- 1Department of Chemistry and Biochemistry, University of Regina, Regina, SK, Canada
- 2Transfercentre Sustainable Electrochemistry, Saarland University and KIST Europe, Saarbrücken, Germany
A mini-review is provided of the literature concerning the performance of proton-exchange-membrane fuel cells (PEMFCs) containing (immobilized) ionic liquids as possible electrolytes, focusing on papers reporting performance metrics (power and current densities). For over a decade, and especially for combined heat and power applications, there has been a drive to design an ionic-liquid-holding membrane that could operate well under non-humidifying (water-free) conditions above 100°C. Such a goal has not yet been achieved: reported power densities are still below those of traditional low-temperature fuel cells. Other recent reviews have already pointed to three main issues: poor conductivity, poor cathode (oxygen-reduction) kinetics, and seepage of the liquid from the membrane. In this review, IL-PEMFCs are grouped into three IL classes (protic, aprotic, and polymerized), performance metric data are summarized, the cases producing the top ten peak power densities of the last 5 years are discussed, and the conductivity mechanisms of the leading cases are elucidated. Purely vehicular protic ILs appear to be too slow, and the trend toward enhanced Grotthuss H+ relays via polymerized ILs is revealed.
1 Introduction
Hydrogen fuel cells are electrochemical energy conversion systems with continuous flow of the redox agents (typically hydrogen and oxygen), generating electrical energy and exhaust water. They are thought to be “greener” technology versus hydrocarbon combustion, due to releasing water rather than carbon dioxide (and NOx and SOx) as the exhaust, and achieving higher (>60%) fuel-efficiency (Kirubakarun et al., 2009). Cost efficiency is a research priority.
Inside their membrane–electrode assembly is a hydrogen oxidation reaction at the anode, an oxygen reduction reaction (ORR) at the cathode, and a polymer electrolyte membrane (PEM) betwixt. Anode-created H+ ions (“protons”) travel through the PEM electrolyte to form cathode-created water. A potential cost saving, in part due to reduced contaminant issues (CO and liquid H2O), might be achievable by operating at higher than 100°C, temperatures at which hydrated PEMs (like traditional Nafion,® a sulfonated (RSO3−) perfluoropolymer) dry out and often lose their conductivity (Lee et al., 2010). Currently, the standard higher-temperature PEM is still polybenzimidazole (PBI) doped with phosphoric acid, which works up to 160°C. However, its performance, even at 200 °C and 3 atm pressures of non-humidified gas (1.05 W cm−2, Li et al., 2004), is worse than that of Nafion in 80°C PEMFCs, where power densities of >1.2 W cm−2 are achieved (for e.g., see Breitwieser et al., 2017).
For over a decade, ionic liquids (ILs) have been proposed as alternative proton-carrying wetting agents for PEMs. They are generally composed of relatively large organic cations and inorganic or organic anions that would have difficulty in forming an ordered crystal and thus remain liquid down to and below 100 °C. Generally, as electrolytes, ILs offer low volatility, low flammability, high power intensity, and low weight and volume compared to ordinary aqueous electrolytes. Many are known, and several have been tested in PEM fuel cells (acronym IL-PEMFCs), to temperatures as high as 200°C (Skorikova et al., 2020). We will sub-categorize these IL-PEMFCs as follows:
(i) PIL cases (protic ionic liquids). These can be formed by proton transfer, and their cations have N-H bonds, such as dema (diethylmethylammonium) cations.
(ii) AIL cases (aprotic ionic liquids). The cations here have no N-H bonds, such as quaternary ammonium or dialkylimidazolium (e.g., BMIm, butylmethylimidazolium) cations.
(iii) PolyIL cases (polymerized ionic liquids). One of the ions, usually the cation, has been polymerized or else co-polymerized with another material. PolyILs were pioneered by Ohno (Ohno et al., 1998; Nishimura et al., 2014), who synthesized the first polyILs by attaching vinyl groups to ions and using an azo initiator.
There have been other excellent reviews on studies of ILs for use in PEMFCs, which we will cite and commend. The present mini-review will be unique in focusing on the peak power densities (PPDs) and their improvements that have been reported since 2017, with a discussion focused on the conductivity mechanisms compared to those of hydrated Nafion. Another unique feature will be a summary of recent US patents.
2 Works before 2017
We sense a shift away from the use of the PIL sub-category that had been the focus of two reviews in 2017 (Khan et al., 2017; Watanabe et al., 2017), and therefore we thought it might be useful to summarize the situation at the time of those reviews.
