- 1MIIT Key Laboratory of Thermal Control of Electronic Equipment, School of Energy and Power Engineering, Nanjing University of Science and Technology, Nanjing, China
- 2Advanced Combustion Laboratory, School of Energy and Power Engineering, Nanjing University of Science and Technology, Nanjing, China
As soot particles from incomplete combustion of fossil fuels pose a great threat to human health, the development of low-carbon or zero-carbon alternative fuels is essential to mitigate climate change. An experimental and numerical study on the pyrolysis properties of ammonia and ethylene mixtures is conducted, focusing on the properties of soot generated by pyrolysis under different conditions and the coupling relationship between soot properties and soot precursors. The results show that the graphitization degree of soot particles generated at higher pyrolysis temperatures is enhanced, but the oxidation reactivity is decreased. When ammonia is blended, the graphitization of soot decreases and the oxidation reactivity increases. The peak mole fractions of soot precursors are negatively correlated with the graphitization degree of soot particles as the temperature increases.
Introduction
Global environmental problems are becoming increasingly serious as countries industrialize. The incomplete combustion of hydrocarbon fuels inevitably generates soot particles, which negatively affect the environment and climate system and pose a great threat to human health, making the development of environmentally friendly and sustainable alternative fuels essential.
In the context of carbon peaking and carbon neutrality goals, there is an urgent need to develop some environmentally friendly alternative fuels, such as hydrogen, ammonia, and bio-derived oxides (Zheng et al., 2015). Ammonia (NH3) is a carbon-free fuel with early application in agriculture and a long history of mass production, mainly as fertilizer for intensive farming, so its production, storage, and transportation systems are more mature, which provides a good basis for the application of ammonia (Chen et al., 2020). In addition, ammonia has the advantages of high energy density, good economy, safety, and reliability, so it has a very broad application prospect. However, pure ammonia is difficult to burn and has a narrow ignition range, so traditional hydrocarbon fuels are considered for blending with it.
In recent years, scholars have conducted a lot of research on the combustion characteristics, combustion mechanism, and combustion pollutant emission of ammonia, and some results have been achieved. Experimental and simulation research is conducted to assess the effects of the addition of hydrogen on ammonia combustion characteristics and the NOx generation (Lee et al., 2010). The effects of NH3/CH4 molar ratio (R) on flame temperature and mole fraction of each substance are investigated, meanwhile, a new combustion mechanism that can correctly predict the concentration distribution of intermediates and products is proposed (Tian et al., 2009). A further mechanism is proposed to show the NH3 oxidation process and predicted the maximum velocity reaction of laminar combustion rate (Han et al., 2020). Studying the combustion mechanism of ammonia can help to verify or develop NH3 mixed combustion mechanisms for complex fuels, on the other hand, challenges such as low combustibility of ammonia and NOx emissions are expected to be overcome by knowledge of combustion kinetics and chemistry (Kobayashi et al., 2019). Both the physical and chemical properties of soot extracted from toluene flames depend on the fuel flow (Peña et al., 2017). The characteristics of ethylene/ammonia mixture counterflow diffusion flame are investigated, and it is observed that the soot volume fraction (SVF) and the average soot diameter decrease with the addition of ammonia (Zhou et al., 2022). The effects of butanol isomers as fuel-side additives on the microstructure and reactivity of soot are investigated in ethylene normal and inverse diffusion flames (Ying and Liu, 2017). Studying the microstructure and reactivity of soot helps to better understand the soot formation process and develop efficient soot emission reduction technologies.
The generation of soot during the pyrolysis of zero-carbon fuel ammonia and conventional hydrocarbon fuels mixture still needs further investigation. The study aims to analyze the influences of ammonia on the important soot precursors and the properties of soot generated by pyrolysis, expecting to provide experimental reference and theoretical support for the practical application of ammonia energy and control of combustion pollutants. Based on the flow tube pyrolysis experimental system, the soot generated under different pyrolysis conditions is collected, and the graphitization degree and oxidation reactivity of the soot are analyzed by Raman and TGA, respectively. To obtain the coupling relationship between soot yield, the concentration distribution of soot precursors, and the soot properties, the pyrolysis process is simulated by CHEMKIN.
