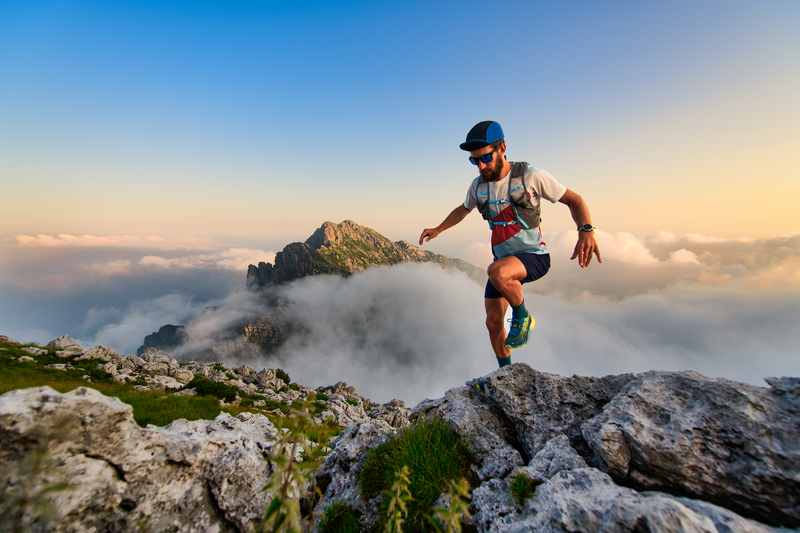
95% of researchers rate our articles as excellent or good
Learn more about the work of our research integrity team to safeguard the quality of each article we publish.
Find out more
REVIEW article
Front. Energy Res. , 16 September 2022
Sec. Advanced Clean Fuel Technologies
Volume 10 - 2022 | https://doi.org/10.3389/fenrg.2022.971704
This article is part of the Research Topic Advances in Process Modeling and Optimization of Clean Energy Processes View all 7 articles
Battery Energy Storage Systems (BESS) are essential for increasing distribution network performance. Appropriate location, size, and operation of BESS can improve overall network performance. The appropriately scaled and installed BESS helps meet peak energy demand, improve the advantages of integrating renewable and distributed energy sources, improve power quality control, and lower the cost of expanding or re-configuring the distribution networks. This paper investigates the feasibility of BESS for providing short-term and long-term ancillary services in power distribution grids by reviewing the developments and limitations in the last decade (2010–2022). The short-term ancillary services are reviewed for voltage support, frequency regulation, and black start. The long-term ancillary services are reviewed for peak shaving, congestion relief, and power smoothing. Reviewing short-term ancillary services provides renewable energy operators and researchers with a vast range of recent BESS-based methodologies for fast response services to distribution grids. Long-term ancillary services will provide the distributed network system operators and researchers with current BESS-based bulk-energy methods to improve network reliability and power quality and maximize revenue from renewable energy generation. The review presents a list of energy storage policies and BESS projects worldwide with a cost-benefit analysis. The challenges for deploying BESS in distribution grids recommended solutions for the implementation challenges, and future research directions are also presented.
Large-scale power plants are traditionally used to provide ancillary services to maintain stable operation of the distribution networks Islam et al. (2017b); Prakash et al. (2020); Islam et al. (2017a). However, the recent increase in renewable energy sources (RESs) has affected the operational schemes of the power grids. The intermittent operation of RESs increases the uncertainties in existing grids and creates technical and operational challenges Xu et al. (2021). The high level of penetration, primarily in the transmission grids, can significantly alter the bulk power system due to intermittent generation and can affect the demand and generation balance, resulting in unusual frequency variations Wang et al. (2017). On the other hand, the active power injections from distributed RESs at the distribution grid can lead to technical issues such as voltage violations, power fluctuations, and network congestion Nour et al. (2019). The traditional power plants are gradually decommissioning due to the increasing penetration of centralized and distributed RESs, which reduces the overall capacity of conventional power plants for ancillary service provision Kryonidis et al. (2021); Podder et al. (2020).
Energy storage systems are capable of providing a variety of distributed auxiliary services and serving as a backup power supply. The integration of BESS in active distribution networks has been encouraged due to the rising penetration of RESs and decommissioning of traditional power pants Kumar et al. (2020a, 2020b). The BESS market, much of which is related to the grid and commercial resilience, is described as 1) ancillary services: short bursts of electricity are provided or absorbed to maintain supply and demand, ensure grid stability (voltage stability), frequency regulation and reserves; 2) peaking capacity: provision of sufficient capacity to satisfy the system’s peak demand; 3) energy shifting: increasing system flexibility needs drive uptake. Energy storage is charged during low costs and released when demand exceeds supply. Batteries may be charged using excess renewable energy or assets that become dispatchable when combined with the battery. 4) Transmission and distribution-level: employing ESS as an alternative to traditional network reinforcement, such as to meet an incremental increase in network capacity instead of an expensive line upgrades.