Watanabe et al. (2017) provided a small review on ILs for fuel cells in their large 2017 review on ILs in a variety of battery applications (Li/Na ion batteries, Li–sulfur batteries, and Li–oxygen batteries, as well as fuel cells). First, they commented that PILs might prove to be a good alternative to phosphoric-acid-doped membranes because (i) PILs were known to conduct H+ ions and (ii) phosphoric acid systems were known to have a high over-potential at lower temperatures. Second, they listed properties of PILs appealing for use in PEMFCs: (i) thermal stability (low volatility), best achieved by PILs with proton-transfer strength (ΔpKa) > 15, (ii) proton conductivity, mentioning the Grotthuss and vehicular mechanism possibilities known in bulk PILs and implicitly assuming that both would then also be possible in wetted PEMs, and (iii) electrochemical activity, noting a significant variation (0.6–1.1 V) in the open-circuit potential (OCP) for the H2 + ½ O2 → H2O reaction in various PILs, with a “sweet spot” maximum for PILs (ΔpKa ∼ 17–18; Miran et al., 2013), and noting previous hypotheses that the variations have to do with the number and strength of NH bonds. Third, they discussed PEM fabrication and cited several PIL + PEM examples, with PEMs such as Nafion, polyvinylidene fluoride, block copolymers, and aromatic polymers such as SPI (sulfonated polyimide) and PBI (polybenzimidazoles). The only fuel cell performance metrics they cited were three current densities (70, 170, and ∼400 mA cm−2) and one power density (0.11 W cm−2). The example that produced ∼400 mA cm−2 and 0.11 W cm−2 (Yasuda et al., 2013) was that of an SPI membrane with the PIL [dema][TfO], a PIL arising from a trialkylamine (diethylmethylamine) and a superacid (triflic acid, CF3SO3H).
The Watanabe et al. review then summarized the situation for PILs in fuel cells as somewhat unimpressive, noting that the requirements of the PIL in a non-humidifying fuel cell (for above 100 °C application) are “extremely severe,” and that PIL-infused membranes were not yet satisfactory. However, it concluded that there is enough motivation for such a “dream membrane,” and enough scope for further investigation, to expect more work in this area.
Khan et al. (2017) published a 2017 review that focused on the use of ILs with the ORR (oxygen reduction reaction) in Li/O2 batteries and fuel cells. In their section on ILs and ORR, they noted that early studies found the limitation that such systems tended to perform only two-electron reductions to peroxides, until a paper by Switzer et al. (2013) who demonstrated a four-electron ORR with a PIL, with a platinum (and not glassy carbon) catalyst electrode. The PIL was actually the AIL [BdMIm][TfO] doped with various strong Brønsted acids. Khan et al. then pointed out, as the Watanabe review did, the “sweet spot” of ∆pKa = 17–18 for maximal ORR open-circuit potential in anhydrous PILs and concluded as the Watanabe review did, that [dema][TfO] seemed to be the most promising IL for PEMFCs at the time (at least for non-humidified PEMFC), with its low over-potential and fast ORR kinetics compared to PILs based on the NTf2− anion.
Khan et al. also pointed out that ILs in fuel cells need to also have good mass transport and solubility for the H2 and O2 gases, and studies of such properties have been made. Hydrophobic ILs have been considered, for their ability to enhance expulsion of H2O product, and have interesting effects on the ORR at the platinum electrode surface (improving electrocatalytic activity), but sluggish bulk properties have prevented them from outperforming [dema][TfO] in a fuel cell as of 2017. The Khan et al. review then summarized the situation for ILs in fuel cells as somewhat challenging, citing high viscosity and poor proton conductivity in the experiments up to 2017. They also expressed concern that the full four-electron ORR in PILs had only been found with the Pt electrode, noting that fuel cell developers would like to move to cheaper and more robust materials (less prone to ion or oxide adsorption).
These two reviews focused on the use of ILs without hydration or H3PO4-doping. In the IL-PEMFC literature as of 2017, four of the top five systems in terms of reported peak power density were from hydrated or H3PO4-doped systems: a PIL case with hydrated Nafion (0.56 W cm−2; Sood et al., 2014), an AIL case with H3PO4-doped PBI (0.71 W cm−2; Hooshyari et al., 2016), and two polyIL cases with H3PO4-doped PBI (0.51 W cm−2; Rewar et al., 2014, and 0.32 W cm−2; Xu et al., 2015). Without hydration or H3PO4-doping, Li et al. (2016) managed a power density of 0.42 W cm−2 by replacing the water in hydrated Nafion with an AIL possessing an alcohol (OH) group on the propylmethylimidazolium cation. They hypothesized that this successful step forward in IL-PEMFCs was due to Grotthuss conductivity through the OH groups, as with hydrated Nafion. It was, in fact, their stated design goal, to use an IL to mimic water’s Grotthuss role in hydrated Nafion, for they had felt, like Watanabe et al. and Khan et al. in their aforementioned reviews, that the results of vehicular mechanisms in PIL-PEMFCs (such as with [dema][TfO]) had been somewhat disappointing.
The next section presents the reviews on the developments since 2016.
3 Works during 2017–2022
3.1 IL-PEMFC peak power densities
Figure 1 summarizes peak power densities (PPDs, mW cm−2) of IL-PEMFCs reported during 2010–2022, plotting them against the current densities observed at each PPD. Two radial lines (P = ε I, ε = 0.4 or 0.5) are drawn to show that the effective potentials ε at PPD consistently range between 0.35 and 0.50 V, except for a recent outlier (ε = 0.61 V, PPD = 0.91 W cm−2; Avid et al., 2022). The generally low-effective potentials are due to over-potential and ohmic resistance.