Research system and methodology
The flow tube pyrolysis experimental system in this study is the same as the previous work (Zang and Liu, 2021). A high-temperature tube furnace is used to provide a stable high-temperature reaction environment for fuel pyrolysis. The tube length is 600 mm and the inner diameter is 45 mm. During the experiment, argon gas is used as the protective gas and a high precision digital mass flow controller, model CS200A, is used to monitor the gas flow rate instantaneously and control the gas flow rate accurately.
The most common hydrocarbon, ethylene, is selected as the base fuel. Ethylene is an important component of many hydrocarbon fuels and also an important intermediate product generated by pyrolysis, a large amount of research data has been accumulated. The blending ratio of ammonia is set at 40%. To investigate the effect of pyrolysis temperatures on soot properties, two different reaction temperatures of 1323 and 1423 K are set. When the temperature has risen to the set value, the fuel starts to pass through the tube furnace for pyrolysis, and the reaction time is set to 60 min. The detailed pyrolysis conditions are shown in Table 1.
The soot samples are collected by deposition sampling method. The soot particles are collected from the glass fiber filter membrane which is located at the outlet of the flow tube, and the soot mass is weighed by comparing the filter membrane mass before and after the pyrolysis process. The characterization methods are the same as the previous studies (Ying and Liu, 2018). Briefly, the graphitization degree of the soot is analyzed by Raman and the soot oxidation reactivity is tested by TGA.
To further understand the process of carbon soot generation, the CHEMKIN/PFR is used to carry out the pyrolysis chemical reaction kinetic simulations. The mole fraction distribution of important soot precursors can be calculated to investigate the relationship between soot properties and soot precursors. The simulations are performed using a kinetic mechanism, the A-G mechanism, for the ammonia chemical reaction that has been validated in the literature (Zhou et al., 2022). The mechanism combines a comprehensive nitrogen chemistry model (Glarborg et al., 2018) with the AramcoMech mechanism, and the detailed mechanism contains 966 reaction components and 4,678 primitive reactions, which can be applied well to the present study.
Results and discussion
Soot mass
By analyzing the soot mass collected under different conditions, the effects of pyrolysis temperature and ammonia blending on soot formation can be found. Table 2 lists the soot mass under different conditions. Pyrolysis of ethylene blended with ammonia at 1323 K has the lowest soot production of 18.8 mg. For pure ethylene pyrolysis, the soot mass at 1423 K is 1.21 times higher than that at 1323 K. For ethylene blended with ammonia pyrolysis, the soot mass at 1423 K is 1.95 times higher than that at 1323 K. It is found that increasing the temperature leads to increased soot formation, furthermore, the effect of temperature on soot formation after blending with ammonia is exacerbated. At 1323 K, the soot generation after ammonia blending accounts for 30% of that in pure ethylene. At 1423 K, the soot generation after blending is 48.6% of that in pure ethylene. It shows that ammonia blending has a significant inhibiting effect on soot formation, and the inhibiting effect is stronger at low temperatures.
Analysis of soot graphitization degree
The microstructure of soot is tested by Raman spectroscopy, and the 5-peak fitting method (Sadezky et al., 2005) is used for the analysis of Raman spectra. The G band located near 1580 cm−1, D1(D) band located near 1350 cm−1, D2 and D4 bands located at about 1620 cm−1 and 1180 cm−1 are all fitted with Lorentz function, and D3 band located near 1500 cm−1 is fitted with Gaussian function. Figure 1 shows the first-order region of the Raman spectra and the split-peak fitting curves of pyrolysis-generated soot under each condition. The variation between the G and D bands is most obvious in Figure 1B, indicating that the soot generated by pure ethylene pyrolysis at 1423 K is the most graphitized.
