- 1State Key Laboratory of Petroleum Pollution Control, CNPC Research Institute of Safety and Environmental Technology, Beijing, China
- 2China University of Petroleum Beijing, Beijing, China
Due to the high energy density, large potential reserves and only release CO2 and water after combustion, natural gas hydrate (NGH) is considered as the most likely new clean energy source to replace traditional fossil energy (crude oil, natural gas, etc.). However, unlike the exploitation of traditional fossil energy, the essence of natural gas hydrate exploitation is to induce the production of methane by artificially decompose the natural gas hydrate and to simultaneously collect the generated methane. Because of the uncontrollable decomposition, the methane percolation and the gas collection efficiency, methane emission is inevitably occurred during natural gas hydrate exploitation, which could significantly affect the environmental friendliness of natural gas hydrate. In this review, the methane emission detection was divided into three interfaces: Seafloor and sediment, seawater, atmosphere. Meanwhile, according the summary and analysis of existing methane emission detection technologies and devices, it was concluded that the existing detection technologies can identify and quantify the methane emission and amount in the three interfaces, although the accuracy is different. For natural gas hydrate exploitation, quantifying the environmental impact of methane emission and predicting the diffusion path of methane, especially the methane diffusion in strata and seawater, should be the focus of subsequent research.
1 Introduction
As the demand of energy kept increasing and the environmental pollution became more serious, fossil energy, including oil, coal, and natural gas were unable to meet the requirements of energy amount and environment protection. Therefore, the development of clean-alternative energy attracted much attention in recent years (Khare et al., 2016; Olabi, 2017; Liu L. et al., 2019). However, common renewable energies, including wind energy, solar energy, biomass energy, nuclear energy and ocean energy were constrained by geographic conditions, infrastructure or politician issues (Twidell and Weir, 2015; Bhattacharya et al., 2016; Quaschning, 2016). More importantly, the capacity and growth rate of renewable energies were much lower than the increase in energy consumption according to the report published by International Energy Agency (IEA) (IEA, 2019).
Natural gas hydrate (NGH) is an ice-like crystalline compound formed by natural gas (mostly methane) molecules and water molecules (Pearson et al., 1983; Li et al., 2012a; Zhao et al., 2015; Khan et al., 2016). Since the crystal structure and unit cell of NGH were determined and published by Claussen and Von Stachelberg in 1949 (Claussen, 1951a; Claussen, 1951b), the research on the physical and chemical properties of NGH continues. Commonly, the empirical formula of NGH can be expressed as (CH4)8(H2O)46 (Dharmawardhana et al., 1980; Stackelberg, 1949; Clennell et al., 1999; Takeyaa et al., 2006; Li et al., 2012b), the crystal structure of NGH was considered to be in three types (sI, sII and sH) (Tse, 1990; Matsumoto et al., 2000; Kumar et al., 2008). Since the naturally-produced NGH was detected in the permafrost of northern Siberia, Messoyakha oilfield in 1965 (Hitchon, 1974; Makogon and Omelchenko, 2013), significant amount of NGH reservoirs have been discovered and exploited worldwide (Dickens et al., 1997; Collett, 1999; Shukla et al., 2019). Although NGH is considered to be an efficient and clean energy, the methane escaping into the seawater and air during production can seriously impact on the environment (Yang L. et al., 2019). Due to its molecule structure, methane is one of the most potent greenhouse gases (GHGs) (Holmes et al., 2017; Krapivina et al., 2017; Tutak and Brodny, 2017). Therefore, only by preventing or reducing the methane emissions during the production of NGH can NGH truly become a clean energy.
Currently, many reviews were conducted by different researchers and groups over the world, including the investigation of NGH’s fundamental properties (e.g. structure, composition, formation and decomposition) (Kvenvolden, 1995; Bavoh et al., 2019; Lijith et al., 2019; Yang M. et al., 2019), the exploration and production of NGH reservoirs (Lee et al., 2011; Li et al., 2016; Acharya et al., 2019; Ke et al., 2019; Li et al., 2019), the utilization and potential of NGH (Kvenvolden and Lorenson, 2001; Koh and Sloan, 2007; Demirbas et al., 2016). Meanwhile, as the development of NGH production pilot worldwide, many researchers have studied the environmental impact of NGH exploration and production (Liu S. et al., 2019; Riley et al., 2018; Stanković et al., 2017; Kvenvolden and Barnard, 1982; Ye et al., 2018. However, few reviews on the study of methane emission detection during the exploitation of NGH reservoirs. Therefore, in this review, we summarized and strived to review holistically the studies and progresses on methane emission detection during NGH production process from the following aspects, including current issues, seafloor and sediment detection, seawater detection, atmosphere detection, and challenges. We hope to provide more information of how methane emitted from NGH and how to detection the methane emission during NGH production. Moreover, we also put forward some suggestions on the establishment of methane emission monitoring system and on the methods for methane emission reduction during NGH production.
2 Existing issues
2.1 Environmental risks during NGH production
Environmental impact is a potential risk which cannot be ignored during the production of NGH. Firstly, the dissociation of NGH could lead to significant drilling risks, resulting in gas leakage, blowout, collapse, wellbore instability or failure (Islam, 1991). Since the decomposition of hydrate is endothermic process and its formation is exothermic process, the temperature gradient in the wellbore and formation could change significantly during the production process, which could possibly lead to stability reduction. Meanwhile, the CH4 concentration in pore water increases as the NGH decomposition process proceeds, which led to an increase in sulphur content and increase the equipment corrosion risk (Song et al., 2016). Secondly, the dissociation of NGH deposits could trigger large underwater landslides on continental margins, which could destroy offshore mining equipment, endanger the lives of operators, and pose a hazard to coastal areas (Locat and Lee, 2002; Collett et al., 2014). Besides, the uncontrollable dissociation of NGH could cause methane emission and significantly impact on the atmospheric environment. The atmospheric record of ice cores during the Pleistocene suggests that the increase of CH4 in the atmosphere is mainly due to the release of NGH by dissociation (Maslin et al., 2004; Sun et al., 2019).
In essence, the NGH production was to artificially induce the decomposition of the NGH (e.g., hot water injection, pressure reduction, chemical injection, direct grinding, etc.), and then collect the methane generated during decomposition process. Therefore, the stability of NGH layers would be reduced while the production proceeds (Maslin et al., 2010). Meanwhile, since the hydrate layer and sediment were integrated in structure at NGH reservoirs, the decrease of stability of hydrate layer will lead to the decrease of structural strength of sediment layer, resulting in the deformation of seafloor and the occurrence of major disasters such as submarine landslides, methane escape, earthquakes and tsunamis.
2.2 Limitation of current monitoring system
With the development of research and the progress on technologies/equipment, many countries engaged in NGH research and exploration had carried out field test production, including Canada, United States, Japan and China (Li et al., 2018) (Table 1).
In these field tests, environmental monitoring had been attached great importance, and monitoring systems suitable for each test`s production characteristics have been established (Figure 1). Atmospheric component monitoring, wellbore leakage monitoring, underwater sensors, underwater/submarine robots, non-contact detection and other technologies were applied and validated in these field tests.