BESS can accommodate different batteries, such as lithium-ion, lead-acid, and nickel-cadmium. The key benefits and drawbacks of common BESS currently employed in power systems applications are presented in Table 1. Besides, each battery type has technical parameters that identify BESS applications and impact battery energy storage efficiency. The main properties of a battery are its storage capacity, power attribute, round-trip efficiency, depth-of-discharge (DoD), and lifetime. The storage capability defines the quantity of electricity accessible in a BESS or the amount of electric charge stored in a battery, power attribute specifies how much power a battery can supply or how much power a BESS can deliver, round-trip efficiency describes the ratio of energy delivered by a battery (during discharge) to the energy given during a charge cycle, depth-of-discharge (DoD) indicates the percentage of energy discharged from a battery relative to its total capacity whereas lifetime, which is defined as the number of charge and discharge cycles of a battery or the amount of energy that a battery can supply during its lifetime (battery throughput) and safety, shows the battery’s compliance with safety requirements. While certain BESS technologies may be reliable and mature IRENA (2015a), with further cost reductions anticipated IRENA (2015b), economic concerns are still preventing BESS from becoming a mainstream solution for ancillary services in power grids Olatomiwa et al. (2016). Inappropriate dispatch strategy for BESS can also lead to instability issues, speedy degradation, and uneconomic operation of power grids Olatomiwa et al. (2016). BESS planning and operation are the key to an effective and efficient solution for grid ancillary support Jayasekara et al. (2015); Wang et al. (2018); Divshali and Söder (2017); Hashemi and Østergaard (2016); Wang L. et al. (2015); Zeraati et al. (2016); Marra et al. (2014); Tan et al. (2020); Hemmati et al. (2017); Hu et al. (2014); Nair et al. (2020).
There are various review papers that have discussed BESS, as shown in Table 2. For example, a review of the methods and applications for battery sizing was presented in Yang et al. (2018). The review provides a valuable contribution to the literature as it clusters battery sizing based on renewable energy sources, making it clear to identify critical metrics and select the most appropriate methods for battery sizing based on various renewable energy applications. However, the review does not cover the applications of BESS for ancillary services in the distribution grids. An overview of the energy storage systems (ESS) in terms of placement, sizing, operation and power quality was presented in Das et al. (2018). The study does not cluster different ancillary service applications of BESS, instead focuses on the ESS placement, sizing, operation and power quality areas. A study of BESS in the United Kingdom (United Kingdom) was presented in Mexis and Todeschini (2020), which describes the BESS-based projects in the United Kingdom and different BESS technologies. The ancillary services provided by BESS were briefly explained. A more extensive description of the common ancillary services specific to the BESS in distribution grids was not provided. The review also does not cover current challenges related to BESS deployment. A comprehensive review of BESS integration in distribution grids was presented in Stecca et al. (2020), which describes the different aspects of integrating BESS in distribution grids. The study was divided into three sections to explain the different BESS technologies, functionalities, sizing, location, and control of grid-connected BESS in distribution systems. However, it does not provide challenges related to integrating BESS in distribution grids, and a very brief discussion on the ancillary services was provided.
A review of the state-of-the-art literature on the economic analysis of BESS was presented in Rotella Junior et al. (2021) but did not describe the BESS applications for ancillary support. Optimal BESS sizing, system constraints, optimization model and methodologies, and their benefits and drawbacks were presented in Hannan et al. (2021). BESS provision for ancillary services was not discussed. A review of control mechanisms for smoothing wind power output using battery energy storage systems was presented in de Siqueira and Peng (2021). The study was primarily focused on the power smoothing capabilities of BESS with wind application and did not include other common ancillary services. Another review of the latest technologies, sizing techniques and considerations, efficiency, cost, and recycling perspectives of BESS was presented in Sufyan et al. (2019). The application of BESS for ancillary services and the existing challenges were only described from the sizing point of view. A review of hybrid-PV BESS was presented in Rana et al. (2022) to describe the methods for lifetime improvement, cost reduction analysis, optimal sizing and control, power quality issues, and peak shaving.