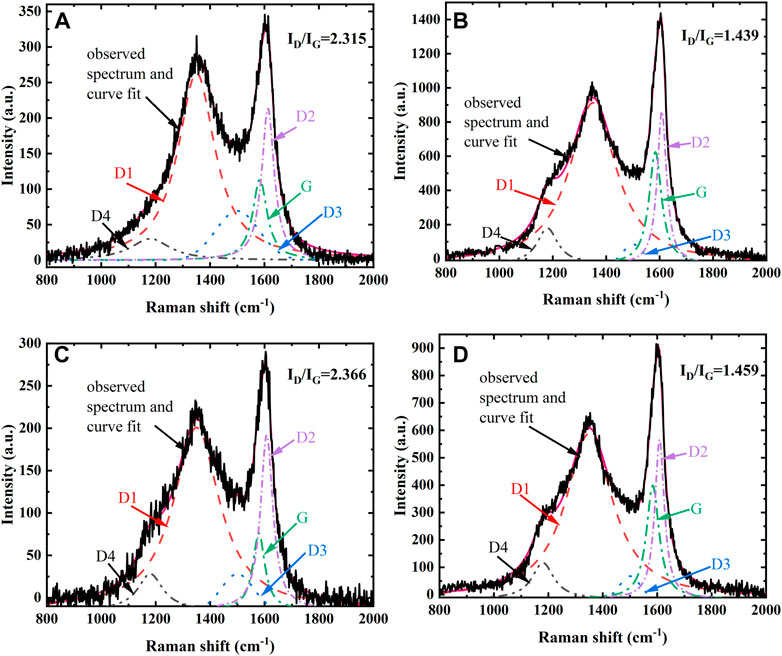
FIGURE 1. First-order Raman spectra and split-peak fitting curves of soot from different conditions, (A) 1323 K, (B) 1423 K of pure ethylene pyrolysis, (C) 1323 K, (D) 1423 K ethylene-doped ammonia pyrolysis.
Since the G band is a graphitic band and D1 band is a disordered band, the ratio of the intensity of D1 bend and G band is used to characterize the degree of graphitization and internal structural orderliness of soot (Al-Qurashi and Boehman, 2008). In addition, ID/IG is inversely proportional to the more graphitic structure aromatic islands (Commodo et al., 2017), which enlarges with increasing amorphous and disordered carbon (Ess et al., 2016). Figure 1 also gives the ID/IG value of the soot. As the temperature increased from 1323 to 1423 K, the ID/IG values reduced by 1.6 times for pure ethylene pyrolysis and 1.6 times for ammonia blending pyrolysis, respectively, indicating that the temperature had a significant influence on soot graphitization degree. With the increasing temperature, the graphitization degree of soot increases, which may be because the soot particles generated by pyrolysis under high temperature undergo significant carbonization, thus reducing the amorphous carbon content and enhanced the structural orderliness (Ferreira et al., 2010). The effect of ammonia blending on the ID/IG values can be ignored compared to temperature, and the ratio increased slightly after ammonia blending. From the analysis of the first-order region of the Raman spectra and split-peak fitting curves of soot and the ID/IG values, it can be concluded that the soot generated at higher pyrolysis temperatures has a higher graphitization degree and the degree of graphitization decreases after ammonia blending.
Analysis of soot oxidation reactivity
The reactivity of soot is characterized by a thermogravimetric analyzer (TGA). Figure 2 shows the weight loss curves of the pyrolysis-generated soot at a constant temperature of 500°C. The larger slope of the curve indicates a faster oxidation rate. Taking the low-temperature condition of pure ethylene pyrolysis as the base condition, it can be seen that the order of oxidation rate from largest to smallest is as follows: pyrolysis of ammonia and ethylene mixtures at 1323 K is the fastest, the base condition, then soot generated by pyrolysis of ammonia and ethylene mixtures at 1423 K, and the oxidation rate of soot produced by pyrolysis of pure ethylene at 1423 K is the lowest. In summary, with the increase in pyrolysis temperature, the oxidation rate of the soot slows down. After blending with ammonia, the oxidation rate of soot increases.
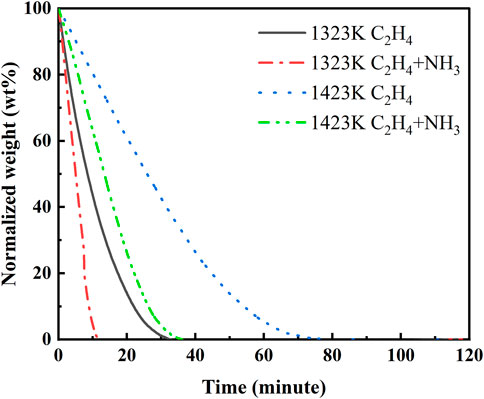
FIGURE 2. Oxidation curves for different pyrolysis conditions generating carbon soot particles at 500°C.
