear power plants have relied on LWR despite its several disadvantageous features: very short life span of the new fuel (1–3 years); generated plutonium waste; high pressure leading to hazard in case of failure and high risk for steam explosion (namely, the Chernobyl accident in 1986); high risk of fuel melting (namely, the Fukushima incident in 2011); high core radioactivity; and waste disposal problems (Dolan, 2017). On the other hand, Molten Salt Reactors (MSRs) have resurged in the search for constant advances in nuclear energy technologies. Its concept has been considered promising for further research and development by the Generation IV International Forum (GIF), as it offers significant improvements regarding environmental and safety concerns, by using liquid fuel, as well as economics, as shown in a model developed by Rykhlevskii (2019) in a simulation around reprocessing in an MSR. The thorium-uranium (Th-U) fuel cycle is an approach that can ensure the long-term supply of nuclear fuel, and that could be implemented in small modular MSR (Yu et al, 2019). Yu et al, (2019) found that in such applications the thorium energy contribution starts at 37.6% for the once-through mode of operation, increasing after several years to 47.4% once the batch reprocessing mode is implemented. At longer time scales of decades, the fuel transitions from low enriched uranium to 233U with online reprocessing mode, whereby the Th energy contribution can reach 89.1%.
In addition, International Atomic Energy Agency (2002) reports studies around more reactor systems and associated fuels, coolants/moderators. Some other reactors investigated on the thorium fuel cycles are PWR, CANDU, VVERT reactor, HTGR, WWER type reactors, FR, HWR, and ADS. Table 1 is a benchmark between other types of reactors, which shows the merit of MSR for the transmutation of minor-actinides (MA).
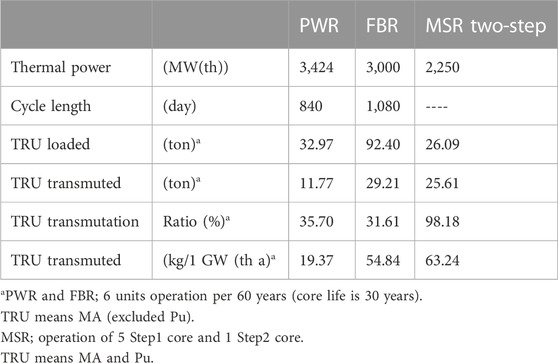
TABLE 1. Comparison of MA transmutation with other types of reactors (reprinted from International Atomic Energy Agency (2002)).
The lack of dedicated mines for thorium is somewhat worrying when compared to current commercial operations of uranium. However, thorium would be far from being the only natural resource from the periodic table requiring co-exploitation. Several examples can be obtained of valuable by-products of mining, simply by looking at the flowsheet of the Vale Base Metals (former Inco) operations in Ontario, Canada. While nickel is the main commodity of these operations, several additional valuable metals are co-extracted and refined, such as cobalt, platinum group metals (PGMs), selenium, and tellurium. Ault et al, (2015) thoroughly discuss the opportunities for integrated mining of thorium, which would be particularly beneficial as a means to close the resource gaps of what are currently terms ‘critical metals’ (or ‘critical minerals’). According to their study, the adoption and scale-up of the thorium nuclear fuel cycle should not result in thorium mines being constructed, because there is plenty of opportunities to recover thorium together with uranium and titanium mining (as well as smaller amounts recovered during tin, REE and iron mining), amounting to at least 100,000 t Th annually (mostly from titanium mines) from such active mines before new mines need to be brought online. Jordan et al, (2015), on the other hand, discuss that recovery costs will depend on whether thorium is a direct by-product of heavy mineral sand mining or a by-product of a by-product (i.e., tailings recovery), the former suggesting higher recovery costs. Considering the energy generation potential of this much thorium, it would take decades to require new resources. Ault et al, (2015) suggested that if all current nuclear energy generation switched from uranium to thorium, the by-product potentials would supply up to 12 times the amount of thorium required, assuming no reprocessing, and 250 times if reprocessing fuel cycle were implemented. It should be reminded, according to Ault et al, (2015), that the once-through thorium cycle still requires enriched uranium or plutonium as a seed starter, but a closed breeding fuel cycle does not, plus if uranium mining is reduced the titanium mining activities would more than sufficiently provided the needed thorium supply. Ault et al, (2016) go further in discussing the environmental benefits of not opening new mining activities and reducing the number of radionuclides ending up in mining tailings by switching from uranium to thorium fuel cycle. They also point out that while chemical processing for thorium recovery is needed, it is not a particularly challenging barrier in terms of technical and economic requirements.