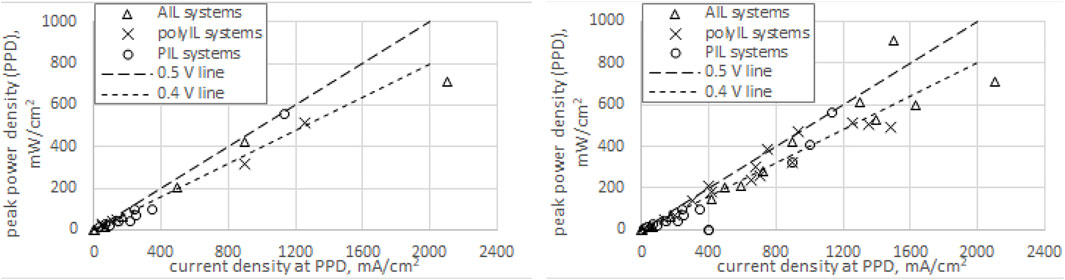
FIGURE 1. Plots of reported IL-PEMFC peak power density (PPD) versus current density at PPD. Left plot: pre-2017 data only. Right plot: data up to and including 2022, revealing a shift from PIL to AIL and polyIL systems. Data and references are available upon request.
Of the top 15 reported PPDs (≥0.32 W cm−2), 10 are new cases (2017–2022) and are described as follows, after some general observations. Of these top 15, nine were achieved by securing acceptable pre-2014 voltages (0.33–0.41 V) at higher and higher current densities, while six achieved improved voltages as well (0.47–0.61 V). Only three of the top 15 are from PIL-based systems (six from AIL and six from polyIL). All 15 employed either hydration or H3PO4-doping. None achieved 1 W cm−2 power density. The 10 new high-power-density cases, in decreasing order of PPD, are:
(i) 0.91 W cm−2 with 1.5 A cm−2 current density (Avid et al., 2022), using hydrated Nafion with the AIL butylmethylimidazolium bistriflimide (written as either [BMIm][NTf2] or [BMIm][TFSI]). They achieved a remarkably improved effective voltage of 0.61 V. The gains they achieved are ascribed by them to an improved electrode design: a high-surface-area mesoporous carbon support containing platinum particles not in contact with the Nafion ionomer, but with the AIL present in the pores assisting water molecules to complete the connection. They comment that the AIL appears to perform well (compared to water) in reducing the electrical double layer that forms around highly charged platinum catalyst nanoparticles, leading to improved ORR kinetics. They misstate the reasons for good H+ conductivity of the AIL in the mesopores, borrowing the Watanabe idea of a high ∆pKa, but this is not relevant to AILs. We suspect that the AIL may be assisting a H3O+/H2O Grotthuss relay through the electrical double layer.
(ii) 0.61 W cm−2 with 1.3 A cm−2 current density (Rajabi et al., 2020), using PBI mixed in organic solution with dendrimer-amine-functionalized mesoporous silica with the AIL [Pr (MIm)2][Br]2, dried, later doped with H3PO4, and run at 180 °C temperature. They noted that the presence of the AIL and the functionalized silica both improved the H3PO4 uptake of PBI.
(iii) 0.60 W cm−2 with 1.6 A cm−2 current density (Zanchet et al., 2021), using the AIL [C16MIm][TfO] with hydrated Nafion and a zeolite filler. This AIL has a long hexadecyl alkyl arm.
(iv) 0.53 W cm−2 with 1.4 A cm−2 current density (da Trindade et al., 2020), using the hydrated sulfonated polymer SPEEK (sulfonated poly ether ether ketone) with the AIL [BMIm][HSO4]. Though technically an “aprotic” IL, its amphiphilic anion could be participating in the usual hydrated-SPEEK Grotthuss relay. The ionic liquid effect was a 40% power enhancement at 100°C relative to the power density of hydrated SPEEK alone.
(v) 0.508 W cm−2 with 1.35 A cm−2 current density (Xiao et al., 2022), using what may be a polyIL, made by cross-linking the AIL [VEIm][H2PO4] with PBI with added functionalized carbon nanotubes, and later doped with H3PO4. It may, however, be the case that the AIL is simply physisorbed in the system, given that the PBI is made before the AIL and carbon nanotubes are added. Here, V is a vinyl group.
(vi) 0.495 W cm−2 with 1.5 A cm−2 current density (Li et al., 2022), using what may be a polyIL, made by cross-linking the AIL [VBIm][NTf2] with a fluoro PBI (6FPBI), and later doped with H3PO4. As with the previous case, the AIL may only be physisorbed here.