There is often a strong link between the soot oxidation reactivity and soot microstructure (Vander Wal et al., 2010). Coupling the results of Raman tests with TGA tests, it can be found that the ID/IG values characterizing the soot graphitization increase when the pyrolysis temperature decreases, in other words, the degree of graphitization as well as the structural orderliness of soot decreases and the oxidation reactivity increases. In addition, soot graphitization enhances and the oxidation rate decreases when ammonia is mixed.
Analysis of coupling between soot properties and pyrolysis gas phase chemistry
The investigation of the relationship between the concentration distribution of important soot precursors and the soot properties is conducive to furthering our understanding of soot formation. The peak mole fractions of some soot precursors, including A1 (benzene), A2 (naphthalene), A3 (anthracene), and A4 (pyrene), are coupled to the ID/IG values of Raman spectra. The coupling results are shown in Figure 3.
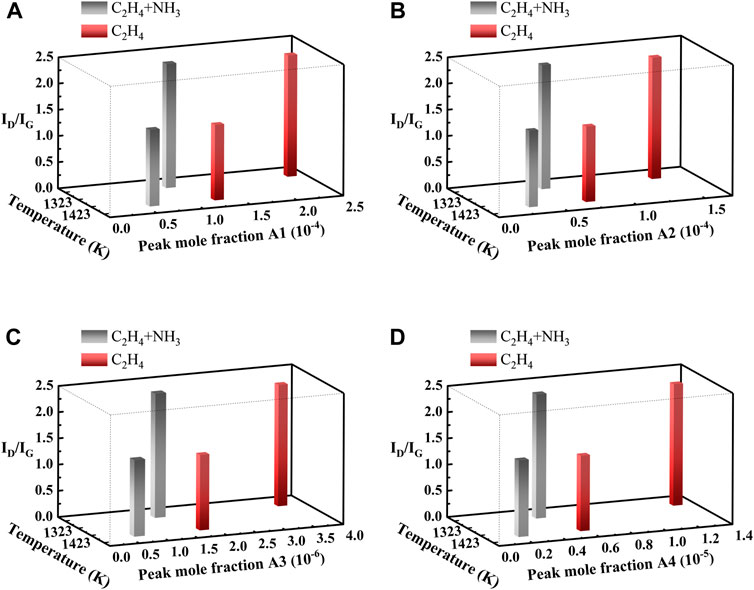
FIGURE 3. Coupling plots of peak mole fractions of A1, A2, A3, and A4 with ID/IG obtained from simulations of different pyrolysis conditions: (A) A1, (B) A2, (C) A3, (D) A4.
Polycyclic aromatic hydrocarbons (PAHs) are important soot precursors. A1 is the simplest cyclic aromatic hydrocarbon produced during combustion. Related studies show that the benzene ring is the basis for the growth of PAHs (Wang, 2011; Dobbins, 2017), which grows through cyclization to produce stable PAH molecules. The HACA mechanism describes the growth process of PAHs and finally generates soot through a series of reactions (Frenklach, 2002). Therefore, the mole fraction distributions of A1, A2, A3, and A4 are selected. It is essential to study the generation pattern of important soot precursors under different pyrolysis conditions to control the soot mass.
As shown in the xy plane in Figure 3, the x-axis represents the temperature and the y-axis represents the peak mole fractions of soot precursors, the peak mole fractions of A1, A2, A3, and A4 show a common trend. As the temperature increases, the peak mole fractions of A1, A2, A3, and A4 all decrease. At the same temperature, the peak mole fractions of soot precursors produced by the pyrolysis of ethylene and ammonia mixtures are lower than those produced by the pyrolysis of pure ethylene. This pattern indicates that the addition of ammonia can effectively reduce the production of some soot precursors.
The z-axis represents the ID/IG value, which shows that the peak mole fraction of each soot precursor tends to change with temperature in the same way as the ID/IG value, both decrease with the increasing temperature. The ID/IG value reflects the graphitization degree and the structural orderliness of the soot. Based on the above discussion, it can be inferred that the higher the peak mole fractions of A1, A2, A3, and A4, the weaker the soot graphitization and the stronger the oxidation reactivity of soot.