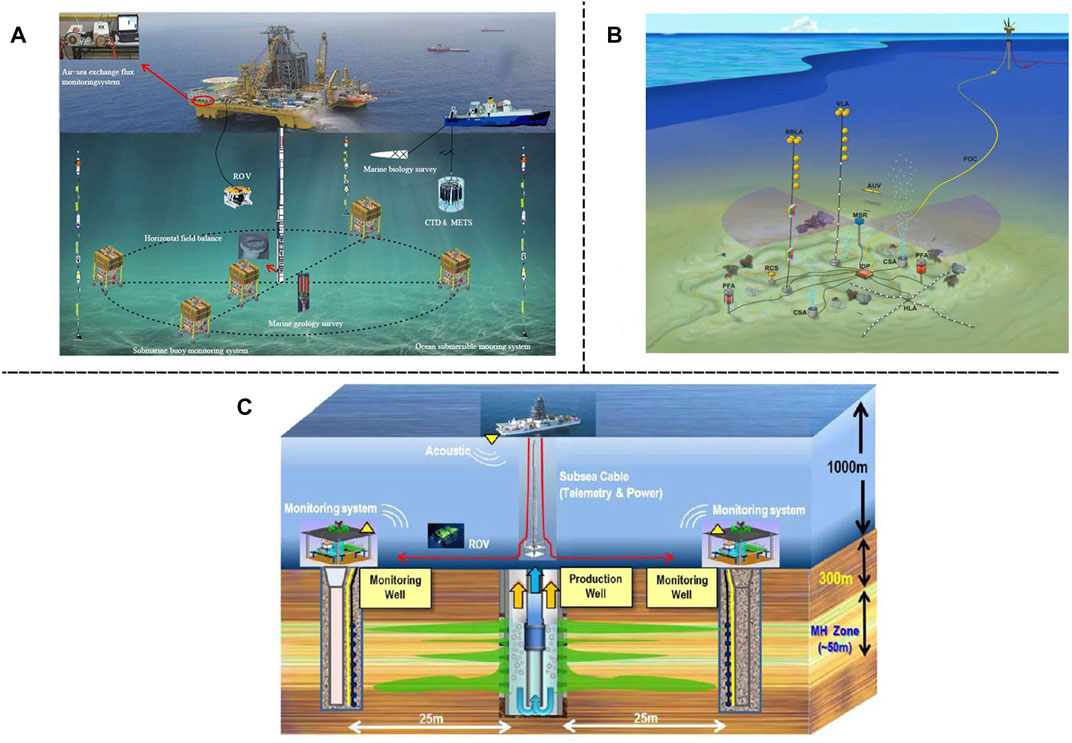
FIGURE 1. Current monitoring systems for NGH field test. Image (A) “Four-in-one” environmental monitoring system employed during South China Sea NGH production field test in 2017 (Li et al., 2018). Image (B) illustration of the seafloor observatory at the Woolsey Mound (Mississippi Canyon Lease Block 118, MC118) in 2012 (Macelloni et al., 2012). Image (C) production and monitoring systems at Nankai Trough in 2013 (Chee et al., 2014).
During 2011 to 2017, the China Geological Survey (CGS) launched a filed trial of NGH production at Shenhu area, South China Sea. In this field test, new submarine technologies, such as swath bathymetry, three-dimensional seismic data, side-scan sonar, and AUVs (He et al., 2018), as well as numerical simulation tools (Shi et al., 2019), were utilized for the establishment of a “four-in-one” (atmosphere, seawater column, seafloor and underground) comprehensive environmental monitoring system Figure 1A (Li et al., 2018; ye et al., 2018).
In 2008, the Gulf of Mexico Hydrates Research Consortium designed and implemented the Monitoring Station/Seafloor Observatory (MS-SFO) (Majumdar and Cook, 2018; Moore et al., 2022), which was a seafloor observatory network with the purpose of monitoring gas hydrate-bearing sediment dynamics the Woolsey Mound in MC118. The seafloor observatory consists of seismic-acoustic receiving arrays, geochemical arrays in bottom water column and upper sediments, micro-biologic sensors, and s series of arrays: Horizontal Line Array (HLA), Vertical Line Array (VLA), Chimney Sampler Array (CSA), Pore-fluid Array (PFA), and Benthic Boundary Line Array (BBLA), etc.
Japan`s Research Consortium for Methane Hydrate Resources conducted a series of studies to develop environmental monitoring technologies for the NGH production field test since 2001. Besides, these technologies, which mainly included sensors (e.g., methane sensors, seafloor deformation sensors, biosensors), integrated environmental monitoring system, and auxiliary devices (e.g., power system, electric cables, etc.), were applied and verified in the world’s first depressurization method to exploit offshore NGH reservoir in 2013, Nankai trough, Japan (Fujii et al., 2015; Yamamoto, 2015).
In NGH production field tests to date, rigorous monitoring systems had been deployed and no large-scale methane leakage had been reported. However, due to the greater hazards of geological disasters, the focus of monitoring systems was on the changes of sediment stability and formation structure. The methane leakage detection and monitoring were realized by placing sensors in seawater at different depths. This method shown the advantages of low cost, accurate data, short response time and strong reliability, but it also shown a small monitoring range and significantly affected by seawater flow. Therefore, continuously developments on new monitoring technologies for different monitoring scenarios were still necessary, to achieve methane emission detection over a wider area.
3 Methane emission detection technologies
In the process of NGH production, methane exists in three layers: the seafloor and sedimentary layer, seawater, and atmosphere. Since the occurrence state of methane, media, type of disturbance, temperature and pressure conditions in these three scenarios are significantly different, the methane emissions detection techniques need to be selected according to the differences in utilization scenarios.
3.1 Seafloor and sediment
In seafloor and sediments, free gaseous methane exists in porous media composed of argillaceous silt, the contact detection methods are not feasible. Meanwhile, since the muddy silty sand layer existence in deep water, and compared with onshore strata, the muddy silty layer has the characteristics of weak cementation and low strength, which makes conventional geophysical detection methods unable to be applied. Due to their characteristics, sound wave detection, seismic reflection, underwater optical observation and other technologies shown the ability to complete the detection of subsea and sedimentary methane emissions (Table 2).
3.1.1 Non-explosive seismic reflection
Seismic reflection technology had developed as a method to image subsurface structures, especially in the oil and gas exploitation industries. It is possible to identify sedimentary structures from the reflection configuration and faults by offsetting the reflections on the seismic reflection profiles (Tsuru et al., 2018). Commonly, explosives were utilized as the energy sources to generate pulse waves with high sound pressure. However, the high sound pressure generated by explosive (more than 160 dB in the frequency range below 400 Hz) could cause serious damage to aquatic mammals and fish and threaten their lives (Hatakeyama et al., 1997). In this situation, non-explosive energy sources, including underwater speaker (UWS), low-power air gun, electrical transmitter and mechanical shock were utilized on marine geological prospecting. These non-explosive sources generate non-pulse waves (electrical transmitter could generate both pulse and non-pulse waves) with low sound pressure over a certain period of time (lower than 130 dB in the frequency range of 100–1,000 Hz) (IAGC, 2002), and it is possible to conduct seismic surveys with a relatively low environmental impact on marine ecosystems.
Since offshore NGH layers are usually shallowly buried and the overlying layers are not as dense as onshore strata, high-resolution structural profiles could be obtained using non-pulsed waves, and the differences in wave velocities in different regions can be obtained to determine the accumulation of gaseous methane. These studies had been confirmed in a study by Japanese researchers (Tsuru et al., 2019). Non-explosive source detection could be used as a safe and low environmental impact detection method to continuously and real-time detect the accumulation and distribution of gaseous methane in seafloor and sedimentary layers.