(vii) 0.47 W cm−2 with 0.93 A cm−2 current density (Ma et al., 2021), using an interesting polyIL, made from co-polymerizing biphenyl, TFAc (1,1,1-trifluoroacetone), 1-methyl-4-piperidone, a beta cyclodextrin, and the AIL bromohexylmethylpiperidinium bromide, later doped with water. This is a hydrated cationic membrane, known as an anion exchange membrane, unlike hydrated Nafion which is a hydrated anionic membrane.
(viii) 0.41 W cm−2 with ∼1 A cm−2 current density (da Trindade et al., 2019a), from a polymer blend of hydrated SPEEK with more resilient PBI (polybenzimidazole), paired with the heavily sulfonated PIL [Et3NCH2CH2CH2SO3H][HSO4].
(ix) 0.39 W cm−2 with 0.75 A cm−2 current density (Liu et al., 2021), from a polyIL named NbPBI-TPAm doped with H3PO4. The polyIL was made from polymerizing a diacid form of norbornene (Nb) with the usual ingredients to make PBI and later cross-linked using the quaternary ammonium AIL TPAmBr (triethylpent-4-enylammonium bromide).
(x) 0.32 W cm−2 with 0.90 A cm−2 current density (Skorikova et al., 2020), using quasi-solidified closed-pore PBI containing the ionic liquid [dema][NTf2] and doped with H3PO4.
3.2 Other IL-PEMFC properties
For a summary of modern studies of the IL leaching (seepage) problem, we recommend the review of Ebrahimi et al. (2021), in which their Section 4 and Table 3 deals specifically with the leaching issue. For a summary of modern studies of IL effects on ORR kinetics, we recommend the short review of Wippermann et al. (2022) and add to this the mention of a newer paper by Korte et al., which noticed, using atomic force microscopy, an altered double-layer structure near a Pt (100) surface when doping [dema][TfO] with water (Rodenbücher et al., 2021).
A special focus on IL-PEM conductivity can be found in two large reviews on IL-PEMs and PEMFCs by Elwan et al. (2021a); Elwan et al. (2021b). The first review, on AIL and PIL systems, online December 2020, is 30 pages long citing 298 references and provides a general review on a great many IL-PEM systems, grouped by PEM polymers used (Nafion, PBI, SPEEK, etc.). It also touches on the leaching and ORR kinetics issues. In its summary remarks, it notes the challenges with IL leakage (leaching) from PEMs and the challenging trade-off between conductivity and mechanical stability and recommends more computational modeling of PIL-PEMs with more focus on conductivity mechanisms, to improve currently achieved conductivities. It also recommends more testing of IL-PEMs in actual fuel cells. In the cases since early 2017 that they report, there are four cases of conductivities above 100 mS cm−1 that we surmise might be worthy of testing in fuel cells (Wang et al., 2018; da Trindade et al., 2019b; Zakeri et al., 2019a; Zakeri et al., 2019b). Their second review, on polyIL systems, online August 2021, is 29 pages long citing 285 references and again recommends more testing of polyIL-PEMs in actual fuel cells. Two other reviews on polyIL-PEMs have appeared very recently (Wong et al., 2022; Zunita et al., 2022).
An important IL-PEM conductivity paper that they did not cite is one by Smith et al. (2019). They made interesting cyclic voltammetry and polarization measurements to demonstrate that the pure ionic liquid [dema][TfO] cannot, in fact, spontaneously perform proton conduction for H2 + ½ O2 → H2O at 22°C unless doped with excess acid or base, and that the cell potential depends so systematically on the pKa of an acid dopant HA that the depositor of H+ onto the ORR cathode is more likely to be excess acid, HA, rather than the protonated base BH+ as speculated by Miran et al. (2013) and Watanabe et al. (2017). This observation is important for our conductivity discussion in the next section.
4 Discussion
Some of the faith initially put in PILs as alternative wetting agents may have been due to a belief that the “proton” in PILs, the one transferred from the acid to the base, would be an “active” proton, whether in a vehicular mechanism (postulated by Watanabe for anhydrous PILs) or in a Grotthuss relay through an amphiphilic medium (as in water, or ILs with the anions HSO4− or H2PO4−). Although [dema][TfO] produced higher conductivity than the supposedly Grotthuss-capable [dema]HSO4] and [dema][H2PO4] (Watanabe et al., 2017), the effects of the presence of excess acid (intentional or accidental) were not well understood. The 10 new top-performing IL-PEMFC cases discussed in Section 3.1 all involve hydration or H3PO4-doping, providing Grotthuss conductivity that we conjecture is being additionally stabilized by the presence of IL or polyIL. In Figure 2, we offer our best hypotheses for the conductivity mechanisms occurring in PIL-PEMFC membranes to date. We think that Grotthuss-conductivity stabilization is, at the moment, the best design strategy, and polyILs may prove fruitful in this regard.
