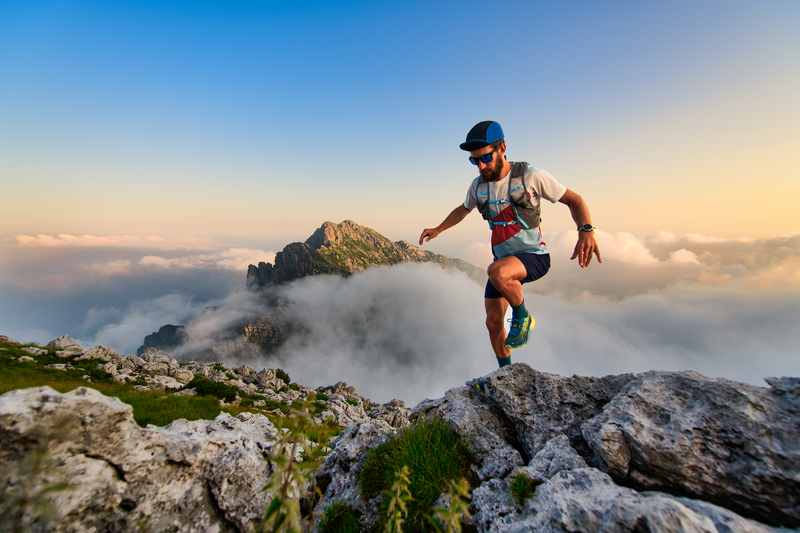
95% of researchers rate our articles as excellent or good
Learn more about the work of our research integrity team to safeguard the quality of each article we publish.
Find out more
ORIGINAL RESEARCH article
Front. Energy Res. , 17 January 2023
Sec. Carbon Capture, Utilization and Storage
Volume 10 - 2022 | https://doi.org/10.3389/fenrg.2022.995800
This article is part of the Research Topic Rising Stars in Carbon Capture, Utilization and Storage: 2022 View all 6 articles
The catalytic production of higher alcohols (HAs) is a promising path for converting CO2 into value-added chemical products. The application is still limited by the low selectivity of HAs (less than 10%) on most catalysts. Here, we report a tandem catalyst consisting of Mn-Cu-K modified iron carbide and CuZnAlZr catalyst. The modification of iron carbide with Mn, Cu and K promoters improves the formation of HAs (13.5% Sel.), and the construction of tandem catalysts with CuZnAlZr can further enhance the catalytic performance. By examining different catalyst filling methods and the filling ratio of the tandem catalyst, it was found that the powder mixing resulted in a higher selectivity of HAs with a mass ratio of the two components of 1:1, and a synergistic effect leads to a higher selectivity of HAs (15.5%) with about 40% of propanol and butanol among HAs.
The increase in global CO2 emissions has led to a gradual increase in atmospheric CO2 concentration, which has been linked to a series of environmental problems such as global climate change, melting glaciers and rising sea levels. As a part of an integrated solution (including the increased use of renewable energy, CO2 capture, utilization and storage), catalytic conversion of CO2 into chemicals with added value, such as methanol, lower olefins and liquid fuels, can not only reduce the CO2 concentration, but also relieve the dependence on fossil fuels (Liang et al., 2019; Tada et al., 2019; Gao et al., 2020; Temvuttirojn et al., 2020; Wu et al., 2021) In recent years, there has been significant interest in the hydrogenation of CO2 into HAs (represents ethanol and alcohols with carbon number above 2 such as propanol and butanol). This is because higher alcohols require catalysts with both the ability to form oxygenated compounds and the ability to grow carbon chains compared to methanol, and alcohol products with long carbon chains have higher industrial applications (Luk et al., 2017; Zeng et al., 2021) HAs is widely used as fuels and solvents in industry and consumed in large quantities each year, and the demand for HAs is likely to increase significantly in the future given the rapid growth in the world’s demand for chemicals and fuels.