3.1.2 Underwater optical observation
Unlike onshore reservoirs, offshore NGH reservoirs are covered with seawater, which is transparent and makes direct observation with optical equipment possible. The keys to underwater optical observation is optical receiver. Since the light propagation is more complex in a seawater environment, the light received by the optical receiver has three parts: the imaging beam reflected from the target, absorbed by the water medium and lost by scattering; the backscattered light between the light source and the target affects the specific illumination of the image; the forward scattered light formed by the small scattering angle between the target and the receiver, which can directly affect the detail resolution of the target. Therefore, the research focus of underwater optical observation is to reduce the influence of strong scattering effect and fast absorption power attenuation characteristics of water medium on underwater communication, imaging and target detection.
At present, several underwater optical observation technologies had been applied in practice and achieved good working results: 1) Synchronous scanning imaging, is the synchronization of the scanning beam (continuous laser) and the receiving line of sight, using the principle that the backscattered light intensity of water decreases rapidly relative to the central axis. This technique uses collimating beam point scanning and narrow field of view tracking receiving of highly sensitive detectors based on photomultiplier tubes (Liu, 1999). This technique could effectively improve the signal-to-noise ratio and the action range of imaging. 2) Range gating technique, is to use pulsed laser and gating camera to separate the scattered light at different distances from the reflected light of the target, in order to make the radiation pulse reflected from the observed target reach the camera and image within the same time when the camera gating works (Busck and Heiselberg, 2004; Tu et al., 2021). This method is very useful for solving the backscattering problem caused by suspended particles in seawater. 3) Polarization imaging technique, is to improve the resolution of imaging by using the polarization characteristics of reflected light and backscattered light of objects (Boer et al., 1998; Kong et al., 2020). This technique could improve the specific illumination and resolution by adjusting the ratio of reflected and scattered light energy. 4) Underwater laser three-dimensional imaging technique, is to measure the round-trip time between the transmitter and the target, and recover the distance image of the original target (Schulein and Javidi, 2010).
Underwater optical observation technologies could rapidly, continuously and intuitively observe the upper surface of NGH layer and sedimentary layer, and provide early warning for gas leakage and fracture production, but it cannot quantify the amount of gas escape and obtain gas components.
3.1.3 Sub-bottom profiler
Sub-bottom Profiler, also known as shallow seismic profiler, is to detect the profile structure of shallow bottom strata by transmitting sound waves and receiving reflected sound waves. It is an improved device based on ultra-broadband submarine profiler, which displays the strata at the bottom of oceans, rivers and lakes. Combined with geological interpretation, it can detect the geological structure below the water bottom. The instrument has high performance in formation resolution and formation penetration depth, and can choose any combination of sweep signals to design and adjust working parameters in real time, as well as to measure bedrock depth and thickness in offshore oilfield drilling (Yang et al., 2021). Therefore, it is a widely used instrument in Marine geological survey, geophysical exploration and ocean engineering, ocean observation, seabed resources exploration and development, waterway harbor engineering, seabed pipeline laying (Li et al., 2021).
Due to its mechanism and characteristics, although the sub-bottom profiler had been utilized to detect the structure of sedimentary layer and cannot directly detect the distribution of gaseous methane, it can continuously detect the structural changes of sedimentary layer and hydrate layer, and monitor the formation and development of cracks in real time, and to predict the methane escape channels of gaseous methane based on the change of sediment structure and pore distribution. Therefore, although this technology cannot directly detect methane distribution, it can provide data support for monitoring and control of methane escape through real-time detection of potential methane leakage channels.
3.2 Seawater
The traditional method for detecting methane gas in seawater was to collect water samples with a water sampler, and to obtain the dissolved gas in the laboratory through gas-liquid distribution and purging, then analyze the content of methane in seawater by gas chromatography. Although these analytical methods was mature enough after decades of continuous utilization and improvement, it may cause sample contamination, mixing and dissolved gas escape during the sampling process, and degassing and isotope fractionation of samples in deeper waters, which may cause errors in the test results.
The in situ detection technologies of seawater dissolved gas could be used for underwater real-time and in situ high-resolution observation. The in situ detector can be placed in the subsurface buoy at different depths for continuous monitoring, and can be integrated with other chemical and physical sensors to realize continuous and real-time underwater observation (Figure 2). It provided a new observation method for detecting the abnormal concentration of methane in seawater, discovering new gas hydrate occurrence areas, and deeply understanding the effect of gas hydrate seepage on global climate change and global carbon cycle (Table 3).
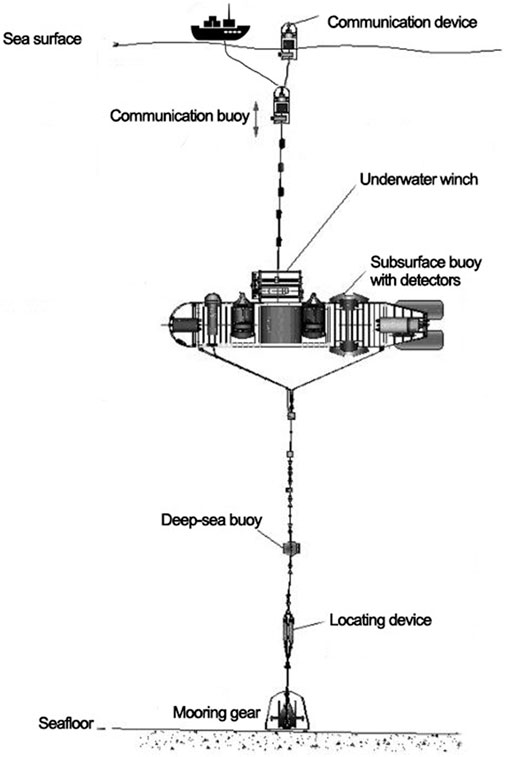
FIGURE 2. Schematic diagram of subsurface buoy with sensors (Xu, 2017).
Currently, most in situ seawater methane detectors are designed on “sampler-analyzer” principle: the high-pressure seawater enters into the instrument through the ballast tank inlet, and forms the seawater at constant pressure through the decompression and flow stabilization device; the gas in the seawater separated through the gas-liquid separation device, and quantitatively sent to the analysis device for testing and output electrical signal. Commonly, samplers include membrane degassing device, decompression shunt devices, etc., and analyzers include electrochemical monitoring, optical analysis, mass spectrometry and biosensors.
3.2.1 Electrochemical sensor
The electrochemical sensor usually uses the semiconductor probe (Garcial and Masson, 2004) in the detection cavity to detect the gas passing through the gas-liquid separation membrane, and outputs the voltage signal, and uses the signal change to reflect the measured gas concentration (Fukasawa et al., 2008). The content of CH4 was measured by the electrochemical reaction between CH4 and adsorbed oxygen under the heating voltage on the surface of the semiconductor material SnO2, which caused the change of electrical conductivity.