The investigation of the existing review papers shows that various concepts have been covered in the literature, mainly focusing on optimal siting, sizing, and scheduling algorithms, BESS projects, control algorithms for wind power smoothing, and different BESS technologies. None of the existing surveys have presented an extensive review of BESS-based algorithms for distribution grid ancillary support. This paper extensively reviews the latest research and developments on fixed and static BESS-based solutions for distribution network ancillary support to bridge these research gaps. Relevant journal papers have been selected to provide an up-to-date review in the last decade. The review is divided into short-term and long-term ancillary services. The short-term ancillary services for future distribution grids are reviewed for voltage control, frequency regulation, and black start. Long-term ancillary services are for congestion management, peak shaving, and power smoothing. The findings are summarized in a series of tables with detailed information about different grid ancillary services, optimization algorithms, existing methodologies for BESS planning (siting and sizing), and current control strategies for BESS dispatch and their limitations. This review provides a survey of energy storage policies worldwide. Cost-benefit analysis and a list of field demonstration projects related to BESS are presented. Challenges for deploying BESS are also identified, and future research directions are provided. It should be noted that the research issues associated with mobile battery energy storage systems, such as EVs, are different and have not been covered in this paper.
The rest of this paper is organized as follows. The review methodology is described in Section 2. Section 3 provides a review of ancillary services for distribution grids. The energy storage systems policies are described in Section 4. A list of global BESS projects with cost-benefit analysis is provided in Section 5. Section 6 presents the challenges for deploying BESS, while Section 7 concludes the findings and provides future research directions.
The proposed framework for this review is based on the preferred reporting items for systematic reviews and meta-analyses (PRISMA) approach to identify and review the published literature over the last decade (2010–2022). The PRISMA approach, as shown in Figure 1, uses a three-step process of planning, conducting, and reporting to provide a checklist of items that are used to increase the transparency and clarity of reviews Page and Moher (2017). The review questions and the purpose of conducting the study are identified in the planning process. The conducting process helps implement strategies for finding relevant articles and extracting the results. The final reporting process assists in investigating the results from selected papers and delivering the concluding remarks such as current limitations, existing challenges, and potential future research directions. The following subsection describes the planning, conducting, and reporting stages.
In this phase, we identify the critical research areas and keywords for research. Then, the research questions, as shown in Table 3, are formulated. The guideline from Page and Moher (2017) has been used to develop the research questions in this paper.
In this process, we use search engines and digital libraries, such as Scopus, Google Scholar, and Web of Science (WOS), to collect relevant resources for the review. A simple search strategy is established using the Boolean operators, such as “AND” and “OR,” to join the keywords. For instance, our search process is established using “Battery” OR “BESS” AND “Ancillary Services” to indicate that any items falling under the terms “Battery” or “BESS” with “Ancillary Services” should be included in the conducting process. In the first round, 941 research papers are collected from the above-mentioned search engines for this research. These papers are collected based on the research topics, contents, and focuses. Then, in the next round, a filtering process is used to eliminate the irrelevant papers, mainly those that do not fit with the scope of this review or are too old. Only the articles published between the years 2010 and 2022 are selected for this review to provide the recent update on research and developments related to the BESS provision for distribution grid ancillary support. In the final filtration process, the majority of the conference papers are removed. Only top-ranked journal papers and a few relevant conference papers that are prepared with BESS for ancillary support in distribution grids are selected. After the final stage of filtration, 115 papers are selected for investigation. These papers are divided into two major sections; BESS provisions for short-term and long-term ancillary services.
For this review, the findings of collected articles are reported using VOSviewer experiments. Scholarly articles, such as scientific papers, books, or research reports, typically contain millions of raw data, and analyzing them can be time-consuming and challenging. Introducing clustering solutions is one method to solve this problem Ali et al. (2021). Clustering techniques find similar publications or journals by grouping each article and establishing a citation network. VOSviewer, a powerful visualization tool, is used in this work to provide clustering solutions to identify the most common subjects in the sparse literature. The collected articles from the conducting process are used to create a network visualization map, and the results are presented as visual clusters (Figure 2). The size of a cluster, as illustrated in Figure 2, shows the number of articles that belong to that cluster. Cluster-relatedness is represented by colored lines between clusters, with line width denoting the number of citations between clusters. Our analysis has found that “battery energy storage systems” have gained significant attention in the last 12 years. The standard ancillary services provided by battery energy storage systems are categorized into four clusters, as shown in Figure 2. The first cluster includes the research and innovations in voltage regulation support using BESS. The second cluster highlights the articles related to peak shaving and congestion management. The third cluster demonstrates the analysis for power smoothing and power quality improvement in distribution grids, whereas the fourth cluster shows the innovations for frequency support. In the terminology of link strength, the keyword “battery energy storage systems” is the largest, appearing 450 times.
Different terminologies are used to classify the types of ancillary services for distribution grids Malhotra et al. (2016). In this paper, the ancillary services for distribution grids are selected and are presented for short-term and long-term applications, as shown in Figure 3. A review of BESS-based methodologies for providing short-term ancillary services to the distribution grids is presented in the following subsection.
The short-term ancillary services are known as fast response services that are primarily focused on compensating demand and generation unbalance Mexis and Todeschini (2020). BESS can be deployed to improve the grid’s performance by ensuring stable, robust, and reliable grid operation. In this paper, the common short term ancillary services are reviewed for voltage support Hesse et al. (2017), frequency regulation Farhadi and Mohammed (2015) and black-start Akhil et al. (2015).