HAs are mainly produced through the hydration of petroleum-derived olefins and the fermentation of starch. However, these methods are agriculture-dependent and have low productivity. Therefore, it is necessary to develop new and efficient methods to produce HAs (Xu et al., 2020a) At present, much progress has been made in the hydrogenation of CO2 to methanol, and a few catalysts have been applied in industrial scale applications (Behrens et al., 2012; Temvuttirojn et al., 2020) However, direct conversion of CO2 to HAs is currently still a grand challenge (Zhao et al., 2018; Nie et al., 2019; Yang et al., 2019; Zhang et al., 2021) Most studies on CO2 hydrogenation to HAs focused on modified Fischer-Tropsch Synthesis (FTS) catalysts (Fe and Co catalysts), modified methanol synthesis catalysts (Cu-based catalysts) and Rh-based catalysts, etc. (Ning et al., 2016; Luk et al., 2017; Zhao et al., 2017; Ao et al., 2018; Cao et al., 2018) The synthesis of HAs requires rationally designed catalysts to promote the growth of carbon chains and to accelerate the insertion of oxygen-containing intermediates (e.g., CHO*, CO) into the carbon chains (Yang et al., 2019; Zhang et al., 2021)
Fe-based catalysts have recently been extensively studied in the production of hydrocarbons via CO2 hydrogenation (Liu et al., 2018; Liu et al., 2019; Liu et al., 2020; Zhu et al., 2020; Zhu et al., 2022), and Fe5C2 is essential for carbon chain growth (Liu et al., 2021) Iron carbide has moderate chain growth ability, higher activity and lower cost than Co and Ce based catalysts, so it is commonly used for CO2/CO hydrogenation to produce C2∼C4 hydrocarbons. Fe-Mn catalyst is active for the hydrogenation of CO2 to HAs, and Mn is usually used as a structural as well as electronic promoter, which can improve the ability of CO insertion (Kangvansura et al., 2016; Yang et al., 2018; Nie et al., 2019) Martin et al. reported a K/Mn/Fe/NCNT catalyst for CO2 hydrogenation, where alcohols were observed in products containing methanol and ethanol (8.4% in total), but not in products with longer carbon chains (Kangvansura et al., 2016; Kangvansura et al., 2017) In addition, Fe-Cu catalyst has been used for the production of HAs from syngas with high activity. Metallic Cu is responsible for the adsorption and insertion of non-dissociated CO, and the FeCx-Cu interface promotes the formation of HAs (Xu et al., 2020a; Xu et al., 2020b) Alkali metals such as Na, K and Cs are widely used as promoters, and they can inhibit the further hydrogenation of the adsorbed CxHy intermediates, providing more opportunities for the formation of higher alcohols and olefins (Iranmahboob et al., 2003; Tienthao et al., 2007; Christensen et al., 2009; Cosultchi et al., 2012; Portillo Crespo et al., 2015; Xu et al., 2020a) Furthermore, alkali promoters can also promote the formation and stabilization of Fe5C2, facilitating the chain growth (Xu et al., 2020b) Besides, Liu et al. reported a tandem catalyst composed of CuZnAl and K-CuMgZnFe for the synthesis of HAs in CO2 hydrogenation, and the proper proximity of the two components accelerates the transfer of CO* intermediates from CuZnAl to K-CuMgZnFe (Xu et al., 2021) Wang et al. reported a Na-Fe@C catalyst and demonstrated that oxygen-containing compounds can effectively migrate at the interface of Fe5C2 as well as CuZnAl oxides, which further promotes the formation of higher alcohols. Therefore, we speculate that the modified iron carbide catalyst in our work is equally capable of species migration with the CuZnAlZr catalyst, leading to the improvement of the selectivity of higher alcohols (Wang et al., 2021) Sun et al. reported a CoMn/CuZnAlZr catalyst for the preparation of HAs, where the CuZnAlZr catalyst can effectively promote the formation of CHxO* intermediates, and the abundant CHxO* intermediates participate in the subsequent HAs formation. Therefore, we prepared a similar CuZnAlZr catalyst and tested it in tandem with our MnCuK-FeC catalyst (Lin et al., 2019)
In this work, Mn, Cu and K were used to modify iron carbide and enhance its ability to produce more HAs. A CuZnAlZr catalyst was prepared and coupled with Mn-Cu-K modified iron carbide for CO2 hydrogenation. By testing different catalyst filling methods and filling mass ratios, it was found that the catalysts performed best when mixed with 1:1 powder, and the selectivity of HAs in the total product reached 15.5%.
Ferrous oxalate (Aladdin Chemicals, >99%), aqueous manganese nitrate (Aladdin Chemicals, 50%), copper nitrate (Cu(NO3)2·6H2O, Aladdin Chemicals, >99%), potassium nitrate (Aladdin Chemicals, >99%), zinc nitrate (Zn(NO3)2·6H2O, Aladdin Chemicals, >99%), aluminum nitrate (Al(NO3)3·9H2O, zirconium nitrate (Zr(NO3)4·5H2O, Aladdin Chemicals, >99%), anhydrous sodium carbonate (Aladdin Chemicals, >99%).
Mn-Cu-K modified iron carbide catalysts were prepared by a previously reported method (Liu et al., 2021) Typically, 2.0 g of ferrous oxalate, 0.65 g of aqueous manganese nitrate, 0.38 g of copper nitrate and 0.06 g of potassium nitrate were mixed at room temperature. The obtained composite was kept at room temperature for 48 h and then dried at 60°C for 24 h. The obtained powder was then placed in a tube furnace and heated to 350°C at 0.45°C/min under a 10% CO-N2 atmosphere, and then carburized for 4 h. After natural cooling of the catalyst to room temperature, the gas was switched to 1% O2-N2 for 24 h to passivate it. The product is denoted as MnCuK-FeC. The unmodified FeC catalyst was prepared in a similar way without the introduction of any other promoters.
CuZnAlZr catalyst was prepared by a previously reported co-precipitation method (Lin et al., 2019) 9.66 g of copper nitrate, 3.79 g of zinc nitrate, 7.51 g of aluminum nitrate, and 0.86 g of zirconium nitrate were dissolved in 40 ml of deionized water. Next, 12.72 g of anhydrous sodium carbonate was subsequently weighed and dissolved in 60 ml of deionized water. The above two solutions were slowly added dropwise to a beaker containing 200 ml of deionized water at a controlled temperature of 30°C and a pH of 8.0. After stirring at 30°C for 3 h, the resulting suspension was centrifuged, washed, and dried at 80°C for 24 h. Finally, the dried product was calcined in a muffle furnace at 350°C with a heating rate of 5°C/min for 4 h to obtain the CuZnAlZr catalyst. The subsequent catalyst composed with MnCuK-FeC and CuZnAlZr (mass ratio = 1:1) was named as MnCuK-FeC/CuZnAlZr.