The first commercially available SnO2-sensing underwater CH4 sensor (METS) was produced by Capsum in 1999 (Garcial and Masson, 2004). A polydimethylsiloxane (PDMS) membrane was installed under the protective shell to separate dissolved gases from seawater and was supported by a metal sintered plate. The standard METS sensor has a detection range of 10 to 4,000 nmol/L, a maximum operating water depth of 2000 m, and a typical response time of 1–30 min (Di et al., 2014). combined METS sensor and CTD sensor to design an in situ on-line gas flow measurement (GFM) device. The concentration range of CH4 was 50–20 μmol/L and the resolution was less than 10 nmol/L. Anchored over hydrocarbon seps in the northern South China Sea, the device was used for 19 days of in situ measurements to obtain real-time data on gas flow and dissolved CH4 concentrations. Because of the significant lag of METS sensors in the variation of dissolved CH4 concentration, the data need to be corrected.
At present, electrochemical sensors based on semiconductor gas sensitive materials had been widely used in various industries because of their advantages of high precision, low price and small size. However, in the future, it is still necessary to shorten the response time of the instrument, expand the detection range and improve the accuracy of the direction of development and research.
3.2.2 Optical measurement method
The optical measurement methods have the characteristics of non-destructive, fast and high precision, including infrared absorption spectroscopy, fading wave and Raman spectrum.
Infrared absorption spectroscopy, is to irradiate molecules by infrared light at a certain frequency, if the vibration frequency of a group in the molecule is consistent with the external infrared radiation frequency, the energy of light is transferred to the molecule through the change of molecular dipole moment, the group will absorb infrared light of a certain frequency, resulting in a vibration transition. The infrared absorption spectra of the sample can be obtained by recording the molecular absorption of infrared light with instruments. The wavelength, intensity and shape of the absorption peaks in the spectra can be used to judge the groups in the molecules and analyze the structure of the molecules.
The fading wave generated by infrared light at the interface between the waveguide material and its coating can be used to detect the concentration of gas, which is another method of infrared spectroscopy applied to the detection of dissolved gas in seawater (Pejcic et al., 2007). When the light incident from the optically dense medium to the optically sparse medium, if the incident angle was greater than the critical Angle, the phenomenon of total reflection will be generated. The light wave generated along the parallel direction of the critical surface is the fading wave, and its amplitude decreases exponentially with the distance from the critical surface (Yuan et al., 2020). The sensor based on fading wave has the advantages of high sensitivity, simple design and small size, which is conducive to the realization of sensor miniaturization.
Raman spectroscopy is a fast, non-contact and non-destructive molecular vibration spectroscopy technique, which can reflect the internal energy level structure of molecules (Brewer et al., 2004). The principle is: in the process of molecular vibration, polar group vibration, molecular asymmetrical vibration leads to molecular dipole moment changes, produce infrared activity; The non-polar group vibration and the full symmetric vibration of the molecule change the molecular polarizability and produce Raman activity (White et al., 2006). Raman spectra of molecules of different substances may have similar peak positions, but the intensity of the peak is significantly different. The intensity of the peak reflects a large amount of information about molecular structure, so the research on molecular structure mainly focuses on the intensity analysis of the peak (Hester et al., 2007; White, 2009). A unique advantage of Raman spectroscopy in in situ geochemical exploration of the ocean is its ability to measure solid, liquid and gas phases, which greatly expanding its application.
Although the optical measurement methods commonly have high precision and can analyze the gas components in real time. However, limited by the mechanism of optical detection and light wave transmission, the optical measurement method cannot realize quantitative detection, and the detection range in water is much smaller than that on land. Meanwhile, due to the high precision of optical detection equipment, this method has high cost and poor durability.
3.2.3 Underwater mass spectrometry
Underwater mass spectrometry (UMS) is a method for qualitative and quantitative analysis of the mass and strength of material ions (Maher et al., 2015). Gaseous molecules lose an electron after being bombarded by a certain energy electron flow and become positively charged ions. Under the comprehensive action of electric field and magnetic field, these ions are collected by the detector and recorded into a spectrum according to the mass charge ratio (m/z), forming a mass spectrum (Camilli et al., 2010; Gentz and Schlüter, 2012). Applying the analytical function of mass spectrometry to the in situ detection of dissolved substances in Marine water is an important progress in the study of marine chemistry. UMS have been widely used in the study of water chemistry. Current UMS are based on membrane injection mass spectrometry (MIMS) technology, which allows gases and small volatile organic molecules to enter the mass spectrometer directly by using a hydrophobic semi-permeable membrane and applying a vacuum on one side. Some UMS devices are set up on mobile platforms to generate two/three-dimensional maps of chemical concentrations in water bodies (Camilli and Duryea, 2009), while others have been developed to detect in situ isotope ratios and pore water (Bell et al., 2012). The mass spectrometry method has the advantages of short response time, high sensitivity and strong specificity, which can provide the information of elements, structure and isotope of a large number of chemical substances, and can be used to identify some unknown compounds.
Even though UMS has many advantages, due to its complex structure and high precision requirements (Figure 3), the UMS has the disadvantages of high manufacturing and maintenance cost, poor durability and small effective range, which limits the application of this technique. The current UMS technology is still immature, the main goal of related research is to achieve high precision and stable detection of multi-component gas under low power consumption condition.
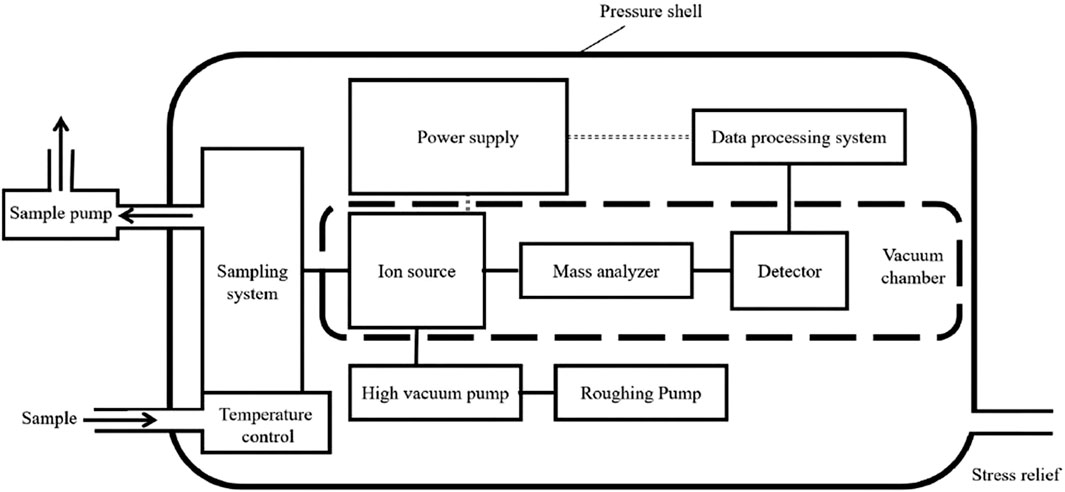
FIGURE 3. Schematic diagram of underwater mass spectrometry system (Gao et al., 2022).
3.2.4 Biosensor
Biosensor is a sensor technology that integrates biotechnology and electronic technology. It converts biochemical reaction into electrical signal by using the principle of biochemistry and electrochemical reaction. Through the processing and detection of electrical signal, the measured substance and its concentration can be measured. Damgaard (Damgaard and Revsbech, 1997) embedded the cultured methane oxidizing bacteria in the oxygen storage sac and the permeable membrane oxygen sensing probe. The oxidation degree of the oxidizing bacteria changed with the change of methane concentration, and the concentration of dissolved methane gas could be measured indirectly by detecting the change of oxygen consumption. The results of seawater experiments of the biomethane sensor show that the measurement response time is 20s, the methane detection range is 50–100 mol/L, and the sensitivity is up to 5 mol/L (Damgaard et al., 2001).