Author contributions
RJ and H-SY conceptualized the study and wrote the first draft of the manuscript. LM and RS wrote sections of the manuscript. All authors contributed to the manuscript revision, and read and approved the submitted version. All authors listed have made a substantial, direct, and intellectual contribution to the work and approved it for publication.
Funding
This research was supported by the Basic Research Project (GP2022-010, 22-3212-1) of the Korea Institute of Geoscience and Mineral Resources (KIGAM), funded by the Ministry of Science and ICT of the Republic of Korea. LM and RS acknowledge funding from the Ontario Ministry of Agriculture, Food and Rural Affairs (OMAFRA) for the HQP scholarship of LM.
Conflict of interest
The authors declare that the research was conducted in the absence of any commercial or financial relationships that could be construed as a potential conflict of interest.
Publisher’s note
All claims expressed in this article are solely those of the authors and do not necessarily represent those of their affiliated organizations, or those of the publisher, the editors and the reviewers. Any product that may be evaluated in this article, or claim that may be made by its manufacturer, is not guaranteed or endorsed by the publisher.
References
Alexander, J. S., Edwards, G. W. R., Bromley, B. P., and Lane, K. (2020). The effects of typical thorium impurities on thorium-based nuclear fuels. CNL Nucl. Rev. 9 (1), 27–38. doi:10.12943/CNR.2018.00002
Ashley, A. F., Lindley, B. A., Parks, G. T., Nuttal, W. J., Gregg, R., Hesketh, K. W., et al. (2014). Fuel cycle modelling of open cycle thorium-fuelled nuclear energy systems. Ann. Nucl. Energy 69, 314–330. doi:10.1016/j.anucene.2014.01.042
Ault, T., Krahn, S., and Croff, A. (2015). Assessment of the potential of by-product recovery of thorium to satisfy demands of a future thorium fuel cycle. Nucl. Technol. 189 (2), 152–162. doi:10.13182/NT14-19
Ault, T., Krahn, S., Worrall, A., and Croff, A. (2018). Applications for thorium in multistage fuel cycles with heavy water reactors. Nucl. Technol. 204 (1), 41–58. doi:10.1080/00295450.2018.1468702
Ault, T., Van Gosen, B., Krahn, S., and Croff, A. (2016). Natural thorium resources and recovery: Options and impacts. Nucl. Technol. 194 (2), 136–151. doi:10.13182/NT15-83
Balachandran, K. (2011). Thorium resources potential of red sand (Teri) placers of India. Vienna, Austria: IAEA Tech. Mtg on World Thorium Resources.
Banerjee, S., Sinha, R. K., and Kailas, S. (2011). Thorium utilization for sustainable supply of nuclear energy. J. Phys. Conf. Ser. 312, 062002. doi:10.1088/1742-6596/312/6/062002
Bell, J. S., Chan, P. K., and Prudil, A. A. (2019). A thoria and thorium uranium dioxide nuclear fuel performance model prototype and knowledge gap assessment. ASME J Nucl. Rad Sci 5 (1), 011005. doi:10.1115/1.4039778
British Petroleum, (2019). BP statistical review of world energy 2019. 68. London, United Kingdom: BP plc.
British Petroleum, (2022). BP statistical review of world energy 2022. 71. London, United Kingdom: BP plc.