The traditional power distribution grids were designed considering unidirectional power flow, typically from high voltage or medium voltage transformers to the end-users connected via distribution lines Prakash et al. (2016, 2017a). The recent advents of distributed RESs have caused bidirectional power flow in distribution grids, leading to voltage issues in the network Alyami et al. (2014); Prakash et al. (2019); Mamun et al. (2022); Chand et al. (2019). The intermittent renewable energy connected to the power grids must meet the voltage requirements and standards to guarantee that the nominal grid voltages operate within limits Prakash et al. (2022a). Several solutions have been proposed to overcome voltage issues in distribution networks. Curtailing power to reduce the demand and generation unbalance is a traditional approach for mitigating overvoltage, but it limits the maximum utilization of RES Alyami et al. (2014); Chand et al. (2020a). Providing reactive power support from PV converters Samadi et al. (2014), installing sensors and voltage regulators Chamana and Chowdhury (2018), adjusting on-load tap changers Todorovski (2014) or even re-configuring the grid Shayani and de Oliveira (2010) are other traditional solutions in the existing literature. While the traditional approaches can effectively mitigate voltage problems, they lead to increased network losses, and excessive green energy curtailment Chaudhary and Rizwan (2018); Chand et al. (2020b); Islam et al. (2018).
The recent advancements of BESS and their flexible nature provide a promising and most effective solution for voltage support in active distribution grids Chaudhary and Rizwan (2018). BESS can act as a load and a generator, and can be switched on and off instantaneously. They can be used to consume excess renewable generation during peak generating hours and reduce the overvoltage issues in the network Chaudhary and Rizwan (2018). Similarly, they can act as a generator during peak load hours to reduce the under-voltage issues. A typical example of a BESS application for mitigating overvoltage and under-voltage issues is shown in Figure 4. The generation from photovoltaic units exceeds the load demand between 08:00 to 14:00, creating excessive reverse power flow at the substation and resulting in overvoltage issues. Similarly, between 19:00 to 21:00, the peak load causes voltage issues. As shown in Figure 4, BESS is charged and discharged accordingly to mitigate the overvoltage and under-voltage issues, respectively.
In this paper, the control schemes of BESS for voltage regulation are characterized as centralized, decentralized, and localized. A centralized control schemes adopts a single central control center that gathers the required measurements from the distribution grid (primarily through the smart meters or remote terminals). Then the central controller retrieves the information and responds to the voltage problems by communicating the set-points to the distributed energy resources and voltage control devices Antoniadou-Plytaria et al. (2017). The decentralized controllers gathers local measurements, process them, and provides counteractions to appropriately control the voltage profiles. Decentralised controllers have more flexibility and reliability compared to the centralized controllers as they can be based on zone controllers rather than on single DER controller. This provides flexibility for multiple point of control to regulate voltage issues in distribution grids Antoniadou-Plytaria et al. (2017). Finally, the localized control are mainly the inverter based volt/var controller that creates a closed-loop dynamical system in which the measured voltage influences the reactive power injection to impact the voltage profiles Farivar et al. (2015). A literature survey for centralized, decentralized and localized control is presented as follows.
Considering the centralized control, a method for optimal planning and operation of community BESS to provide voltage support to the distribution network was proposed in Jayasekara et al. (2015). The planning and operation of BESS were done from the system operators’ perspective, assuming that the community BESS is owned and operated by them. The aim of the research was to provide an overview of voltage support using bulk-energy from BESS. As a result, the study was mainly suitable for medium voltage or large-scale systems and did not consider multiple distributed BESS installations. In a practical scenario, multiple BESS could operate at the same time and optimal coordination might be required to maintain smooth and steady operation of the grid Wang Y. et al. (2015). A control strategy to coordinate photo-voltaic (PV) generators and BESS for voltage regulation in medium voltage distribution grids was proposed in Wang et al. (2018). But, the methodology did not provide any economic analysis to demonstrate the benefits/savings for minimizing BESS charging/discharging and SVR tap operations.
Moreover, a novel optimization model for siting and sizing of central BESS in a low voltage distribution network was proposed in Divshali and Söder (2017). The objective function was formulated to achieve voltage regulation and increase the hosting capacity limit of the network. The research only considers steady-state conditions for designing the controller for hosting capacity improvements and does not consider any dynamic studies. Dynamic studies can provide better and more accurate analysis as it will allow to model the complex scenarios and various uncertainties such as transient conditions, real-time distribution grid behavior and batch and semi-batch processes Divshali and Söder (2018). A centralized methodology that mitigates the voltage violations in low voltage distribution grids by combining the support from BESS operation and reactive power of distributed RESs was proposed in Hashemi and Østergaard (2016). The method is highly dependent on the input parameters such as electricity price and grid data (including network topology, line characteristics, and locations of PV), which could be challenging to obtain in a real practical scenario Ali et al. (2020a,b). A method has used a centralized control scheme to coordinate multiple BESS for voltage regulation in a distribution network in Wang L. et al. (2015).