Although biosensors have the ability to detect dissolved gas, they can only be applied in some specific environments because the physiological state of microorganisms is affected by temperature and pH. Its high detection limit and inability to detect isotopes also limit its wide application.
3.3 Atmosphere
Due to the limited application scenarios, atmospheric methane detection during offshore NGH production cannot be carried out by traditional point detection methods, such as catalytic combustion, semiconductor sensors, gas chromatography, etc. A large-scale monitoring device should be placed on offshore platform, monitoring ship, aircraft or even satellite for regional monitoring. Among all the large-scale monitoring technologies, absorption spectrum technology is the most feasible and most widely used. With the development of research and technology, satellite monitoring had become the main technology for atmospheric methane monitoring, The mechanism of the methane monitoring satellite is to estimate the total amount of methane through the spectral absorption of methane at different wavelength positions. The range of remote sensing detection is the methane amount in the column space from the satellite to the ground (Buchwitz et al., 2017). The devices used on the satellite are generally solar backscatter instruments and thermal emitters, while the solar backscatter instruments commonly were used to measure the total amount of methane and the thermal emitters were used to measure the amount of methane in the upper atmosphere. The size range of satellite remote sensing pixels is about 4–50 km2 (Conley et al., 2016). The satellites currently in use operate mainly in sun-synchronous orbits, but isolated missions require remote sensing satellites to be placed in geostationary orbits, which continuously monitor only a fixed area. Generally speaking, methane remote sensing satellites can be used to estimate the total amount of methane in a certain limited area, but the detection accuracy cannot reach the level of specific equipment.
So far, there are 11 methane monitoring satellites in operation around the world, which can achieve real-time monitoring of abnormal methane emissions around the world. However, due to the limitations of monitoring accuracy, data inversion accuracy and anti-disturbance capability, satellite methane monitoring still needs to be further optimized.
4 Conclusion
At present, the most serious environmental threat to gas hydrate production is the methane emission caused by uncontrolled decomposition. In this study, three scenarios in which methane exists in the process of NGH production were put forward: seafloor and sediment, seawater, and atmosphere. Besides, the methane monitoring technologies applicable to the three scenarios were summarized and analyzed, aiming at a complete and full description of the advantages and disadvantages of existing methane monitoring technologies, and providing new ideas and directions for the development of methane monitoring technologies. The main conclusions are as follows.
(1) In terms of technology, the durability, response time and limit of detection of the sensors still need to be improved, and the accuracy, resolution and continuity of the optical detection means have shortcomings. In addition, it is also a way to improve detection capability by combining detection techniques of different mechanisms to cover each other’s shortcomings.
(2) In terms of methodology, the effectiveness of the monitoring system could be improved by optimizing its composition and location. Analyzing the NGH distribution and sediment characteristics based on the preliminary exploration and hydrological data, and carry out numerical simulation to predict the methane leakage characteristics in the early stage, and select the detection method and monitoring point based on the numerical simulation results.
(3) In terms of science, research on the environmental impact of methane emission during NGH production should be strengthened to clarify the impact of methane emissions on marine ecology and greenhouse effect. On this basis, targeted methods to control methane emissions or eliminate the negative effects of methane should be developed.
Author contributions
SL contributed to conception and design of the study and wrote the first draft of the manuscript, MX and XC wrote sections of the manuscript, WP participated in part of the literature research. All authors contributed to manuscript revision, read, and approved the submitted version.
Funding
This study was supported by the CNPC R&D Foundation (Grant NO. 2021DJ4902).
Conflict of interest
The authors declare that the research was conducted in the absence of any commercial or financial relationships that could be construed as a potential conflict of interest.
Publisher’s note
All claims expressed in this article are solely those of the authors and do not necessarily represent those of their affiliated organizations, or those of the publisher, the editors and the reviewers. Any product that may be evaluated in this article, or claim that may be made by its manufacturer, is not guaranteed or endorsed by the publisher.
References
Acharya, T., Pierce, K., and White, M. (2019). Natural gas hydrates: A review of formation, and prevention/mitigation in subsea pipelines. Adv. Sci. Eng. Med. 11 (6), 453–464. doi:10.1166/asem.2019.2384
Bavoh, C. B., Lal, B., Ose, i. H., Sabil, K. M., and Mukhtar, H. (2019). A review on the role of amino acids in gas hydrate inhibition, CO2 capture and sequestration, and natural gas storage. J. Nat. Gas Sci. Eng. 64, 52–71. doi:10.1016/j.jngse.2019.01.020
Bell, R. J., Savidge, W. B., Toler, S. K., Byrne, R. H., and Short, R. T. (2012). In situ determination of porewater gases by underwater flow-through membrane inlet mass spectrometry. Limnol. Oceanogr. Methods 10 (3), 117–128. doi:10.4319/lom.2012.10.117b
Bhattacharya, M., Paramati, S. R., Ozturk, I., and Bhattacharya, S. (2016). The effect of renewable energy consumption on economic growth: Evidence from top 38 countries. Appl. Energy 162 (15), 733–741. doi:10.1016/j.apenergy.2015.10.104
Boer, J. F., Milner, T. E., Gemert, M. J. C., and Nelson, J. S. (1998). Two-dimensional birefringence imaging in biological tissue using polarization sensitive optical coherence tomography. Int. Soc. Opt. Photonics, 3196. doi:10.1117/12.297943
Brewer, P. G., Malby, G., Pasteris, J. D., White, S. N., Peltzer, E. T., Wopenka, B., et al. (2004). Development of a laser Raman spectrometer for deep-ocean science. Deep Sea Res. Part I Oceanogr. Res. Pap. 51 (5), 739–753. doi:10.1016/j.dsr.2003.11.005
Buchwitz, M., Schneising, O., Reuter, M., Heymann, J., Krautwurst, S., Bovensmann, H., et al. (2017). Satellite-derived methane hotspot emission estimates using a fast data-driven method. Atmos. Chem. Phys. 17 (9), 5751–5774. doi:10.5194/acp-17-5751-2017
Busck, J., and Heiselberg, H. (2004). Gated viewing and high-accuracy three-dimensional laser radar. Appl. Opt. 43 (24), 4705. doi:10.1364/AO.43.004705
Camilli, R., and Duryea, A. N. (2009). Characterizing spatial and temporal variability of dissolved gases in aquatic environments with in situ mass spectrometry. Environ. Sci. Technol. 43 (13), 5014–5021. doi:10.1021/es803717d
Camilli, R., Reddy, C. M., Yoerger, D. R., Van Mooy, B. A. S., Jakuba, M. V., Kinsey, J. C., et al. (2010). Tracking hydrocarbon plume transport and biodegradation at deep water horizon. Science 330 (6001), 201–204. doi:10.1126/science.1195223
Chee, S., Leokprasirtkul, T., Kanno, T., Osawa, O., Sudo, Y., Takekoshi, M., et al. (2014). “A deepwater sandface monitoring system for offshore gas hydrate production,” in Offshore technology conference, Texas: OTC, 1–8. doi:10.4043/25328-MS
Claussen, W. F. (1951b). A second water structure for inert gas hydrates. J. Chem. Phys. 19 (11), 1425–1426. doi:10.1063/1.1748079
Claussen, W. F. (1951a). Suggested structures of water in inert gas hydrates. J. Chem. Phys. 19 (2), 259–260. doi:10.1063/1.1748187
Clennell, M. B., Hovland, M., Booth, J. S., Henry, P., and Winters, W. J. (1999). Formation of natural gas hydrates in marine sediments 1: Conceptual model of gas hydrate growth conditioned by host sediment properties. J. Geophys. Res. 104, B1022985–B1023003. doi:10.1029/1999JB900175
Collett, T. S., Boswel, R., Cochran, J. R., Kumar, P., Lall, M., Mazumdar, A., et al. (2014). Geologic implications of gas hydrates in the offshore of India: Results of the national gas hydrate program expedition 01. Mar. Petroleum Geol. 58, 1–2. doi:10.1016/j.marpetgeo.2014.07.020
Collett, T. S. (1999). Detailed evaluation of gas hydrate reservoir properties using JAPEX/JNOC/GSC Mallik 2L-38 gas hydrate research well downhole well-log displays. Bull. Geol. Surv. Can. 544, 295–311.