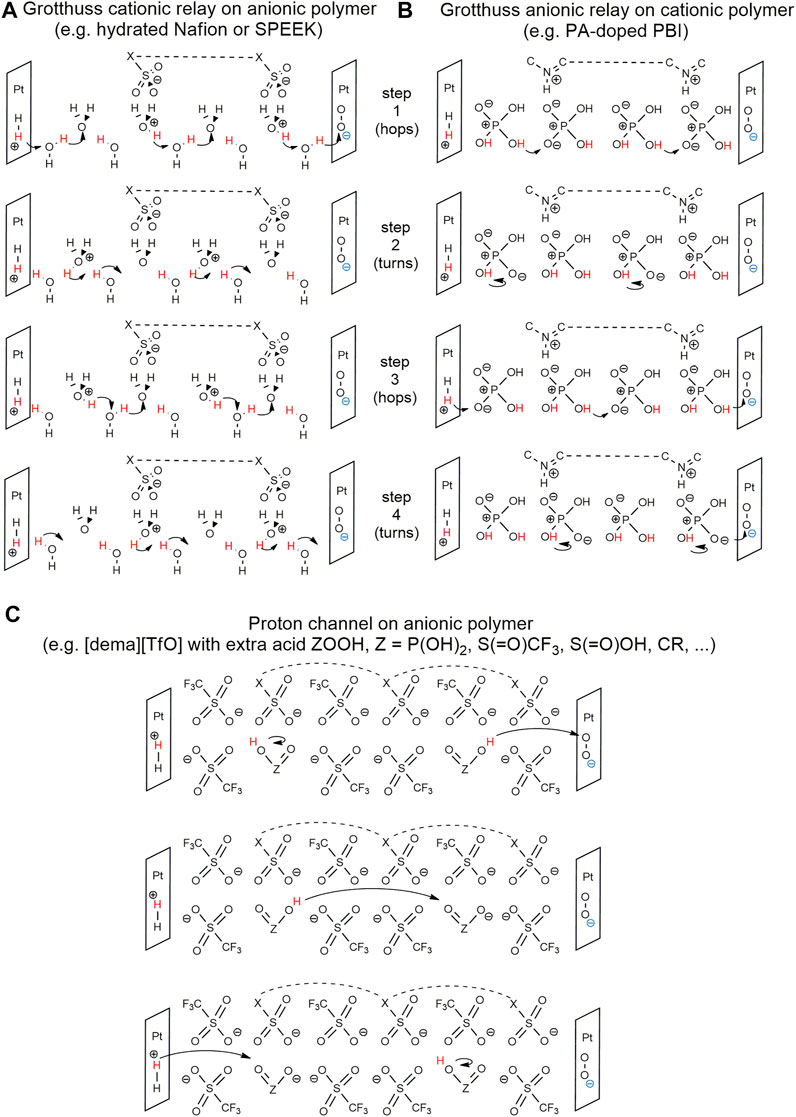
FIGURE 2. Hypothetical mechanisms (A–C) for H+ conductivity through IL-PEMs to the ORR cathode. The protonated-dema countercations in mechanism C are assumed to be in nearby stabilizing positions.
Reconsidering the PIL-PEMFC cases of Watanabe ([dema][TfO] and anionic sulfonated polymers) that managed power densities of 0.06–0.11 W cm−2 at 120°C with no amphiphilic dopant (Miran et al., 2013; Yasuda et al., 2013), Watanabe’s hypothesis of a vehicle mechanism for these, with the amines as vehicle-carrying active protons, is unlikely to be true, for two reasons. First is that any base B formed from deprotonation of BH+ at the cathode has no electromotive impetus to travel to the anode, and a significant fraction of them would be rendered inert before they get there, by the strong TfOH entities formed initially at the anode. Second is that Smith et al. (2019) provide strong evidence that acid impurities, if present, would be the deliverers of H+ in the ORR at the cathode, and we like their hypothesis that some acid impurities may have appeared in Watanabe’s systems due to accidental amine loss to vaporization at elevated temperatures. We instead propose an ion channeling conductivity mechanism for these cases, with strong excess-acid protons lightly skipping along sulfonate groups (mechanism C in Figure 2).
It is hoped that this insight may aid future designs of IL-PEMFCs with improved conductivity performance.
5 Appendix: US patent summary, 2017–2022
“Ionic liquid as promotor to enhance the performance of oxygen reduction catalyst for fuel cell application” (10,777,823), by Huang et al., claims an ORR catalyst consisting of platinum nanoparticles, with or without carbon, together with an ionic liquid consisting of a methylhexahydropyrimidopyrimidinium nonafluoro butanesulfonate. The authors speculate that the perfluoro nature of the anion aids in preventing water adsorption at the electrode surfaces.
“Block copolymer, multilayered structure, solid polymer membrane, fuel cell, method for producing multilayered structure, and method for producing multilayered structure including inorganic nanoparticles” (10,851,237), by Yabu, claims a block copolymer that can produce a solid membrane including nanoparticles and a solvent. It does not specify particular ionic liquids for the solvent. The block copolymer contains catechol (a particular dihydroxyphenyl) groups for desired hydrophilicity.