The centralized control for voltage regulation requires uninterruptible communication between central and BESS controllers. The efficiency of the centralized controller is decreased if the communication is interrupted or lost. As a result, the support for regulating voltage problems is not guaranteed Kryonidis et al. (2021). The distributed control does not require global grid information; instead, a communication link between neighboring installations is required Antoniadou-Plytaria et al. (2017). Voltage rise or drop issues were solved using the distributed BESS in Wang Y. et al. (2015). A coordinated control scheme was proposed that comprises distributed and localized controllers. The distributed control has used a consensus algorithm to regulate the voltage issues while the localized control maintains the desired BESS state of charge (SoC). Similar research has used consensus-based control strategies to achieve voltage regulation by ensuring that appropriate BESS SoC is maintained in Zeraati et al. (2016). However, the methodology does not include battery degradation analysis and uses 100 percent charging and discharging efficiency of BESS in most of the investigation, which maybe impractical. According to the research in Wankmüller et al. (2017), battery degradation must be included in the optimization models as they are very crucial for providing realistic estimates of profitability.
Voltage regulation was achieved using distributed control strategy that combines the management of plug-in electric vehicle batteries and curtailment of PV generation in Zeraati et al. (2017). The consensus-based control algorithm ensures that curtailment of PV generation is only applied when the plug-in electric vehicles cannot regulate voltage issues. A distributed BESS management scheme using reinforcement learning to mitigate overvoltage issues in a PV-rich distribution grids was proposed in Al-Saffar and Musilek (2020). The results shows that the methodology mitigates voltage issues by controlling network-wide installed BESS and minimizes power losses due to power transfer in the network. However, other practical factors such as thermal constraints and unbalanced scenarios of distribution grids were not considered, which did not demonstrate the scalability of the methodology to larger practical distribution grids. Event triggered voltage control techniques to optimize BESS power for voltage regulation in power grids were proposed in Kang et al. (2022) and Zhang et al. (2022).
It is evident from the existing literature that centralized and decentralized controls require reliable communication between storage controllers. Voltage regulation is not guaranteed in case of any communication issues in the distribution network. To eliminate the communication requirement and dependency, localized controls have been proposed in the literature. A simple yet most common local control of residential BESS has used excess PV power to charge the BESS and discharge when PV generation is low or zero in Barcellona et al. (2019). The methodology proposed does not provide battery degradation analysis and considers full discharge of the battery, which is impractical and can affect the battery’s health in real scenarios. Additionally, an optimization model without any battery degradation analysis could lead to an inaccurate estimation of the profitability Wankmüller et al. (2017). A similar control based on voltage sensitivity analysis to mitigate overvoltage issues in the distribution network was proposed in Marra et al. (2014). The signal for BESS charging is triggered by using a predefined PV power threshold. The methodology was further extended to address the under-voltage issues during peak demand in Cortés et al. (2018). Still, BESS support for weak grid dynamics, such as integrating electric vehicles and high penetration of renewable energy sources, were not considered.
Adaptive control to manage the operation of BESS for voltage regulation and congestion management of distribution grids was proposed in Procopiou et al. (2018). A model-driven control algorithm to incorporate BESS for voltage regulation in distribution grids was proposed in Krata and Saha (2018). A similar coordinated control strategy to coordinate BESS operation with the on load tap changers for voltage regulation in distribution feeders was proposed in Tewari et al. (2020). However, the proposed scheme was only tested on the IEEE test cases. The working principle of the methodology on a real distribution grids with various regulation devices were not demonstrated.
According to the IEEE/CIGRE Joint Task Force on Stability Terms, frequency stability is described as “the ability of a power system to maintain steady frequency following a severe system upset resulting in a significant imbalance between generation and load” Kundur et al. (2004). To ensure that the frequency variation is within the specified limits, it is essential to immediately balance any differences between demand and generation Rajan et al. (2021); Chand et al. (2020c). Failure to maintain the frequency within acceptable limits may lead to cascaded outages and blackouts Rajan et al. (2021). BESS has flexible and fast response characteristics that can balance demand and generation by either consuming or producing power based on the network requirements. The following discussion is divided into two parts. First, the research on providing frequency regulation support with BESS is discussed. Then, the innovations and control algorithms related to BESS for multiple purposes, including frequency control, are presented.