Chadirji-Martinez, K., Grosvenor, A. P., Crawford, A., Chernikov, R., Heredia, E., Feng, R., et al. (2022). Thorium speciation in synthetic anhydrite: Implications for remediation and recovery of thorium from rare-Earth mine tailings. Hydrometallurgy 214, 105965. doi:10.1016/j.hydromet.2022.105965
Clark, D. L., Keogh, D. W., and NeuRunde, M. P. W. (2000). “Thorium and thorium compounds,” in Kirk-othmer encyclopedia of chemical technology (New Jersey, United States: John Wiley & Sons, Inc).
Demirbaş, A. (2005). Options and trends of thorium fuel utilization. Energy sources. 27 (7), 597–603. doi:10.1080/00908310490448596
Dhana Raju, R., Azam Ali, M., and Krishnan, S. S. (2001). Beach and inland heavy mineral sand deposits of India, special issue, exploration and research for atomic minerals 13. Hyderabad, Telangana: Atomic Minerals Directorate for Exploration and Research, 159.
Dittmar, M. (2018). The future of nuclear energy: Facts and fiction chapter IV: Energy from breeder reactors and from fusion? Phys. Soc. arXiv. doi:10.48550/arXiv.0911.2628
Dolan, T. J. (2017). “Molten salt reactors and thorium energy,” in Molten salt reactors and thorium energy (Sawston, United Kingdom: Woodhead Publishing).
Du Toit, M. H., and Cilliers, A. C. (2014). Preliminary economic evaluation of thorium-based fuels in PWRs. Nucl. Technol. 187 (3), 260–269. doi:10.13182/NT13-134
Ember, (2022). European electricity review 2022. Available online: https://ember-climate.org/app/uploads/2022/02/Report-EER.pdf (accessed 04 15, 2023).
Ember, (2023). Yearly electricity data. Available online: https://ember-climate.org/data-catalogue/yearly-electricity-data/ (accessed 04 15, 2023).
Ganesan, S. (2016). “Nuclear data development related to the Th–U fuel cycle in India,” in Thorium energy for the world. Editors J. .P. Revol, M. Bourquin, Y. Kadi, E. Lillestol, J. C. de Mestral, and K. Samec (Berlin, Germany: Springer).
Geoscience Australia, (2012). Australian’s identified mineral resources, mines and processing centres. Symonston, Australia: Geoscience Australia.
Global Carbon Atlas, (2021). Global carbon Atlas. Available online: http://www.globalcarbonatlas.org/ (accessed 12 26, 2022).
Gunsing, F., et al. (2016). “Nuclear data for the thorium fuel cycle and the transmutation of nuclear waste,” in Thorium energy for the world. Editors J. .P. Revol, M. Bourquin, Y. Kadi, E. Lillestol, J. .C. de Mestral, and K. Samec (Berlin, Germany: Springer).
György, H., and Czifrus, S. (2017). Investigation on the potential use of thorium as fuel for the Sodium-cooled Fast Reactor. Ann. Nucl. Energy 103, 238–250. doi:10.1016/j.anucene.2017.01.030
Hania, P. R., and Klaassen, F. C. (2012). Thorium oxide fuel. Compr. Nucl. Mater., 87–108. doi:10.1016/b978-0-08-056033-5.00052-5
Haque, F., Santos, R. M., and Chiang, Y. W. (2021). Urban farming with enhanced rock weathering as a prospective climate stabilization wedge. Environ. Sci. Technol. 55 (20), 13575–13578. doi:10.1021/acs.est.1c04111
Hargraves, R. (2012). Thorium energy cheaper than coal. California, United States: CreateSpace Independent Publishing Platform, 482.