Conclusion
This study investigates the soot properties generated by pyrolysis of ammonia and conventional hydrocarbon fuel ethylene mixture at different temperatures by a combination of experiments and simulations, and the relationship between the soot gas-phase chemistry and soot properties is further analyzed. The main results are summarized as follows:
1) Temperature has a significant effect on the graphitization and oxidation reactivity of soot generated by pyrolysis. The soot generated at higher pyrolysis temperature has higher graphitization degree and lower oxidation reactivity. When blending ammonia, the soot graphitization is decreased and the oxidation reactivity is enhanced.
2) When blending ammonia, the peak mole fractions of soot precursors including A1, A2, A3, and A4 all decrease, the soot graphitization degree reduces, and the oxidation reactivity enhances.
Data availability statement
The original contributions presented in the study are included in the article/Supplementary Material, further inquiries can be directed to the corresponding authors.
Author contributions
JH: investigation, data curation, formal analysis, and writing-original draft; YY: methodology, supervision, writing-review and editing, paper revision, and funding acquisition; MC: investigation and validation; DL: supervision, project administration, and funding acquisition.
Funding
This work was supported by the National Natural Science Foundation of China [52106160, 52076110], the Natural Science Foundation of Jiangsu Province [BK20200490], State Key Laboratory of Engines, Tianjin University, and the Fundamental Research Funds for the Central Universities [30920031103].
Conflict of interest
The authors declare that the research was conducted in the absence of any commercial or financial relationships that could be construed as a potential conflict of interest.
Publisher’s note
All claims expressed in this article are solely those of the authors and do not necessarily represent those of their affiliated organizations, or those of the publisher, the editors and the reviewers. Any product that may be evaluated in this article, or claim that may be made by its manufacturer, is not guaranteed or endorsed by the publisher.
References
Al-Qurashi, K., and Boehman, A. L. (2008). Impact of exhaust gas recirculation (EGR) on the oxidative reactivity of diesel engine soot. Combust. Flame 15, 675–695. doi:10.1016/j.combustflame.2008.06.002
Chen, D., Li, J., Huang, H., Chen, Y., He, Z., and Deng, L. (2020). Progress in ammonia combustion and reaction mechanism. Chemistry 83, 508–515. doi:10.14159/j.cnki.0441-3776.2020.06.004
Commodo, M., D’Anna, A., Falco, G. D., Larciprete, R., and Minutolo, P. (2017). Illuminating the earliest stages of the soot formation by photoemission and Raman spectroscopy. Combust. Flame 181, 188–197. doi:10.1016/j.combustflame.2017.03.020
Dobbins, R. A. (2017). Hydrocarbon nanoparticles formed in flames and diesel engines. Aerosol Sci. Technol. 41, 485–496. doi:10.1080/02786820701225820
Ess, M. N., Bladt, H., Mühlbauer, W., Seher, S. I., Zöllner, C., Lorenz, S., et al. (2016). Reactivity and structure of soot generated at varying biofuel content and engine operating parameters. Combust. Flame 163, 157–169. doi:10.1016/j.combustflame.2015.09.016
Ferreira, E. H. M., Moutinho, M. V. O., Stavale, F., Lucchese, M. M., Capaz, R. B., Jorio, A., et al. (2010). Evolution of the Raman spectra from single-few-and many-layer graphene with increasing disorder. Phys. Rev. B 82, 125429. doi:10.1103/physrevb.82.125429
Frenklach, M. (2002). Reaction mechanism of soot formation in flames. Phys. Chem. Chem. Phys. 4, 2028–2037. doi:10.1039/b110045a
Glarborg, P., Miller, J. A., Ruscic, B., and Klippenstein, S. J. (2018). Modeling nitrogen chemistry in combustion. Prog. Energy Combust. Sci. 67, 31–68. doi:10.1016/j.pecs.2018.01.002