A novel approach for optimal BESS sizing to stabilize the frequency during high PV generation hours was proposed in Aghamohammadi and Abdolahinia (2014). The researchers in Wu et al. (2015) have proposed an algorithm to coordinate PV and BESS for frequency regulation. Bus-signaling technique was used to ensure that the BESS is never over or undercharged. Still, the methodology was based on a trade-off between the investment of communication links and the high quality of power supply, which may vary based on different applications. A direct ramp rate control technique to manage the BESS SoC and their support functionality for frequency regulation was used by the research in Bullich-Massagué et al. (2017). However, it does not consider optimal sizing of BESS for frequency regulation. Inappropriate or random BESS size could add to unnecessary costs and lead to technical problems such as creating frequency oscillations, increasing system losses or causing system collapse Kerdphol et al. (2016). Also, the ramp rate control was designed considering a substantial restriction with a time window of 2 s, which can lead to unnecessary power oscillations. A coordinated control strategy using torque limit control and BESS to enhance the temporary inertial response of the wind turbine generators and mitigate the secondary frequency drop issues, respectively was proposed in Wu et al. (2017). The methodology does not consider BESS’s optimal size and location, which could help achieve a trade-off between optimal frequency regulation performance and economic perspectives of BESS. The controller aims to enhance the BESS lifespan by avoiding excessive use, so the BESS is smoothly disconnected once the predefined frequency limit is reached.
A controller for frequency regulation was designed by combining the adaptive droop control and SoC recovery control for BESS in Tan et al. (2020). The research aims to provide a controller that can improve the BESS’s system frequency dynamics and performance. A control algorithm for BESS to participate in primary frequency regulation in power grids was developed in Meng et al. (2021). The methodology was tested on different load disturbances to demonstrate the benefits of virtual inertia control and droop control. A location-dependent control of BESS for fast frequency response services was proposed in Zhao et al. (2021). After comparison with other centralised approaches, it was found that the proposed methodology in Zhao et al. (2021) can provide faster-acting frequency support. However, several uncertainties such as communication delays were not considered in the study, which could be helpful to demonstrate the practical scenarios of power grids.
The application of BESS has been analyzed for multiple purposes instead of only frequency support. A novel control strategy to utilize the maximum reserve capacity of BESS for primary frequency control and self-consumption was presented in Engels et al. (2019). A linear recharging method for controlling the BESS SoC guarantees an adequate BESS energy reserve for self-consumption. A comparison of revenue generated for a single application (frequency regulation) and multiple applications (frequency regulation and self-consumption) of BESS was made. It was found from the obtained results that the BESS for multiple applications increases the revenue by 25%. A similar study presented a framework for maximizing the profit of BESS in Zhang et al. (2016). However, it did not include high ramping effects of BESS in the economic assessment. The ramping effects of BESS have a direct impact on the degradation and, if not analyzed properly, can lead to increased investment costs Rajan et al. (2021).
An optimal BESS planning strategy for frequency control in a Mexican power grid was proposed in Ramírez et al. (2018). The objective function was modeled to site the BESS based on the distributed renewable energy penetration limit and generation contingency. Then, a droop controller was implemented to control the BESS for frequency regulation. Although the methodology provides positive results for regulating the system frequency, the analysis does not consider any limits for BESS SoC, which could result in speedy degradation of BESS. Also, the placement methodology allocates BESS on larger transient frequency deviation buses and is only limited to the primary frequency control application. A novel methodology was used to control a utility scale BESS for primary frequency and local voltage regulation services in Zecchino et al. (2021). A similar study proposed a re-configurable BESS emulation tool for frequency and voltage support in Boles et al. (2019). Unlike other existing emulators, the emulator proposed in Boles et al. (2019) incorporates BESS power electronics and control interface to automate frequency and voltage support services. A study examined and presented the application of BESS for multiple ancillary services, including voltage regulation, congestion relief, demand response, self consumption, energy arbitrage, and frequency regulation in Maeyaert et al. (2020). Some common ancillary services such as power smoothing, peak shaving and black-start were not covered. Optimal planning of BESS was done in Wu et al. (2021) to determine appropriate size of BESS for frequency regulation and energy arbitrage.
The ability of the grid to restore its working state after being shut down due to faults is known as black-start Datta et al. (2021). In a power system grid, any black-start source should have the capacity to self-start, supply the required power to the non-black-start units, and immediately provide support to stabilize grid voltage, and frequency Datta et al. (2021). In the recent literature, BESS is an ideal and widely accepted solution for black-start in power grids due to its voltage source converter-based active and reactive power regulation capabilities.