Ho, M., Obbard, E., Burr, P. A., and Yeoh, G. (2019). A review on the development of nuclear power reactors. Energy Procedia 160, 459–466. doi:10.1016/j.egypro.2019.02.193
Hummel, W. (2005). Solubility equilibria and geochemical modeling in the field of radioactive waste disposal. Pure Appl. Chem. 77 (3), 631–641. doi:10.1351/pac200577030631
International Atomic Energy Agency, (2023). Power reactor information system - in operation & suspended operation. Available Online: https://pris.iaea.org/PRIS/WorldStatistics/OperationalReactorsByCountry.aspx (accessed 05 01, 2023).
International Atomic Energy Agency, (2012). Role of thorium to supplement fuel cycles of future nuclear energy systems. IAEA Nuclear Energy Series No. NF-T-2.4. Available online: https://www.iaea.org/publications/8703/role-of-thorium-to-supplement-fuel-cycles-of-future-nuclear-energy-systems (accessed 12 26, 2022).
International Atomic Energy Agency, (2005). Thorium fuel cycle-potential benefits and challenges. IAEA-TECDOC-1450. Available online: https://www.iaea.org/publications/7192/thorium-fuel-cycle-potential-benefits-and-challenges (accessed 12 26, 2022).
International Atomic Energy Agency, (2002). Thorium fuel utilization: Options and trends. IAEA-TECDOC-1319 Available online: https://www.iaea.org/publications/6395/thorium-fuel-utilization-options-and-trends (accessed 12 26, 2022).
International Atomic Energy Agency, (2019). World thorium occurrences, deposits and resources. IAEA-TECDOC-1877. Available online: https://www-pub.iaea.org/MTCD/Publications/PDF/TE-1877web.pdf (accessed 12 25, 2022).
International Energy Agency (2011). World energy outlook 2011 - energy for all. Available online: https://www.iea.org/reports/energy-for-all (accessed 12 26, 2022).
Jiyang, Y., Kan, W., Songbo, Y., Baoshan, J., Shifei, S., Gong, S., et al. (2004). Thorium fuel cycle of a thorium-based advanced nuclear energy system. Prog. Nucl. Energy 45 (1), 71–83. doi:10.1016/j.pnueene.2004.07.004
Jordan, B. W., Eggert, R. G., Dixon, B. W., and Carlsen, B. W. (2015). Thorium: Crustal abundance, joint production, and economic availability. Resour. Policy 44, 81–93. doi:10.1016/j.resourpol.2015.02.002
Kakodkar, A., and Sinha, R. K. (2006). “The twin challenges of abundant nuclear energy supply and proliferation risk reduction – a view,” in 50th IAEA General Conference, Vienna, Austria, 18 September 2006.
Kazimi, M. S. (2003). Thorium fuel for nuclear energy. Am. Sci. 91 (5), 408–415. doi:10.1511/2003.32.884
Khalidy, R., and Santos, R. M. (2021). Assessment of geochemical modeling applications and research hot spots – A year in review. Environ. Geochem. Health 43 (9), 3351–3374. doi:10.1007/s10653-021-00862-w
Lainetti, P. E. O. (2017). “Prospective thorium fuels for future nuclear energy generation,” in INAC 2017: International Nuclear Atlantic Conference, Minas Gerais, Brazil, October 2017.