X-ray diffraction (XRD) patterns were obtained on a Rigaku SmartLab diffractometer with Cu Kα radiation (λ = 1.5406 Å) at a scan rate of 8°/min. The 2θ degree range was from 10° to 80°. In-situ XRD experiments were conducted with an XRK 900 reaction chamber manufactured by Anton Paar to track the phase transition. Typically, 60 mg of catalyst was loaded into a gas-tight chamber and pre-carburized in pure CO at 350°C and ambient pressure. After the sample was cooled to 300°C, the feed gas was switched to the reaction gas (CO2:H2:N2 = 21:63:16) at a rate of 30 ml/min and the reaction pressure was maintained at 0.8 MPa.
CO2-temperature programmed desorption (TPD) was measured using a Quantachrome ChenBETPulsar analyzer. Approximately 120 mg of passivated catalyst was loaded and pretreated with 10% H2-Ar for 25 min at 400°C to remove the surface passivated layer. The gas was then switched to CO2 for 1 h at room temperature to achieve saturated adsorption. The atmosphere was changed to He to start the analysis procedure, and the sample was then heated to 700°C at 10°C/min.
We obtained in-situ diffuse reflectance infrared Fourier transform (DRIFT) spectra on a Thermo NicoletTM iSTM50 spectrometer with a liquid nitrogen-cooled mercury cadmium telluride (MCT) detector. Before testing, we pretreated samples with 10% CO-N2 at 350°C and ambient pressure for 1 h, and then samples were purged with N2 and cooled to 300°C and the background was collected. The reactant gas (CO2: H2 = 1:3) was fed to the samples at 300°C and 1 MPa, then the spectra were subsequently obtained.
Fourier Transform Infrared spectroscopy (FT-IR) measurements were carried out on a Thermo Scientific NicoletTM iSTM50 spectrometer. The spectra were collected from 500 to 4000 cm−1 with a resolution of 4 cm−1.
Micro Confocal Raman Spectroscopy measurements were carried out on a Invia Qontor spectrometer. The spectra were collected from 200 to 2000 cm−1 with a 532 nm laser.
High resolution transmission electron microscopy (HRTEM) was performed on an FEI Tecnai F30 instrument. The sample was dispersed in ethanol and the suspended solution was dropped on a copper grid before imaging.
X-ray photoelectron spectroscopy (XPS) analysis was performed by a spectrometer (Model Max 200, Leybold, Germany) using Al Kα radiation as the source of excitation.
Energy dispersive spectroscopy (EDS) mapping and scanning transmission electron microscopy (STEM) images were obtained on an F200 field emission transmission electron microscope with a voltage of 200 kV.
The 57Fe Mössbauer spectra was measured on a Topologic 500A spectrometer at room temperature. The emission source was 57Co (Rh) constant-motion acceleration mode, and the spectra were analyzed using a Lorentzian line shape for fold fitting.
The CO2 hydrogenation reaction was carried out in a fixed bed reactor. 0.2 g of the catalyst as well as 0.5 g of quartz sand were loaded. Before testing, the catalyst was pre-carburized in a pure CO stream (15 ml/min) at 350°C and ambient pressure for 1 h. The gas was then switched from CO to N2 for purging. After the temperature was lowered to 300°C, the reaction gas (T = 300°C, p = 3 MPa, CO2:H2:N2=21:63:16, 6000 ml h−1·gcat−1) was fed. The products were analyzed using an on-line gas chromatograph, model Agilent 7890B, with a thermal conductivity detector (TCD) for N2, CO, CO2 and CH4, and a flame ionization detector (FID) for hydrocarbon and alcohol products.