A two-stage methodology to coordinate multiple BESS for black-start was proposed in Li C. et al. (2020). The first stage partitioned BESS into twenty-four operating modes considering the working partitions of BESS SoC. Adaptive control was then used to manage the BESS charging/discharging power and predefined SoC constraints in the second stage. A methodology to restart the grid using wind power generators was presented in Liu and Liu (2019). To ensure reliable operation of the wind generators and minimize any distribution model mismatch during the black-start process, optimal siting and sizing of BESS was done. An optimization methodology for black-start using PV-BESS was proposed in Li et al. (2019). The optimization process was collectively solved in three layers; the data analysis layer was used to analyze/predict available PV power for black-start, and the coordination layer was used to determine the optimal control quantity of PV units and BESS power. In contrast, the scheduling control layer has determined the actual control process of PV and BESS controllers.
Moreover, the application of BESS for black-start was validated using a real case study of an Italian MV distribution grid in Manganelli et al. (2018). The effectiveness of BESS for black-start in distribution grids was demonstrated using different scenarios; BESS in coordination with distributed generators and BESS alone. However, the study does not consider detailed modeling and experimental activities for investigating transient, voltage, and frequency control. As a result, scalability of the methodology to a practical system cannot be guaranteed. The methodology can only be implemented in a practical system once the distribution system operator can fully control the DG Izadkhast et al. (2022). A similar investigation in Strunck et al. (2019) and Strunck et al. (2021) determined the black-start capability of an actual distribution grid in Germany that has a high share of BESS and combined heat and power (CHP) plants. Still, the dynamic effects and protection devices that have a significant role in system restoration were not considered. A practical wind farm with high-capacity BESS in Hailar was used for black-start in Liu et al. (2016). The effectiveness of the proposed black-start scheme was validated by simulating the actual East Hailar thermal power plant. A controller to evaluate the performance of BESS for providing voltage and frequency support during black-start was designed in Izadkhast et al. (2022). It was found that BESS has full capabilities for stabilizing the voltage profile and regulate the frequency during black-start.
A summary of the BESS-based methodologies for short-term ancillary services is presented in Table 4. The literature is categorized based on the three short-term ancillary services; voltage control, frequency regulation, and black-start. Research contributions, methodologies, and the limitations of each research article are described.
The long-term ancillary services are also known as “bulk energy,” which aims to store and use a large amount of energy to obtain an efficient and economical power system operation Akhil et al. (2015). BESS in transmission and distribution grids are operated over a long period for ancillary support to improve the system’s efficiency and reduce the costs of producing and delivering electricity Mexis and Todeschini (2020). Congestion relief, peak shaving, and power smoothing are reviewed for long-term ancillary services in this paper.
Congestion management in distribution networks refers to reducing the overloading of distribution network equipment. Due to the recent increase in distributed energy resources, overloading in distribution networks has become a major technical issue which leads to stability and security issues, uneconomic operation of the grid, damaging of network equipment, or even collapsing the grid if not mitigated on time Gupta et al. (2017); Prakash et al. (2017b). The traditional solutions for mitigating the congestion of distribution grids include network configuration, utilization of compensating devices, managing on-load tap changers or re-scheduling the loads, and generating units Pillay et al. (2015). The recent literature indicates that BESS if appropriately managed, can be the most promising and efficient solution for managing congestion issues in the distribution grids Kryonidis et al. (2021). This section reviews the most recent BESS-based solutions for congestion management in power distribution grids.
A novel methodology for optimal planning and scheduling of BESS to avoid thermal overload in distribution grids was proposed in Hemmati et al. (2017). The objective function was used to minimize the total power flow in the network, hence reducing congestion, minimizing power losses, enhancing network stability, and improving network reliability. However, the methodology did not consider battery degradation analysis and did not demonstrate the scalability to a larger practical distribution grid. Similar research mitigates voltage and line loading issues in the distribution network in Bahramipanah et al. (2016). A decentralized control algorithm was proposed to manage the distributed BESS where the communication between different areas and regions of the network is achieved using the concept of multi-agents. Low voltage distribution grid congestion was reduced by deploying centralized community BESS in van Westering and Hellendoorn (2020). BESS control was formulated and solved by a linear optimization problem and a linear programming solver.
A coordinated scheme mitigates distribution line and transformer overloading by managing the charging strategy of multiple EVs in Hu et al. (2014), but does not analyze the economic feasibility of utilizing EV batteries for congestion management. Similar charging strategies for roof-top PV and BESS integrated EV charging stations were proposed to avoid transformer overloading issues in de Mattos Affonso and Kezunovic (2018) and Datta et al. (2020). A novel approach for BESS charging/discharging to mitigate uncertainties related to RESs and relieve congestion in power grids was proposed in Prajapati and Mahajan (2021). Although the methodology provides impressive results, optimal planning of BESS is required to demonstrate a practical scenario. The methodology considers assumed BESS sizes, which could be oversized or undersized for the grid. Random or assumed BESS sizes can result in additional costs and lead to technical problems, which could affect the normal operation of the grid Kerdphol et al. (2016).