Conley, S., Franco, G., Faloona, I., Blake, D. R., Peischl, J., and Ryerson, T. B. (2016). Methane emissions from the 2015 aliso Canyon blowout in los angeles, CA. Science 351 (6279), 1317–1320. doi:10.1126/science.aaf2348
Damgaard, L. R., Nielsen, L. P., and Revsbech, N. P. (2001). Methane microprofiles in a sewage biofilm determined with a microscale biosensor. Water Res. 35 (6), 1379–1386. doi:10.1016/s0043-1354(00)00412-7
Damgaard, L. R., and Revsbech, N. P. (1997). A microscale biosensor for methane containing methanotrophic bacteria and an internal oxygen reservoir. Anal. Chem. 69 (13), 2262–2267. doi:10.1021/ac9611576
Demirbas, A., Rehan, M., Al-Sasi, B. O., and Nizami, A. S. (2016). Evaluation of natural gas hydrates as a future methane source. Petroleum Sci. Technol. 34 (13), 1204–1210. doi:10.1080/10916466.2016.1185442
Dharmawardhana, P. B., Parrish, W. R., and Sloan, E. D. (1980). Experimental thermodynamic parameters for the prediction of natural gas hydrate dissociation conditions. Industrial Eng. Chem. Fundam. 19 (4), 410–414. doi:10.1021/i160076a015
Di, P., Feng, D., and Chen, D. (2014). In- situ and on- line measurement of gas flux at a hydrocarbon seep from the northern South China sea. Cont. Shelf Res. 81, 80–87. doi:10.1016/j.csr.2014.04.001
Dickens, G. R., Paull, C. K., and Wallace, P. (1997). Direct measurement of in situ methane quantities in a large gas-hydrate reservoir. Nature 385, 6615426–6615428. doi:10.1038/385426a0
Fujii, T., Suzuki, K., Takayama, T., Tamaki, M., Komatsu, Y., Konno, Y., et al. (2015). Geological setting and characterization of a methane hydrate reservoir distributed at the first offshore production test site on the Daini-Atsumi Knoll in the eastern Nankai Trough, Japan. Mar. Petroleum Geol. 66 (2), 310–322. doi:10.1016/j.marpetgeo.2015.02.037
Fukasawa, T., Oketani, T., Masson, M., Groneman, J., Hara, Y., and Hayashi, M. (2008). “Optimized METS sensor for methane leakage monitoring,” in OCEANS 2008- MTS/IEEE Kobe Techno-Ocean, Kobe, Japan, 08-11 April 2008 (IEEE), 1–8. doi:10.1109/oceanskobe.2008.4530982
Gao, J. Q., Lin, Z. H., Jiang, Y., Xu, H. Y., Dai, X. H., Huang, Z. J., et al. (2022). Research progress of underwater mass spectrometry in situ analysis. Chin. J. Anal. Chem. 50 (5), 666–679. doi:10.19756/j.issn.0253-3820.210570
Garcial, M. L., and Masson, M. (2004). Environmental and geologic application of solid-state methane sensors. Environ. Geol. 46 (8), 1059–1063. doi:10.1007/s00254-004-1093-1
Gentz, T., and Schlüter, M. (2012). Underwater cryotrap-membrane inlet system (CT-MIS) for improved in situ analysis of gases. Limnol. Oceanogr. Methods 10 (5), 317–328. doi:10.4319/lom.2012.10.317
Hatakeyama, Y., Inoue, Y., Takei, T., Sakaguchi, S., Fujii, K., Ikeda, A., et al. (1997). Effects of underwater sounds on fish, fisheries research monograph. Jpn Fish Resour. Conserv. Assoc., Tokyo 47, 73–109.
He, J., Liang, Q. Y., Ma, Y., Shi, Y., and Xiao, Z. (2018). Geohazards types and their distribution characteristics in the natural gas hydrate area on the northern slope of the South China Sea. Geol. China 45 (1), 15–28. doi:10.12029/gc20180102
Hester, K. C., Dunk, R. M., White, S. N., Brewer, P., Peltzer, E., and Sloan, E. (2007). Gas hydrate measurements at Hydrate Ridge using Raman spectroscopy. Geochimica Cosmochimica Acta 71 (12), 2947–2959. doi:10.1016/j.gca.2007.03.032
Hitchon, B. (1974). Occurrence of natural gas hydrates in sedimentary basins. Natural gases in marine sediments. Boston, MA, United States: Springer, 195–225.
Holmes, C. D., Archibald, A. T., Eastham, S. D., and Søvde, O. A. (2017). “The global warming potential of methane reassessed with combined stratosphere and troposphere chemistry,” in American Geophysical Union, Fall Meeting, New Orleans, United States, December, 2017.
IAGC (2002). Airgun arrays and marine mammals. USA: International Association of Geophysical Contructors.
International Energy Agency (IEA) (2019). World energy outlook 2019. Paris: IEA. Available at: https://www.iea.org/reports/world-energy-outlook-2019.