CO2 conversion rate and product selectivity are calculated by the following Eqs. 1–9:
The CH represents all hydrocarbon products. The
The XRD pattern of the passivated MnCuK-FeC catalyst (Supplementary Figure S1A) shows the characteristic reflections of Fe3O4 (JCPDS, no. 19–0629) at 35.3°, 42.9°, 56.9°, and 62.4°. The XRD and 57Fe Mössbauer spectra (Figure 1A and Table 1A) indicated that the catalyst composition was mainly Fe3O4. The XPS results in Supplementary Figure S2 show that the catalyst surface contains Fe3O4, MnO, CuO, but only Fe3O4 was observed in the XRD results, possibly owing to the high dispersion of Mn and Cu (Supplementary Figure S10A) (Xu et al., 2020b) The XRD pattern of CuZnAlZr catalyst is shown in Supplementary Figure S1B and the catalyst showed characteristic diffraction peaks of CuO at 35.3°, 38.8° and 48.1°, but no other species was observed, consistent with the high content of Cu in the catalyst. Therefore, in order to further investigate the composition of the two catalysts, FT-IR and Raman tests were performed to determine the composition of the two catalysts, and the results obtained were compared with those in the literature. The results are shown in Supplementary Figures S4 and S5. The MnCuK-FeC catalyst shows signals of Fe5C2, MnO and CuO in the Raman spectra, while the CuZnAlZr catalyst shows signals of the four metal oxides (CuO, ZnO, Al2O3, ZrO2), which corresponds to the XPS results in Supplementary Figure S3 (Zhuo et al., 2008; Ahmed et al., 2013; Liu et al., 2014a; Bauer, 2018; Mironova-Ulmane et al., 2018; Lee et al., 2021) Besides, the FT-IR results also show the chemical bonds contained in the two catalysts (Zheng et al., 2013; Jayarambabu, 2014; Elango et al., 2017; Naayi et al., 2018; Chang et al., 2022)
The passivated MnCuK-FeC catalyst was pretreated by CO prior to the catalytic reactions, and in-situ XRD was performed to track the structural evolution (Figure 2A). Under CO treatment, the reflections of Fe3O4 rapidly disappeared at 350°C and the reflections of Fe5C2 became more intense, suggesting the transition from Fe3O4 to Fe5C2. The formation of Fe5C2 is corroborated by the result of the spent MnCuK-FeC catalyst Mössbauer spectra (Table 1B). Meanwhile, the CuO in the catalyst was also reduced, the reflections of metallic Cu appeared at 43.3°, 50.5° and 73.9° after the temperature raised to 250 °C. The reflections of Fe3O4 disappeared after 60 min of CO treatment at 350°C, suggesting a full carburization. For CuZnAlZr (Figure 2B), the reflections of CuO disappeared rapidly at 250°C under CO atmosphere, and the diffractions of metallic Cu appeared, indicating that CuO in CuZnAlZr catalyst was quickly reduced to Cu0. These results are consistent with the CO in-situ XRD results of MnCuK-FeC/CuZnAlZr mixed catalyst (Figure 3), which showed reflections of metallic Cu after the CO pretreatment.
FIGURE 2. In-situ XRD patterns during CO treatment of (A) MnCuK-FeC catalyst and (B) CuZnAlZr catalyst.
We first investigated the catalytic performance of the unmodified iron carbide (XRD result in Supplementary Figure S6). As shown in our previous work, we can see that the unmodified iron carbide showed the high hydrocarbon selectivity (94.2%) and low CO selectivity (5.6%) (Huang et al., 2022), with almost no alcohols in the products (Table 2). This indicates that the catalyst has a strong capacity on the production of hydrocarbons, while the non-dissociative activation of CO is poor and the insertion of CO into the carbon chain is restricted to form HAs. Therefore, we added Mn and Cu to iron carbide to enhance its non-dissociative activation of CO and promote the formation of HAs (Kangvansura et al., 2016; Xu et al., 2020b) Besides, alkali promoter K was introduced to increase the C/H ratio on the catalyst surface to inhibit deep hydrogenation (Iranmahboob et al., 2003; Cosultchi et al., 2012; Liu et al., 2014b) As shown in Figure 4, no desorption peak of CO2 was observed in the MnCu-FeC, while a strong desorption peak appeared between 500°C and 650°C after the addition of 1 wt% K. This indicates that an increased adsorption of CO2 on the catalyst surface, inhibiting the deep hydrogenation of CH products. And it is reported that the unsaturated carbon chains were more conducive to the insertion of CO, thus accelerating the formation of HAs (Tienthao et al., 2007; Christensen et al., 2009) Therefore, we added Mn, Cu, and K to the iron carbide with an appropriate ratio, which was used to enhance the non-dissociated CO activation and inhibit deep hydrogenation of the catalyst. The MnCuK-modified iron carbide catalyst was evaluated for CO2 hydrogenation. It was found that the product distribution exhibits a significant change. More HAs (13.5% in total products) were generated while the proportion of propanol and butanol reached 35% in the alcohols (Table 2). This result is consistent with our expectation that the collective addition of MnCuK into iron carbide can substantially promote the non-dissociative activation of CO and favor the generation of oxygenated compounds, especially HAs products.
Next, we tested the single CuZnAlZr catalyst for CO2 hydrogenation, and the results showed that the CuZnAlZr catalyst exhibited high reverse water gas shift (RWGS) performance, which is consistent with the similar results reported in the literature (Lin et al., 2019) In this work, Mn, Cu and K were used to modify iron carbide and enhance its ability to produce more HAs. A CuZnAlZr catalyst was prepared and coupled with Mn-Cu-K modified iron carbide for CO2 hydrogenation. By testing different catalyst filling methods and filling mass ratios, it was found that the catalysts performed best when mixed with 1:1 powder, and the selectivity of HAs in the total product reached 15.5%
We investigated the proximity of MnCuK-FeC and CuZnAlZr by comparing different catalyst loading methods. The mass ratio of MnCuK-FeC and CuZnAlZr was fixed at 1:1. As shown in Figures 5A,B, for the dual-bed configuration (models a and b), the conversion of CO2 was 38.5% and 38.9%, and the selectivity of HAs was 13.0% and 10.5%, respectively (Table 2), suggesting that different catalyst loading methods have a significant effect on the selectivity of HAs. Therefore, we loaded the two catalysts with a powder mixture, and the results in Table 2 show that powder mixing-filling method (Figure 5C) can further improve the selectivity of HAs from 13.5% (MnCuK-FeC alone) to 15.5%.Table 3
FIGURE 5. Results of CO2 hydrogenation performed on different catalyst filling methods. (A) CO2 conversion and selectivity of CH, MeOH, HAs and CO. (B) Selective distribution of different alcohol products. (The mass ratio of MnCuK-FeC and CuZnAlZr in different filling methods is 1:1) Reaction conditions: 3 MPa, 300°C, 6000 ml h−1·gcat−1, H2:CO2 = 3:1.