“Hydrocarbon-based cross-linked membrane in which nanoparticles are used, method for manufacturing said membrane, and fuel cell”(10,868,322), by J. Kim, claims a cross-linked composite membrane for PEMFCs made from sulfonated polyphenylsulfone and sulfonated polyhedral oligomeric silsesquioxane, cross-linked by sulfonic acid groups. There is no mention of the use of ionic liquids in such membranes in the patent.
“Catalyst and electrode catalyst layer, membrane electrode assembly, and fuel cell using the catalyst” (11,031,604), by Mashio et al., claims a catalyst (electrode) with pores for excellent gas transportability for fuel cells. No specific ionic liquid is mentioned; ionic liquid is listed as a possibility for the proton-conducting electrolyte to carry protons from the electrode metal to the PEM.
“Composite proton-conducting membrane” (11,196,072), by Gervasio et al., claims a composite proton-conducting membrane consisting of 70–90 wt% inorganic polymer with pores filled with 30–10 wt% organic polymer, with both polymers able to conduct protons up to about 220°C with no added water or solvent. The inorganic polymer appears to be a ceramic form of tin pyrophosphate anion (TiP2O74-) doped perhaps with some trivalent metal ions and H+. The organic polymer is a “quaternary ammonium-biphosphate ion-pair-coordinated polyphenylene”; the ionic liquid cation (the quaternary ammonium) may be tethered to the polymer, with biphosphate anions (H2PO4−), perhaps affording a Grotthuss relay in a semicrystalline state.
Author contributions
CN performed an initial literature and patent review, draft summary of some papers, and made a preliminary version of AE’s Figure 2. AE performed two more literature reviews and a patent review and wrote the manuscript, including Figure 1 and finalizing Figure 2. RM proofread the manuscript and provided input on the introduction and Figure 2.
Funding
AE and CN participation was funded by Canada’s Natural Sciences and Engineering Research Council (Discovery Grant RGPIN-2017-06247).
Acknowledgments
The authors thank K. E. Johnson (University of Regina) for discussion of Smith and Walsh’s cyclic voltammogram results.
Conflict of interest
The authors declare that the research was conducted in the absence of any commercial or financial relationships that could be construed as a potential conflict of interest.
Publisher’s note
All claims expressed in this article are solely those of the authors and do not necessarily represent those of their affiliated organizations, or those of the publisher, the editors, and the reviewers. Any product that may be evaluated in this article, or claim that may be made by its manufacturer, is not guaranteed or endorsed by the publisher.
References
Avid, A., Ochoa, J. L., Huang, Y., Liu, Y., Atanassov, P., and Zenyuk, I. V. (2022). Revealing the role of ionic liquids in promoting fuel cell catalysts reactivity and durability. Nat. Commun. 13, 6349. doi:10.1038/s41467-022-33895-5
Breitwieser, M., Klose, C., Klingele, M., Hartmann, A., Erben, J., Cho, H., et al. (2017). Simple fabrication of 12 μm thin nanocomposite fuel cell membranes by direct electrospinning and printing. J. Power Sources 337, 137–144. doi:10.1016/j.jpowsour.2016.10.094
da Trindade, L. G., Borba, K. M. N., Zanchet, L., Lima, D. W., Trench, A. B., Rey, F., et al. (2019b). SPEEK-Based proton exchange membranes modified with MOF-encapsulated ionic liquid. Mat. Chem. Phys. 236, 121792. doi:10.1016/j.matchemphys.2019.121792
da Trindade, L. G., Zanchet, L., Martins, P. C., Borba, K. M., Santos, R. D., Paiva, R. D. S., et al. (2019a). The influence of ionic liquids cation on the properties of sulfonated poly (ether ether ketone)/polybenzimidazole blends applied in PEMFC. Polymer 179, 121723. doi:10.1016/j.polymer.2019.121723
da Trindade, L. G., Zanchet, L., Souza, J. C., Leite, E. R., Martini, E. M. A., and Pereira, E. C. (2020). Enhancement of sulfonated poly(ether ether ketone)-based proton exchange membranes doped with different ionic liquids cations. Ionics 26, 5661–5672. doi:10.1007/s11581-020-03684-5
Ebrahimi, M., Kujawski, W., Fatyeyeva, K., and Kujawa, J. (2021). A review on ionic liquids-based membranes for middle and high temperature polymer electrolyte membrane fuel cells (PEM FCs). Int. J. Mol. Sci. 22, 5430. doi:10.3390/ijms22115430