Islam, M. R. (1991). A new recovery technique for gas production from alaskan gas hydrates. J. Petroleum Sci. Eng. 11 (4), 267–281. doi:10.1016/0920-4105(94)90046-9
Ke, W., Svartaas, T. M., and Chen, D. (2019). A review of gas hydrate nucleation theories and growth models. J. Nat. Gas Sci. Eng. 61, 169–196. doi:10.1016/j.jngse.2018.10.021
Khan, M. N., Warrier, P., Peters, C. J., and Koh, C. A. (2016). Review of vapor-liquid equilibria of gas hydrate formers and phase equilibria of hydrates. J. Nat. Gas Sci. Eng. 35, 1388–1404. doi:10.1016/j.jngse.2016.06.043
Khare, V., Nema, S., and Baredar, P. (2016). Solar–wind hybrid renewable energy system: A review. Renew. Sustain. Energy Rev. 58, 23–33. doi:10.1016/j.rser.2015.12.223
Koh, C. A., and Sloan, E. D. (2007). Natural gas hydrates: Recent advances and challenges in energy and environmental applications. AIChE J. 53 (7), 1636–1643. doi:10.1002/aic.11219
Kong, Z., Yin, Z., Cheng, Y., Li, Y., Zhang, Z., and Mei, L. (2020). Modeling and evaluation of the systematic errors for the polarization-sensitive imaging lidar technique. Remote Sens. 12, 203309. doi:10.3390/rs12203309
Krapivina, V. F., Varotsosb, C. A., and Soldatov, V. Y. (2017). Simulation results from a coupled model of carbon dioxide and methane global cycles. Ecol. Model. 359 (10), 69–79. doi:10.1016/j.ecolmodel.2017.05.023
Kumar, R., Linga, P., Moudrakovski, I., Ripmeester, J. A., and Englezos, P. (2008). Structure and kinetics of gas hydrates from methane/ethane/propane mixtures relevant to the design of natural gas hydrate storage and transport facilities. AIChE J. 54 (8), 2132–2144. doi:10.1002/aic.11527
Kvenvolden, K. A. (1995). A review of the geochemistry of methane in natural gas hydrate. Org. Geochem. 23 (11-12), 997–1008. doi:10.1016/0146-6380(96)00002-2
Kvenvolden, K. A., and Barnard, L. A. (1982). Hydrates of natural gas in continental margins: Environmental processes: Model investigations of margin environmental and tectonic processes. Stud. Cont. Margin Geol. A110, 631–640. doi:10.1306/M34430C35
Kvenvolden, K. A., and Lorenson, T. D. (2001). The global occurrence of natural gas hydrate. Nat. Gas Hydrates Occur. Distribution, Detect. Occur. Distribution, Detect. 124, 3–18. American Geophysical Union. doi:10.1029/GM124p0003
Lee, J. Y., Ryu, B. J., Yun, T. S., and Cho, G. C. (2011). Review on the gas hydrate development and production as a new energy resource. KSCE J. Civ. Eng. 15 (4), 689–696. doi:10.1007/s12205-011-0009-3
Li, F., Yuan, Q., Li, T., Li, Z., Sun, C., and Chen, G. (2019). A review: Enhanced recovery of natural gas hydrate reservoirs. Chin. J. Chem. Eng. 27 (9), 2062–2073. doi:10.1016/j.cjche.2018.11.007
Li, J. F., Ye, J. L., Qin, X. W., Qiu, H. J., Wu, N. Y., Lu, H. L., et al. (2018). The first offshore natural gas hydrate production test in South China Sea. China Geol. 1 (1), 5–16. doi:10.31035/cg2018003
Li, M., Tao, Q., Hou, G., and Zhai, J. (2021). A novel sub-bottom profiler seabed sediment classification method based on BPNN with biot-stoll model and attenuation-based model. IEEE Access 9, 53379–53391. doi:10.1109/ACCESS.2021.3071299
Li, X. S., Xu, C. G., Zhang, Y., Ruan, X. K., Li, G., and Wang, Y. (2016). Investigation into gas production from natural gas hydrate: A review. Appl. Energy 172, 286–322. doi:10.1016/j.apenergy.2016.03.101
Li, X. S., Yang, B., Li, G., and Li, B. (2012a). Numerical simulation of gas production from natural gas hydrate using a single horizontal well by depressurization in Qilian Mountain permafrost. Industrial Eng. Chem. Res. 51, 4424–4432. doi:10.1021/ie201940t
Li, X. S., Yang, B., Li, G., Li, B., Zhang, B., and Chen, B. Y. (2012b). Experimental study on gas production from methane hydrate in porous media by huff and puff method in pilot-scale hydrate simulator. Fuel 94, 486–494. doi:10.1016/j.fuel.2011.11.011
Lijith, K. P., Malagar, B. R. C., and Singh, D. N. (2019). A comprehensive review on the geomechanical properties of gas hydrate bearing sediments. Mar. Petroleum Geol. 104, 270–285. doi:10.1016/j.marpetgeo.2019.03.024
Liu, L., Ryu, B. J., Sun, Z., Wu, N., Cao, H., Geng, W., et al. (2019). Monitoring and research on environmental impacts related to marine natural gas hydrates: Review and future perspective. J. Nat. Gas Sci. Eng. 65, 82–107. doi:10.1016/j.jngse.2019.02.007
Liu, S., Peng, P., Liu, Q., and Fan, C. (2019). Economic-related CO2 emissions analysis of Ordos Basin based on a refined STIRPAT model. Greenh. Gases Sci. Technol. 9 (5), 1064–1080. doi:10.1002/ghg.1920
Liu, X., and Zhang, M. (1999). Analysis of main parameters for synchronous scanning underwater laser imaging system. J. Southeast Univ. 29 (1), 6. doi:10.3321/j.issn:1001-0505.1999.01.027
Locat, J., and Lee, H. J. (2002). Submarine landslides: Advances and challenges. Can. Geotechnical J. 39 (1), 193–212. doi:10.1139/t01-089
Macelloni, L., Simonetti, A., Knapp, J. H., Knapp, C. C., Lutken, C. B., and Lapham, L. L. (2012). Multiple resolution seismic imaging of a shallow hydrocarbonplumbing system, Woolsey Mound, Northern Gulf of Mexico. Mar. Petroleum Geol. 38 (1), 128–142. doi:10.1016/j.marpetgeo.2012.06.010
Maher, S., Jjunju, F. P. M., and Taylor, S. (2015). Colloquium: 100 years of mass spectrometry: Perspectives and future trends. Rev. Mod. Phys. 87 (1), 113–135. doi:10.1103/revmodphys.87.113
Majumdar, U., and Cook, A. E. (2018). The volume of gas hydrate-bound gas in the northern Gulf of Mexico. Geochem. Geophys. Geosystems 19 (11), 4313–4328. doi:10.1029/2018GC007865
Makogon, Y. F., and Omelchenko, R. Y. (2013). Commercial gas production from Messoyakha deposit in hydrate conditions. J. Nat. Gas Sci. Eng. 11, 1–6. doi:10.1016/j.jngse.2012.08.002
Maslin, M., Owen, M., Betts, R., Day, S., Dunkley Jones, T., and Ridgwell, A. (2010). Gas hydrates: Past and future geohazard? Philosophical Trans. R. Soc. A Math. Phys. Eng. Sci. 368, 2369–2393. doi:10.1098/rsta.2010.0065
Maslin, M., Owen, M., Day, S., and Long, D. (2004). Linking continental-slope failures and climate change; testing the clathrate gun hypothesis. Geol. (Boulder) 32 (1), 53–56. doi:10.1130/G20114.1
Matsumoto, R., Uchida, T., Waseda, A., Uchida, T., Takeya, S., Hirano, T., et al. (2000). Occurrence, structure, and composition of natural gas hydrate recovered from the Blake Ridge, northwest Atlantic. Proc. Ocean Drill. Program Sci. Results 164, 13–28. doi:10.2973/odp.proc.sr.164.247.2000
Moore, M. T., Phillips, S. C., Cook, A. E., and Darrah, T. H. (2022). Integrated geochemical approach to determine the source of methane in gas hydrate from Green Canyon Block 955 in the Gulf of Mexico. AAPG Bull. 106 (5), 949–980. doi:10.1306/05272120087
Olabi, A. G. (2017). Renewable energy and energy storage systems. Energy 136 (1), 1–6. doi:10.1016/j.energy.2017.07.054
Pearson, C. F., Halleck, P. M., McGuire, P. L., Hermes, R., and Mathews, M. (1983). Natural gas hydrate deposits: A review of in situ properties. J. Phys. Chem. 87 (21), 4180–4185. doi:10.1021/j100244a041
Pejcic, B., Eadington, P., and Ross, A. (2007). Environmental monitoring of hydrocarbons: A chemical sensor perspective. Environ. Sci. Technol. 41 (18), 6333–6342. doi:10.1021/es0704535
Riley, D. C., Marin-Moreno, H., Minshull, T. A., and Schaafsma, M. (2018). “A multi-criteria approach to social, economic and environmental assessment of gas hydrate development projects,” in American Geophysical Union, Fall Meeting.