The in-situ DRIFT results in Figure 6 show that on the MnCuK-FeC and CuZnAlZr tandem catalyst, the intensity of the *C2H5O species located at 2925 cm−1 and 2852 cm−1 is stronger than that on the single catalysts. This implies that mixing the two catalyst powders facilitates the formation of *C2H5O during the hydrogenation of CO2, indicating that the reaction process has a tendency to form long-chain oxygen-containing compounds (Lin et al., 2019; Xu et al., 2021) Therefore, we can conclude that by mixing the particles of MnCuK-FeC and CuZnAlZr to shorten the distance between the two components, the formation of long-chain oxygenated intermediates can be enhanced, thereby promoting the production of more HAs. Besides, the mixed catalyst of MnCuK-FeC and CuZnAlZr was able to maintain the stable Fe5C2 and metallic Cu phase states during 5 h on stream, as concluded from the in-situ XRD patterns (Figure 7). Both catalysts were able to maintain phase stability when tested individually, with MnCuK-FeC maintaining Fe5C2 and metallic Cu and CuZnAlZr maintaining the metallic Cu phase state (Supplementary Figure S7 and S8).
FIGURE 6. In-situ DRIFT spectra of CO2+H2 reaction over (A) MnCuK-FeC. (B) CuZnAlZr. (C) MnCuK-FeC/CuZnAlZr. (D) Comparison of the three catalysts after 30min reaction. (Reaction conditions: 15.0 ml/min H2+5.0 ml/min CO2, 1.0 MPa, 300°C).
FIGURE 7. In-situ XRD patterns during reaction process of the MnCuK-FeC/CuZnAlZr catalyst. (1:1 powder mixing and filling).
It is also worth noting that no significant deactivation of the mixed catalyst was observed during 80 h of continuous reaction for the CO2 hydrogenation, as shown in Figure 8.
FIGURE 8. CO2 conversion and product selectivity of MnCuK-FeC/CuZnAlZr in CO2 hydrogenation during 80 h reaction. (Reaction Condition: H2:CO2=3:1, T = 300°C, p = 3 MPa, GHSV=6000 ml h−1·gcat−1).
In summary, we successfully prepared multifunctional catalysts composed of Mn, Cu, and K modified iron carbide and CuZnAlZr, which showed excellent activity and stability in the preparation of HAs by CO2 hydrogenation. Meanwhile, the catalysts were evaluated by different loading methods and catalyst filling mass ratios, and it was found that the best performance was obtained by mixing the two components in a 1:1 mass ratio powder, and the selectivity of HAs in the product was 15.5%, which was a relatively significant improvement (7.6%–13%) compared to the layered loading and other mass ratios of catalyst combinations. In addition, the selectivity of propanol and butanol was about 40% of the HAs. The synergistic effect between the Mn-Cu-K modified iron carbide and CuZnAlZr fractions on the synthesis of HAs may result from the enhanced formation of *C2H5O intermediates and their further hydrogenation to form HAs. This work provides a promising approach for the preparation of multifunctional catalysts for more selective HAs formation via CO2 hydrogenation.
The original contributions presented in the study are included in the article/Supplementary Material, further inquiries can be directed to the corresponding authors.
JH performed the experimental design, samples preparation and testing and manuscript writing, JZ, MW, and FD revised the article, GZ reviewed and supervised the manuscript, CS and WX reviewed, edited, supervised the work, and obtained funding. All authors contributed to the article and approved the submitted version.
This work was financially supported by the Major Science and Technology Special Project of Xinjiang Uygur Autonomous Region (2022A01002-1), the Fundamental Research Funds for the Central Universities (DUT22LAB602), the Liaoning Revitalization Talent Program (XLYC2008032) and the CUHK Research Startup Fund (No.#4930981).
We acknowledge the Center for Advanced Mössbauer Spectroscopy, Mössbauer Effect Data Center, Dalian Institute of Chemical Physics, CAS, for providing the Mössbauer measurement and analysis.
The authors declare that the research was conducted in the absence of any commercial or financial relationships that could be construed as a potential conflict of interest.
All claims expressed in this article are solely those of the authors and do not necessarily represent those of their affiliated organizations, or those of the publisher, the editors and the reviewers. Any product that may be evaluated in this article, or claim that may be made by its manufacturer, is not guaranteed or endorsed by the publisher.