Elwan, H. A., Mamlouk, M., and Scott, K. (2021a). A review of proton exchange membranes based on protic ionic liquid/polymer blends for polymer electrolyte membrane fuel cells. J. Power Sources 484, 229197. doi:10.1016/j.jpowsour.2020.229197
Elwan, H. A., Thimmappa, R., Mamlouk, M., and Scott, K. (2021b). Applications of poly ionic liquids in proton exchange membrane fuel cells: A review. J. Power Sources 510, 230371. doi:10.1016/j.jpowsour.2021.230371
Hooshyari, K., Javanbakht, M., and Adibi, M. (2016). Novel composite membranes based on PBI and dicationic ionic liquids for high temperature polymer electrolyte membrane fuel cells. Electrochim. Acta 205, 142–152. doi:10.1016/j.electacta.2016.04.115
Khan, A., Gunawan, C. A., and Zhao, C. (2017). Oxygen reduction reaction in ionic liquids: Fundamentals and applications in energy and sensors. ACS Sus. Chem. Eng. 5, 3698–3715. doi:10.1021/acssuschemeng.7b00388
Kirubakaran, A., Jain, S., and Nema, R. K. (2009). A review on fuel cell technologies and power electronic interface. Renew. Sus. Energy Rev. 13, 2430–2440. doi:10.1016/j.rser.2009.04.004
Lee, S.-Y., Ogawa, A., Kanno, M., Nakamoto, H., Yasuda, T., and Watanabe, M. (2010). Nonhumidified intermediate temperature fuel cells using protic ionic liquids. J. Am. Chem. Soc. 132, 9764–9773. doi:10.1021/ja102367x
Li, Q., He, R., Jensen, J. O., and Bjerrum, N. J. (2004). PBI-based polymer membranes for high temperature fuel cells – preparation, characterization and fuel cell demonstration. Fuel Cells 4, 147–159. doi:10.1002/fuce.200400020
Li, Y., Shao, W., Ma, Z., Zheng, M., and Song, H. (2022). Performance analysis of a HT-PEMFC system with 6FPBI membranes doped with cross-linkable polymeric ionic liquid. Int. J. Mol. Sci. 23, 9618. doi:10.3390/ijms23179618
Li, Y., Shi, Y., Mehio, N., Tan, M., Wang, Z., Hu, X., et al. (2016). More sustainable electricity generation in hot and dry fuel cells with a novel hybrid membrane of nafion/nano-silica/hydroxyl ionic liquid. Appl. Energy 175, 451–458. doi:10.1016/j.apenergy.2016.03.075
Liu, F., Wang, S., Wang, D., Liu, G., Cui, Y., Liang, D., et al. (2021). Multifunctional poly(ionic liquid)s cross-linked polybenzimidazole membrane with excellent long-term stability for high temperature-proton exchange membranes fuel cells. J. Power Sources 494, 229732. doi:10.1016/j.jpowsour.2021.229732
Ma, L., Hussain, M., Li, L., Qaisrani, N. A., Bai, L., Jia, Y., et al. (2021). Octopus-like side chain grafted poly(arylene piperidinium) membranes for fuel cell application. J. Membr. Sci. 636, 119529. doi:10.1016/j.memsci.2021.119529
Miran, M. S., Yasuda, T., Susan, M. A. B. H., Dokko, K., and Watanabe, M. (2013). Electrochemical properties of protic ionic liquids: Correlation between open circuit potential for H2/O2 cells under nonhumidified conditions and ΔpKa. RSC Adv. 3, 4141–4144. doi:10.1039/c3ra23354e
Nishimura, N., and Ohno, H. (2014). 15th anniversary of polymerised ionic liquids. Polymer 55, 3289–3297. doi:10.1016/j.polymer.2014.02.042
Ohno, H., and Ito, K. (1998). Room-temperature molten salt polymers as a matrix for fast ion conduction. Chem. Lett. Jpn. 27, 751–752. doi:10.1246/cl.1998.751
Rajabi, Z., Javanbakht, M., Hooshyari, K., Badiei, A., and Adibi, M. (2020). High temperature composite membranes based on polybenzimidazole and dendrimer amine functionalized SBA-15 mesoporous silica for fuel cells. New J. Chem. 44, 5001–5018. doi:10.1039/c9nj05369g
Rewar, A. S., Chaudhari, H. D., Illathvalappil, R., Sreekumar, K., and Kharul, U. K. (2014). New approach of blending polymeric ionic liquid with polybenzimidazole (PBI) for enhancing physical and electrochemical properties. J. Mat. Chem. A 2, 14449–14458. doi:10.1039/c4ta02184c
Rodenbücher, C., Chen, Y., Wippermann, K., Kowalski, P. M., Giesen, M., Mayer, D., et al. (2021). The structure of the electric double layer of the protic ionic liquid [dema] [TfO] analyzed by atomic force spectroscopy. Int. J. Mol. Sci. 22, 12653. doi:10.3390/ijms222312653
Skorikova, G., Rauber, D., Aili, D., Martin, S., Li, Q., Henkensmeier, D., et al. (2020). Protic ionic liquids immobilized in phosphoric acid-doped polybenzimidazole matrix enable polymer electrolyte fuel cell operation at 200°C. J. Membr. Sci. 608, 118188. doi:10.1016/j.memsci.2020.118188