Han, X., Wang, Z., He, Y., Zhu, Y., and Cen, K. (2020). Experimental and kinetic modeling study of laminar burning velocities of NH3/syngas/air premixed flames. Combust. Flame 213, 1–13. doi:10.1016/j.combustflame.2019.11.032
Kobayashi, H., Hayakawa, A., Somarathne, K., and Okafor, E. (2019). Science and Technology of ammonia combustion. Proc. Combust. Inst. 37, 109–133. doi:10.1016/j.proci.2018.09.029
Lee, J. H., Kim, J. H., Park, J. H., and Kwon, O. C. (2010). Studies on properties of laminar premixed hydrogen-added ammonia/air flames for hydrogen production. Int. J. Hydrogen Energy 35, 1054–1064. doi:10.1016/j.ijhydene.2009.11.071
Peña, G. D. J. G., Raj, A., Stephen, S., Anjana, T., Hammid, Y. A. S., Brito, J. L., et al. (2017). Physicochemical Properties of Soot generated from toluene diffusion flames: Effects of fuel flow rate. Combust. Flame 178, 286–296. doi:10.1016/j.combustflame.2017.01.009
Sadezky, A., Muckenhuber, H., Grothe, H., Niessner, R., and Pöschl, U. (2015). Raman microspectroscopy of soot and related carbonaceous materials: Spectralanalysis and structural information. Carbon 43, 1731–1742. doi:10.1016/j.carbon.2005.02.018
Tian, Z., Li, Y., Zhang, L., Glarborg, P., and Qi, F. (2009). An experimental and kinetic modeling study of premixed NH3/CH4/O2/Ar flames at low pressure. Combust. Flame 156, 1413–1426. doi:10.1016/j.combustflame.2009.03.005
Vander Wal, R. L., Bryg, V. M., and Hays, M. D. (2010). Fingerprinting soot (towards source identification): Physical structure and chemical composition. J. Aerosol Sci. 41, 108–117. doi:10.1016/j.jaerosci.2009.08.008
Wang, H. (2011). Formation of nascent soot and other condensed-phase materials in flames. Proc. Combust. Inst. 33, 41–67. doi:10.1016/j.proci.2010.09.009
Ying, Y., and Liu, D. (2017). Effects of butanol isomers additions on soot nanostructure and reactivity in normal and inverse ethylene diffusion flames. Fuel 205, 109–129. doi:10.1016/j.fuel.2017.05.064
Ying, Y., and Liu, D. (2018). Nanostructure evolution and reactivity of nascent soot from inverse diffusion flames in CO2, N2, and He atmospheres. Carbon 139, 172–180. doi:10.1016/j.carbon.2018.06.047
Zang, L., and Liu, D. (2021). Formation and characteristics of soot from pyrolysis of ethylene blended with furan fuels. Sci. China Technol. Sci. 64 (3), 585–598. doi:10.1007/s11431-019-1561-0
Zheng, D., Yu, W., and Zhong, B. (2015). RP-3 aviation kerosene surrogate fuel and the chemical reaction kinetic model. Acta Physico-Chimica Sin. 31, 636–642. doi:10.3866/pku.whxb201501231
Keywords: ammonia, soot particles, chemical reaction kinetics, graphitization degree, oxidation reactivity, soot precursors
Citation: He J, Ying Y, Chen M and Liu D (2022) Soot formation characteristics in hybrid pyrolysis of zero-carbon fuel ammonia and ethylene mixtures. Front. Energy Res. 10:996813. doi: 10.3389/fenrg.2022.996813
Received: 18 July 2022; Accepted: 29 August 2022;
Published: 14 September 2022.
Edited by:
Cong Luo, Huazhong University of Science and Technology, ChinaReviewed by:
Zunhua Zhang, Wuhan University of Technology, ChinaGianluigi De Falco, DICMAPI—University of Naples Federico II, Italy
Longhua Hu, University of Science and Technology of China, China
Yu Qiao, Huazhong University of Science and Technology, China
Copyright © 2022 He, Ying, Chen and Liu. This is an open-access article distributed under the terms of the Creative Commons Attribution License (CC BY). The use, distribution or reproduction in other forums is permitted, provided the original author(s) and the copyright owner(s) are credited and that the original publication in this journal is cited, in accordance with accepted academic practice. No use, distribution or reproduction is permitted which does not comply with these terms.
*Correspondence: Yaoyao Ying, eWluZ3lhb3lhb0BuanVzdC5lZHUuY24=; Dong Liu, ZG9uZ2xpdUBuanVzdC5lZHUuY24=
†These authors have contributed equally to this work