The Supplementary Material for this article can be found online at: https://www.frontiersin.org/articles/10.3389/fenrg.2022.995800/full#supplementary-material
Ahmed, M., Rüsing, M., Berth, G., Lischka, K., and Pawlis, A. (2013). CuO and Co3O4 nanoparticles: Synthesis, characterizations, and Raman spectroscopy. J. Nanomater. 2013, 714853. doi:10.1155/2013/714853
Ao, M., Pham, G. H., Sunarso, J., Tade, M. O., and Liu, S. (2018). Active centers of catalysts for higher alcohol synthesis from syngas: A review. ACS Catal. 8, 7025–7050. doi:10.1021/acscatal.8b01391
Behrens, M., Studt, F., Kasatkin, I., Kuhl, S., Havecker, M., Abild-Pedersen, F., et al. (2012). The active site of methanol synthesis over Cu/ZnO/Al2O3 industrial catalysts. Science 336, 893–897. doi:10.1126/science.1219831
Cao, A., Schumann, J., Wang, T., Zhang, L., Xiao, J., Bothra, P., et al. (2018). Mechanistic insights into the synthesis of higher alcohols from syngas on CuCo alloys. ACS Catal. 8, 10148–10155. doi:10.1021/acscatal.8b01596
Chang, H., Lin, Q., Cheng, M., Zhang, K., Feng, B., Chai, J., et al. (2022). Effects of potassium loading over iron–silica interaction, phase evolution and catalytic behavior of precipitated iron-based catalysts for fischer-tropsch synthesis. Catalysts 12, 916. doi:10.3390/catal12080916
Christensen, J. M., Mortensen, P. M., Trane, R., Jensen, P. A., and Jensen, A. D. (2009). Effects of H2S and process conditions in the synthesis of mixed alcohols from syngas over alkali promoted cobalt-molybdenum sulfide. Appl. Catal. A General 366, 29–43. doi:10.1016/j.apcata.2009.06.034
Cosultchi, A., Pérez-Luna, M., Morales-Serna, J. A., and Salmón, M. (2012). Characterization of modified fischer–tropsch catalysts promoted with alkaline metals for higher alcohol synthesis. Catal. Lett. 142, 368–377. doi:10.1007/s10562-012-0765-9
Elango, M., Deepa, M., Subramanian, R., and Mohamed Musthafa, A. (2017). Synthesis, characterization, and antibacterial activity of polyindole/Ag–CuO nanocomposites by reflux condensation method. Polymer-Plastics Technol. Eng. 57, 1440–1451. doi:10.1080/03602559.2017.1410832
Gao, P., Zhang, L., Li, S., Zhou, Z., and Sun, Y. (2020). Novel heterogeneous catalysts for CO2 hydrogenation to liquid fuels. ACS Cent. Sci. 6, 1657–1670. doi:10.1021/acscentsci.0c00976
Guo, H., Li, S., Peng, F., Zhang, H., Xiong, L., Huang, C., et al. (2014). Roles investigation of promoters in K/Cu–Zn catalyst and higher alcohols synthesis from CO2 hydrogenation over a novel two-stage bed catalyst combination system. Catal. Lett. 145, 620–630. doi:10.1007/s10562-014-1446-7
Huang, J., Zhang, G., Zhu, J., Wang, M., Ding, F., Song, C., et al. (2022). Boosting the production of higher alcohols from CO2 and H2 over Mn- and K-modified iron carbide. Ind. Eng. Chem. Res. 61, 7266–7274. doi:10.1021/acs.iecr.2c00720
Iranmahboob, J., Toghiani, H., and Hill, D. O. (2003). Dispersion of alkali on the surface of Co-MoS2/clay catalyst: A comparison of K and Cs as a promoter for synthesis of alcohol. Appl. Catal. A General 247, 207–218. doi:10.1016/s0926-860x(03)00092-9
Jayarambabu, N. (2014). Germination and growth characteristics of mungbean seeds (vigna radiata L.) affected by synthesized zinc oxide nanoparticles. Int. J. Curr. Eng. Technol. 4, 5.
Kangvansura, P., Chew, L. M., Kongmark, C., Santawaja, P., Ruland, H., Xia, W., et al. (2017). Effects of potassium and manganese promoters on nitrogen-doped carbon nanotube-supported iron catalysts for CO2 hydrogenation. Engineering 3, 385–392. doi:10.1016/j.eng.2017.03.013
Kangvansura, P., Chew, L. M., Saengsui, W., Santawaja, P., Poo-arporn, Y., Muhler, M., et al. (2016). Product distribution of CO2 hydrogenation by K- and Mn-promoted Fe catalysts supported on N -functionalized carbon nanotubes. Catal. Today 275, 59–65. doi:10.1016/j.cattod.2016.02.045
Lee, J. H., Lee, H.-K., Kim, K., Rhim, G. B., Youn, M. H., Jeong, H., et al. (2021). Unravelling the K-promotion effect in highly active and stable Fe5C2 nanoparticles for catalytic linear α-olefin production. Mat. Adv. 2, 1050–1058. doi:10.1039/d0ma00920b
Li, S., Guo, H., Luo, C., Zhang, H., Xiong, L., Chen, X., et al. (2013). Effect of iron promoter on structure and performance of K/Cu–Zn catalyst for higher alcohols synthesis from CO2 hydrogenation. Catal. Lett. 143, 345–355. doi:10.1007/s10562-013-0977-7