Lenzen, M. (2008). Life cycle energy and greenhouse gas emissions of nuclear energy: A review. Energy Convers. Manag. 49 (8), 2178–2199. doi:10.1016/j.enconman.2008.01.033
Lindsay, R., Mngonyama, S., Molahlehi, P., Ngwadla, X. E., and Ramonnye, G. J. (2022). Pilot study of thoron concentration in an underground thorium mine. Health Phys. 123 (4), 315–321. doi:10.1097/HP.0000000000001598
Lofts, S., Fevrier, L., Horemans, N., Gilbin, R., Bruggeman, C., and Vandenhove, H. (2015). Assessment of co-contaminant effects on uranium and thorium speciation in freshwater using geochemical modelling. J. Environ. Radioact. 149, 99–109. doi:10.1016/j.jenvrad.2015.07.011
Loiseaux, J.-M., David, S., Heuer, D., and Nuttin, A. (2002). Thorium fuel, an interesting option for future nuclear energy. Comptes Rendus Phys. 3 (7), 1023–1034. doi:10.1016/s1631-0705(02)01373-7
Lung, M., and Gremm, O. (1998). Perspectives of the thorium fuel cycle. Nucl. Eng. Des. 180 (2), 133–146. doi:10.1016/S0029-5493(97)00296-3
Mendoza España, A. D., and Bromley, B. P. (2019). A Canadian perspective of the economic issues associated with deploying thorium-based fuel cycles and breeding in heavy-water reactors. CNL Nucl. Rev. 8 (2), 109–130. doi:10.12943/CNR.2017.00021
Ministry of Economy (2023). Trade and industry - agency for natural resources and energy. Japan’s Energy - 10 questions for understanding the current energy situation. Available online: https://www.enecho.meti.go.jp/en/category/brochures/pdf/japan_energy_2022.pdf (accessed 04 16, 2023).
OECD-NEA (2010). Uranium 2009: Resources, Production and Demand. Paris: OECD-NEA, 37–41. Available online: https://www.oecd-nea.org/jcms/pl_14566/uranium-2009-resources-production-and-demand (accessed 2023-05-08).
Office of Nuclear Energy, (2021). 3 reasons why nuclear is clean and sustainable. Available online: https://www.energy.gov/ne/articles/3-reasons-why-nuclear-clean-and-sustainable (accessed 12 25, 2022).
Odimba, N., and Santos, R. M. (2021). “Application of geochemical modeling in rare earth elements leaching of coal combustion and secondary residues,” in Clean coal technologies. Editors R. K. Jyothi, and P. K. Parhi (Springer Nature), 605–616.
Ramanna, R., and Lee, S. M. (1986). The thorium cycle for fast breeder reactors. Pramana - J. Phys. 27 (1-2), 129–137. doi:10.1007/BF02846334
Rykhlevskii, A., Bae, J. W., and Huff, K. D. (2019). Modeling and simulation of online reprocessing in the thorium-fueled molten salt breeder reactor. Ann. Nucl. Energy 128, 366–379. doi:10.1016/j.anucene.2019.01.030
Şahin, S., Şahin, H. M., Alkan, M., and Yıldız, K. (2004). An assessment of thorium and spent LWR-fuel utilization potential in CANDU reactors. Energy Convers. Manag. 45 (7–8), 1067–1085. doi:10.1016/j.enconman.2003.08.002
Salehuddin, A. H. J. M., Ismail, A. F., Bahri, C. N. A. C. Z., and Aziman, E. S. (2019). Economic analysis of thorium extraction from monazite. Nucl. Eng. Technol. 51, 631–640. doi:10.1016/j.net.2018.11.005
Santos, R. M., and Bakhshoodeh, R. (2021). Climate change/global warming/climate emergency versus general climate research: Comparative bibliometric trends of publications. Heliyon 7 (11), e08219. doi:10.1016/j.heliyon.2021.e08219
Schaffer, M. B. (2013). Abundant thorium as an alternative nuclear fuel. Energy Policy 60, 4–12. doi:10.1016/J.Enpol.2013.04.062
Serfontein, D. E., and Mulder, E. J. (2014). Thorium-based fuel cycles: Reassessment of fuel economics and proliferation risk. Nucl. Eng. Des. 271, 106–113. doi:10.1016/j.nucengdes.2013.11.018
Shellenberger, M. (2017). Nuclear power: Unexpected health benefits. Nat. Energy 2, 17058. doi:10.1038/Nenergy.2017.58
Siddique, M., Iqbal, A., Rahman, A. U., Azam, S., Zada, Z., and Talat, N. (2021). Mechanical and thermodynamic stability, structural, electronics and magnetic properties of new ternary thorium-phosphide silicides ThSixP1-x: First-principles investigation and prospects for clean nuclear energy applications. Nucl. Eng. Technol. 53, 592–602. doi:10.1016/j.net.2020.07.019
The Economist, (2022). France’s nuclear reactors will not work as normal any time soon. Available online: https://www.economist.com/europe/2022/10/13/frances-nuclear-reactors-will-not-work-as-normal-any-time-soon (accessed 05 01, 2023).