Smith, D. E., and Walsh, D. A. (2019). The nature of proton shuttling in protic ionic liquid fuel cells. Adv. Energy Mater 9, 1900744. doi:10.1002/aenm.201900744
Sood, R., Iojoiu, C., Espuche, E., Gouanvé, F., Gebel, G., Mendil-Jakani, H., et al. (2014). Comparative study of proton conducting ionic liquid doped nafion membranes elaborated by swelling and casting methods: Processing conditions, morphology, and functional properties. J. Phys. Chem. C 118, 14157–14168. doi:10.1021/jp502454m
Switzer, E. E., Zeller, R., Chen, Q., Sieradzki, K., Buttry, D. A., and Friesen, C. (2013). Oxygen reduction reaction in ionic liquids: The addition of protic species. J. Phys. Chem. C 117, 8683–8690. doi:10.1021/jp400845u
Wang, X., Wang, S., Liu, C., Li, J., Liu, F., Tian, X., et al. (2018). Cage-like cross-linked membranes with excellent ionic liquid retention and elevated proton conductivity for HT-PEMFCs. Electrochim. Acta 283, 691–698. doi:10.1016/j.electacta.2018.06.197
Watanabe, M., Thomas, M. L., Zhang, S., Ueno, K., Yasuda, T., and Dokko, K. (2017). Application of ionic liquids to energy storage and conversion materials and devices. Chem. Rev. 117, 7190–7239. doi:10.1021/acs.chemrev.6b00504
Wipperman, K., and Korte, C. (2022). Effects of protic ionic liquids on the oxygen reduction reaction - a key issue in the development of intermediate-temperature polymer-electrolyte fuel cells. Curr. Op. Electrochem. 32, 100894. doi:10.1016/j.coelec.2021.100894
Wong, C. Y., Wong, W. Y., Loh, K. S., and Lim, K. L. (2022). Protic ionic liquids as next-generation proton exchange membrane materials: Current status and future perspectives. Reac. Func. Polym. 171, 105160. doi:10.1016/j.reactfunctpolym.2022.105160
Xiao, Y., Shen, X., Sun, R., Wang, S., Xiang, J., Zhang, L., et al. (2022). Enhanced proton conductivity and stability of polybenzimidazole membranes at low phosphoric acid doping levels via constructing efficient proton transport pathways with ionic liquids and carbon nanotubes. J. Power Sources 543, 231802. doi:10.1016/j.jpowsour.2022.231802
Xu, C., Liu, X., Cheng, J., and Scott, K. (2015). A polybenzimidazole/ionic-liquid-graphite-oxide composite membrane for high temperature polymer electrolyte membrane fuel cells. J. Power Sources 274, 922–927. doi:10.1016/j.jpowsour.2014.10.134
Yasuda, T., and Watanabe, M. (2013). Protic ionic liquids: Fuel cell applications. MRS Bull. 38, 560–566. doi:10.1557/mrs.2013.153
Zakeri, M., Abouzari-Lotf, E., Nasef, M. M., Ahmad, A., Miyake, M., Ting, T. M., et al. (2019a). Fabrication and characterization of supported dual acidic ionic liquids for polymer electrolyte membrane fuel cell applications. Arab. J. Chem. 12, 1011–1023. doi:10.1016/j.arabjc.2018.05.010
Zakeri, M., Abouzari-Lotf, E., Nasef, M. M., Ahmad, A., Ripin, A., Ting, T. M., et al. (2019b). Preparation and characterization of highly stable protic ionic-liquid membranes. Int. J. Hydrogen Energy 44, 30732–30742. doi:10.1016/j.ijhydene.2018.04.015
Zanchet, L., da Trindade, L. G., Trombetta, F., Martins, A. D., Martini, E. M. A., Becker, M. R., et al. (2021). Improving nafion/zeolite nanocomposite with a CF3CO3− based ionic liquid for PEMFC application. Ionics 27, 2027–2036. doi:10.1007/s11581-021-03918-0
Keywords: ionic liquids, fuel cells, PEM, power density, proton transport, Grotthuss, ORR
Citation: East ALL, Nguyen CM and Hempelmann R (2023) A 2023 update on the performance of ionic-liquid proton-exchange-membrane fuel cells. Front. Energy Res. 11:1031458. doi: 10.3389/fenrg.2023.1031458
Received: 30 August 2022; Accepted: 09 January 2023;
Published: 26 January 2023.
Edited by:
Sandeep Arya, University of Jammu, IndiaReviewed by:
Suman Lata Tripathi, Lovely Professional University, IndiaAnoop Singh, University of Jammu, India
Copyright © 2023 East, Nguyen and Hempelmann. This is an open-access article distributed under the terms of the Creative Commons Attribution License (CC BY). The use, distribution or reproduction in other forums is permitted, provided the original author(s) and the copyright owner(s) are credited and that the original publication in this journal is cited, in accordance with accepted academic practice. No use, distribution or reproduction is permitted which does not comply with these terms.
*Correspondence: Allan L. L. East, YWxsYW4uZWFzdEB1cmVnaW5hLmNh