Liang, B., Ma, J., Su, X., Yang, C., Duan, H., Zhou, H., et al. (2019). Investigation on deactivation of Cu/ZnO/Al2O3 catalyst for CO2 hydrogenation to methanol. Ind. Eng. Chem. Res. 58, 9030–9037. doi:10.1021/acs.iecr.9b01546
Lin, T., Qi, X., Wang, X., Xia, L., Wang, C., Yu, F., et al. (2019). Direct production of higher oxygenates by syngas conversion over a multifunctional catalyst. Angew. Chem. Int. Ed. Engl. 58, 4675–4679. doi:10.1002/ange.201814611
Liu, J., Sun, Y., Jiang, X., Zhang, A., Song, C., and Guo, X. (2018). Pyrolyzing ZIF-8 to N-doped porous carbon facilitated by iron and potassium for CO2 hydrogenation to value-added hydrocarbons. J. CO2 Util. 25, 120–127. doi:10.1016/j.jcou.2018.03.015
Liu, J., Zhang, A., Jiang, X., Zhang, G., Sun, Y., Liu, M., et al. (2019). Overcoating the surface of Fe-based catalyst with ZnO and nitrogen-doped carbon toward high selectivity of light olefins in CO2 hydrogenation. Ind. Eng. Chem. Res. 58, 4017–4023. doi:10.1021/acs.iecr.8b05478
Liu, J., Zhang, G., Jiang, X., Wang, J., Song, C., and Guo, X. (2021). Insight into the role of Fe5C2 in CO2 catalytic hydrogenation to hydrocarbons. Catal. Today 371, 162–170. doi:10.1016/j.cattod.2020.07.032
Liu, X., Cao, C., Tian, P., Zhu, M., Zhang, Y., Xu, J., et al. (2020). Resolving CO2 activation and hydrogenation pathways over iron carbides from DFT investigation. J. CO2 Util. 38, 10–15. doi:10.1016/j.jcou.2019.12.014
Liu, Y., Cheng, B., Wang, K. K., Ling, G. P., Cai, J., Song, C. L., et al. (2014). Study of Raman spectra for γ-Al2O3 models by using first-principles method. Solid State Commun. 178, 16–22. doi:10.1016/j.ssc.2013.09.030
Liu, Y., Murata, K., and Inaba, M. (2014). Synthesis of mixed alcohols from synthesis gas over alkali and Fischer–Tropsch metals modified MoS2/Al2O3-montmorillonite catalysts. Reac. Kinet. Mech. Cat. 113, 187–200. doi:10.1007/s11144-014-0725-z
Luk, H. T., Mondelli, C., Ferre, D. C., Stewart, J. A., and Perez-Ramirez, J. (2017). Status and prospects in higher alcohols synthesis from syngas. Chem. Soc. Rev. 46, 1358–1426. doi:10.1039/C6CS00324A
Mironova-Ulmane, N., Kuzmin, A., Skvortsova, V., Chikvaidze, G., Sildos, I., Grabis, J., et al. (2018). Synthesis and vibration spectroscopy of nano-sized manganese oxides. Acta Phys. Pol. A 133, 1013–1016. doi:10.12693/aphyspola.133.1013
Naayi, S., Hassan, A., and Salim, E. (2018). FTIR and X-ray diffraction analysis of Al2O3 nanostructured thin film prepared at low temperature using spray pyrolysis method. Int. J. Nanoelectron. Mater. 11, 1–6.
Nie, X., Li, W., Jiang, X., Guo, X., and Song, C. (2019). Recent advances in catalytic CO2 hydrogenation to alcohols and hydrocarbons. Adv. Catal. 65, 121–233. doi:10.1016/bs.acat.2019.10.002
Ning, X., An, Z., and He, J. (2016). Remarkably efficient CoGa catalyst with uniformly dispersed and trapped structure for ethanol and higher alcohol synthesis from syngas. J. Catal. 340, 236–247. doi:10.1016/j.jcat.2016.05.014
Portillo Crespo, M. A., Villanueva Perales, A. L., Vidal-Barrero, F., and Campoy, M. (2015). Effects of methanol co-feeding in ethanol synthesis from syngas using alkali-doped MoS2 catalysts. Fuel Process. Technol. 134, 270–274. doi:10.1016/j.fuproc.2015.02.006
Tada, S., Oshima, K., Noda, Y., Kikuchi, R., Sohmiya, M., Honma, T., et al. (2019). Effects of Cu precursor types on the catalytic activity of Cu/ZrO2 toward methanol synthesis via CO2 hydrogenation. Ind. Eng. Chem. Res. 58, 19434–19445. doi:10.1021/acs.iecr.9b03627
Temvuttirojn, C., Poo-arporn, Y., Chanlek, N., Cheng, C. K., Chong, C. C., Limtrakul, J., et al. (2020). Role of calcination temperatures of ZrO2 support on methanol synthesis from CO2 hydrogenation at high reaction temperatures over ZnOx/ZrO2 catalysts. Ind. Eng. Chem. Res. 59, 5525–5535. doi:10.1021/acs.iecr.9b05691
Tienthao, N., Hassanzahediniaki, M., Alamdari, H., and Kaliaguine, S. (2007). Effect of alkali additives over nanocrystalline Co–Cu-based perovskites as catalysts for higher-alcohol synthesis. J. Catal. 245, 348–357. doi:10.1016/j.jcat.2006.10.026
Wang, Y., Wang, K., Zhang, B., Peng, X., Gao, X., Yang, G., et al. (2021). Direct conversion of CO2 to ethanol boosted by intimacy-sensitive multifunctional catalysts. ACS Catal. 11, 11742–11753. doi:10.1021/acscatal.1c01504
Wu, Q., Shen, C., Rui, N., Sun, K., and Liu, C. J. (2021). Experimental and theoretical studies of CO2 hydrogenation to methanol on Ru/In2O3. J. CO2 Util. 53, 101720. doi:10.1016/j.jcou.2021.101720