Van Gosen, B. S., Gillerman, V. S., and Armbrustmacher, T. J. (2009). Thorium deposits of the United States–Energy resources for the future? U.S. Geol. Surv. Circ. 1336, 21.
Warner, E. S., and Heath, G. A. (2012). Life cycle greenhouse gas emissions of nuclear electricity generation: Systematic review and harmonization. J. Industrial Ecol. 16 (1), S73–S92. doi:10.1111/J.1530-9290.2012.00472.X
World Nuclear Association, (2022a). Carbon dioxide emissions from electricity. Available online: https://www.world-nuclear.org/information-library/energy-and-the-environment/carbon-dioxide-emissions-from-electricity.aspx (accessed 04 15, 2023).
World Nuclear Association, (2023). Nuclear power in Japan. Available online: https://world-nuclear.org/information-library/country-profiles/countries-g-n/japan-nuclear-power.aspx (accessed 04 16, 2023).
World Nuclear Association, (2022c). Supply of uranium. Available online: https://world-nuclear.org/information-library/nuclear-fuel-cycle/uranium-resources/supply-of-uranium.aspx (accessed 04 15, 2023).
World Nuclear Association, (2020b). The nuclear fuel report. Available online: https://world-nuclear.org/getmedia/9a2f9405-1135-407a-85c8-480e2365bee7/nuclear-fuel-report-2021-expanded-summary.pdf.aspx (accessed 04 15, 2023).
World Nuclear Association, (2020a). Thorium. Available online: https://world-nuclear.org/information-library/current-and-future-generation/thorium.aspx (accessed 04 15, 2023).
World Nuclear Association, (2022b). World nuclear performance report 2022/003. Available online: https://www.world-nuclear.org/information-library/energy-and-the-environment/carbon-dioxide-emissions-from-electricity.aspx (accessed 04 15, 2023).
Xiong, X. H., Tao, Y., Yu, Z. W., Yang, L. X., Sun, L. J., Fan, Y. L., et al. (2020). Selective extraction of thorium from uranium and rare Earth elements using sulfonated covalent organic framework and its membrane derivate. Chem. Eng. J. 384, 123240. doi:10.1016/j.cej.2019.123240
Keywords: nuclear fuel, global resources, monazite, molten salt reactor, lifecycle emissions
Citation: Jyothi RK, De Melo LGTC, Santos RM and Yoon H-S (2023) An overview of thorium as a prospective natural resource for future energy. Front. Energy Res. 11:1132611. doi: 10.3389/fenrg.2023.1132611
Received: 27 December 2022; Accepted: 04 May 2023;
Published: 15 May 2023.
Edited by:
Srinivasan Ganesan, Ex-Bhabha Atomic Research Centre, IndiaReviewed by:
Devan K., Indira Gandhi Centre for Atomic Research (IGCAR), IndiaViswanathan Jagannathan, Bhabha Atomic Research Centre (BARC), India
Umasankari Kannan, Bhabha Atomic Research Centre (BARC), India
Copyright © 2023 Jyothi, De Melo, Santos and Yoon. This is an open-access article distributed under the terms of the Creative Commons Attribution License (CC BY). The use, distribution or reproduction in other forums is permitted, provided the original author(s) and the copyright owner(s) are credited and that the original publication in this journal is cited, in accordance with accepted academic practice. No use, distribution or reproduction is permitted which does not comply with these terms.
*Correspondence: Rajesh Kumar Jyothi, cmt1bWFycGhkQGtpZ2FtLnJlLmty; Ho-Sung Yoon, aHN5b29uQGtpZ2FtLnJlLmty
†These authors have contributed equally to this work and share first authorship