Schulein, R., and Javidi, B. (2010). Underwater multi-view three-dimensional imaging. J. Disp. Technol. 4 (4), 351–353. doi:10.1109/jdt.2008.924161
Shi, Y. H., Liang, Q. Y., Yang, J. P., Yuan, Q. M., and Kong, L. (2019). Stability analysis of submarine slopes in the area of the test production of gas hydrate in the South China Sea. China Geol. 2 (3), 11. doi:10.31035/cg2018122
Shukla, K. M., Kumar, P., and Yadav, U. S. (2019). Gas hydrate reservoir identification, delineation, and characterization in the Krishna-Godavari basin using subsurface geologic and geophysical data from the national gas hydrate program 02 expedition, offshore India. Mar. Petroleum Geol. 108, 185–205. doi:10.1016/j.marpetgeo.2018.10.019
Song, Y., Zhang, L., Lv, Q., Yang, M., Ling, Z., and Zhao, J. (2016). Assessment of gas production from natural gas hydrate using depressurization, thermal stimulation and combined methods. Rsc Adv. 6 (53), 47357–47367. doi:10.1039/c6ra05526e
Stanković, J., Džunić, M., Džunić, Ž., and Marinkovic, S. (2017). A multi-criteria evaluation of the European cities’ smart performance: Economic, social and environmental aspects. Zb. Rad. Ekon. Fak. au Rijeci 35 (2), 519–550. doi:10.18045/zbefri.2017.2.519
Sun, Z. F., Jia, S., Yuan, Q., Sun, C. Y., and Chen, G. J. (2019). One-dimensional study on gas production characteristics of methane hydrate in clayey sediments using depressurization method. Fuel 262, 116561. doi:10.1016/j.fuel.2019.116561
Takeyaa, S., Kidab, M., Minami, H., Sakagami, H., Hachikubo, A., Takahashi, N., et al. (2006). Structure and thermal expansion of natural gas clathrate hydrates. Chem. Eng. Sci. 61 (8), 2670–2674. doi:10.1016/j.ces.2005.11.049
Tse, J. S. (1990). Thermal expansion of structure-H clathrate hydrates. J. Inclusion Phenom. Mol. Recognit. Chem. 8 (1-2), 25–32. doi:10.1007/bf01131285
Tsuru, T., Amakasu, K., Park, J-O., Sakakibara, J., and Takanashi, M. (2019). A new seismic survey technology using underwater speaker detected a low-velocity zone near the seafloor: An implication of methane gas accumulation in tokyo bay. Earth, Planets Space 71, 31. doi:10.1186/s40623-019-1011-0
Tsuru, T., Park, J-O., No, T., Kido, Y., and Nakahigashi, K. (2018). Visualization of attenuation structure and faults in incoming oceanic crust of the Nankai Trough using seismic attenuation profiling. Earth Planets Space 70, 31. doi:10.1186/s40623-018-0803-y
Tu, Y., Qin, L., Li, E., Ma, H., Zhang, Y., Gao, Y., et al. (2021). Application of time-domain gating technique in water content measurement of gas–liquid two-phase flow. Rev. Sci. Instrum. 92 (9), 094702. doi:10.1063/5.0055810
Tutak, M., and Brodny, J. (2017). “Analysis of methane emission into the atmosphere as a result of mining activity,” in International Multidisciplinary Scientific GeoConference: SGEM (Sofia: Surveying Geology and Mining Ecology Management), 83–90.
Twidell, J., and Weir, T. (2015). Renewable Energy Resources. London: Routledge. doi:10.4324/9781315766416
White, S. N., Dunk, R. M., Peltzer, E. T., Freeman, J. J., and Brewer, P. G. (2006). In situ Raman analyses of deep-sea hydrothermal and cold seep systems (Gorda Ridge and Hydrate Ridge). Geochem. Geophys. Geosystems 7 (5), 123. doi:10.1029/2005GC001204
White, S. N. (2009). Laser Raman spectroscopy as a technique for identification of seafloor hydrothermal and cold seep minerals. Chem. Geol. 259 (3/4), 240–252. doi:10.1016/j.chemgeo.2008.11.008
Xu, L. B. (2017). Underwater winch and the application to the real-time transmission latent buoyage. Ship Sci. Technol. 39 (5), 132–134. doi:10.3404/j.issn.1672-7619.2017.05.026
Yamamoto, K. (2015). Overview and introduction: Pressure core-sampling and analyses in the 2012-2013 MH21 offshore test of gas production from methane hydrates in the eastern Nankai Trough. Mar. Petroleum Geol. 66, 296–309. doi:10.1016/j.marpetgeo.2015.02.024
Yang, G., Zhu, J., Zhao, D., Xiong, Z., Wang, C., Ou, L., et al. (2021). Development and application of sub-bottom profiler technologies. Mar. Sci. 45 (6), 147–162. doi:10.11759/hykx20201030001
Yang, L., Liu, Y., Zhang, H., Xiao, B., Guo, X., Wei, R., et al. (2019). The status of exploitation techniques of natural gas hydrate. Chin. J. Chem. Eng. 27 (9), 2133–2147. doi:10.1016/j.cjche.2019.02.028
Yang, M., Zhao, J., Zheng, J., and Song, Y. (2019). Hydrate reformation characteristics in natural gas hydrate dissociation process: A review. Appl. Energy 256, 113878. doi:10.1016/j.apenergy.2019.113878
Ye, J. L., Qin, X. W., Qiu, H. J., Liang, Q. Y., Dong, Y. F., Wei, J. G., et al. (2018). Preliminary results of environmental monitoring of the natural gas hydrate production test in the South China Sea. China Geol., 202–209. doi:10.31035/cg2018029
Yuan, F., Hu, M., He, Y., Chen, B., Yao, L., Xu, Z., et al. (2020). Development of an in situ analysis system for methane dissolved in seawater based on cavity ringdown spectroscopy. Rev. Sci. Instrum. 91 (8), 083106. doi:10.1063/5.0004742
Keywords: natural gas hydrate, offshore, methane, detection, monitoring system
Citation: Liu S, Xue M, Cui X and Peng W (2023) A review on the methane emission detection during offshore natural gas hydrate production. Front. Energy Res. 11:1130810. doi: 10.3389/fenrg.2023.1130810
Received: 23 December 2022; Accepted: 16 January 2023;
Published: 26 January 2023.
Edited by:
Anna Stoppato, University of Padua, ItalyReviewed by:
Sukru Merey, Batman University, TürkiyeYanghui Li, Dalian University of Technology, China
Copyright © 2023 Liu, Xue, Cui and Peng. This is an open-access article distributed under the terms of the Creative Commons Attribution License (CC BY). The use, distribution or reproduction in other forums is permitted, provided the original author(s) and the copyright owner(s) are credited and that the original publication in this journal is cited, in accordance with accepted academic practice. No use, distribution or reproduction is permitted which does not comply with these terms.
*Correspondence: Shuangxing Liu, c2h1YW5neGluZ19saXVAb3V0bG9vay5jb20=