Xu, D., Ding, M., Hong, X., and Liu, G. (2020). Mechanistic aspects of the role of K promotion on Cu–Fe-based catalysts for higher alcohol synthesis from CO2 hydrogenation. ACS Catal. 10, 14516–14526. doi:10.1021/acscatal.0c03575
Xu, D., Ding, M., Hong, X., Liu, G., and Tsang, S. C. E. (2020). Selective C2+ alcohol synthesis from direct CO2 hydrogenation over a Cs-promoted Cu-Fe-Zn catalyst. ACS Catal. 10, 5250–5260. doi:10.1021/acscatal.0c01184
Xu, D., Yang, H., Hong, X., Liu, G., and Edman Tsang, S. C. (2021). Tandem catalysis of direct CO2 hydrogenation to higher alcohols. ACS Catal. 11, 8978–8984. doi:10.1021/acscatal.1c01610
Yang, C., Liu, S., Wang, Y., Song, J., Wang, G., Wang, S., et al. (2019). The interplay between structure and product selectivity of CO2 hydrogenation. Angew. Chem. Int. Ed. Engl. 58, 11242–11247. doi:10.1002/anie.201904649
Yang, Y., Lin, T., Qi, X., Yu, F., An, Y., Li, Z., et al. (2018). Direct synthesis of long-chain alcohols from syngas over CoMn catalysts. Appl. Catal. A General 549, 179–187. doi:10.1016/j.apcata.2017.09.037
Zeng, F., Mebrahtu, C., Xi, X., Liao, L., Ren, J., Xie, J., et al. (2021). Catalysts design for higher alcohols synthesis by CO2 hydrogenation: Trends and future perspectives. Appl. Catal. B 2021, 291. doi:10.1016/j.apcatb.2021.120073
Zhang, F., Zhou, W., Xiong, X., Wang, Y., Cheng, K., Kang, J., et al. (2021). Selective hydrogenation of CO2 to ethanol over sodium-modified rhodium nanoparticles embedded in zeolite silicalite-1. J. Phys. Chem. C 125, 24429–24439. doi:10.1021/acs.jpcc.1c07862
Zhao, B., Liu, Y., Zhu, Z., Guo, H., and Ma, X. (2018). Highly selective conversion of CO2 into ethanol on Cu/ZnO/Al2O3 catalyst with the assistance of plasma. J. CO2 Util. 24, 34–39. doi:10.1016/j.jcou.2017.10.013
Zhao, Z., Lu, W., Yang, R., Zhu, H., Dong, W., Sun, F., et al. (2017). Insight into the formation of Co@Co2C catalysts for direct synthesis of higher alcohols and olefins from syngas. ACS Catal. 8, 228–241. doi:10.1021/acscatal.7b02403
Zheng, M., Zhang, H., Gong, X., Xu, R., Xiao, Y., Dong, H., et al. (2013). A simple additive-free approach for the synthesis of uniform manganese monoxide nanorods with large specific surface area. Nanoscale Res. Lett. 8, 166. doi:10.1186/1556-276x-8-166
Zhu, J., Wang, P., Zhang, X., Zhang, G., Li, R., Li, W., et al. (2022). Dynamic structural evolution of iron catalysts involving competitive oxidation and carburization during CO2 hydrogenation. Sci. Adv. 8, eabm3629. doi:10.1126/sciadv.abm3629
Zhu, J., Zhang, G., Li, W., Zhang, X., Ding, F., Song, C., et al. (2020). Deconvolution of the particle size effect on CO2 hydrogenation over iron-based catalysts. ACS Catal. 10, 7424–7433. doi:10.1021/acscatal.0c01526
Keywords: CO2 hydrogenation, higher alcohols, tandem catalyst, modified iron carbide, CuZnAlZr catalyst
Citation: Huang J, Zhang G, Wang M, Zhu J, Ding F, Song C and Guo X (2023) The synthesis of higher alcohols from CO2 hydrogenation over Mn-Cu-K modified Fe5C2 and CuZnAlZr tandem catalysts. Front. Energy Res. 10:995800. doi: 10.3389/fenrg.2022.995800
Received: 16 July 2022; Accepted: 09 November 2022;
Published: 17 January 2023.
Edited by:
David J. Heldebrant, Pacific Northwest National Laboratory (DOE), United StatesCopyright © 2023 Huang, Zhang, Wang, Zhu, Ding, Song and Guo. This is an open-access article distributed under the terms of the Creative Commons Attribution License (CC BY). The use, distribution or reproduction in other forums is permitted, provided the original author(s) and the copyright owner(s) are credited and that the original publication in this journal is cited, in accordance with accepted academic practice. No use, distribution or reproduction is permitted which does not comply with these terms.
*Correspondence: Chunshan Song, Y2h1bnNoYW5zb25nQGN1aGsuZWR1Lmhr; Xinwen Guo, Z3VveHdAZGx1dC5lZHUuY24=
Disclaimer: All claims expressed in this article are solely those of the authors and do not necessarily represent those of their affiliated organizations, or those of the publisher, the editors and the reviewers. Any product that may be evaluated in this article or claim that may be made by its manufacturer is not guaranteed or endorsed by the publisher.
Research integrity at Frontiers
Learn more about the work of our research integrity team to safeguard the quality of each article we publish.