A model predictive control to determine the optimal dispatch of PV-BESS was presented in Nair et al. (2020). A multi-objective function was used to mitigate the congestion issues, delay the BESS degradation, and improve self-consumption. A methodology for optimal BESS siting, sizing, and dispatch to improve the stability and reliability of a medium voltage distribution grid by relieving congestion was presented in Mohamed et al. (2020).
Peak load occurs only for a small portion of time during the day and does not often coincide with peak generation Uddin et al. (2018). A traditional approach is to install additional generating units for meeting the peak load, known as the capacity addition technique Uddin et al. (2018); Prakash et al. (2022b). This approach is not an economical solution as the distribution system operators need to install and manage additional generating units only for a few hours per day Mishra and Palanisamy (2018). It also leads to increased carbon emissions and speedy degradation of network equipment Mishra et al. (2013). Peak shaving is a process of flattening the load curve by lowering the peak load and transferring to an off-peak period Nourai et al. (2008), which is a preferred solution to overcome the drawbacks of the capacity addition technique. Characteristics of BESS make it a promising solution for peak shaving in power grids where BESS can be charged during the off-peak period (low demand) and discharged during peak-period (high demand) Farsadi̇ et al. (2016). A typical example of BESS application for peak shaving is shown in Figure 5. The BESS is charged during off-peak hours, between 01:00 to 08:00, 12:00 to 15:00, 22:00 to 24:00, and discharged during peak hours (10:00 to 12:00 and 15:00 to 17:00) to flatten the load curve.
In this paper, BESS-based solutions for peak shaving are classified into two categories; planning (siting and sizing) and operation (scheduling), respectively. Deployment of BESS is cost-sensitive, and installing oversized or undersized BESS can lead to several technical issues such as voltage violations, thermal overload, or increased losses in the network Uddin et al. (2018). As a result, planning becomes an essential part of the deployment process. The efficiency and lifetime of the BESS depends how they are dispatched Uddin et al. (2018). Optimum dispatch can help to increase the efficiency of BESS, maximize the revenue and delay the battery degradation. Recent research and innovations related to the aforementioned areas are described as follows.
A novel BESS sizing strategy for peak shaving considering the historical load profiles of customers was proposed in Chua et al. (2016). Adaptive control was used to maximize the peak demand reduction of a commercial building in Malaysia. However, the key input to the methodology is the historical load profiles of residential customers, which can be considered confidential information in a practical scenario Ali et al. (2022b,a, 2020c). Optimal BESS allocation in a typical radial distribution network was done by formulating a multi-objective function, aiming to minimize peak shaving, power losses, and investment costs in Lakshmi and Ganguly (2019). The multi-objective function was modeled using Pareto approximation and solved using the particle swarm optimization (PSO) algorithm. The scalability of the methodology to a practical distribution grid is not guaranteed as the study does not consider any uncertainties in load demands and renewable generations, coordination between inverters, PVs, and BESSs, and unbalanced characteristics of distribution grids. A similar research determined the optimal capacity of BESS to shave the peak load of a building at Naresuan University (NU), Phitsanulok, Thailand in Prasatsap et al. (2017). Still, it does not consider battery degradation analysis which is necessary to demonstrate the effects of battery charging and discharging on their health and loss of life. A novel methodology used the Malaysian tariff to determine optimal BESS capacity for maximizing electricity bill savings and peak shaving of commercial and industrial buildings in Subramani et al. (2018). A three-step coherent strategy to determine the optimal size, location, and operation of BESS for peak shaving using historical load data was proposed in Danish et al. (2020). However, the methodology requires customers’ historical load data as input, which could be confidential and difficult to obtain in practical scenarios.
Several methodologies to optimize the operation of BESS for peak shaving in distribution grids were presented in the literature. For example, a rule-based control strategy for peak shaving was proposed in Manojkumar et al. (2021). Dynamic load and feed-in-tariffs are used for day-ahead load and PV profile predictions, which are then used to optimize the charging/discharging of BESS to reduce peak load. A similar research has presented a combination of predictive control and load/generation forecasting for peak shaving at shopping malls in Barchi et al. (2019). It was found that the proposed methodology can effectively shave 55% of the load when the electricity price is maximum and thus provide maximum profit to the customers. Dynamic programming was used to control the BESS for peak shaving and primary frequency regulation in Engels et al. (2019).
A coordinated control strategy to optimize the number of vanadium redox batteries and their charging/discharging profiles for peak shaving was proposed in Li J. et al. (2020). However, the study did not consider the degradation analysis of the batteries which is required to demonstrate the effects of charging and discharging on their health and loss of life. A controller was designed based on a predefined threshold load for BESS charging/discharging in Lucas and Chondrogiannis (2016). The command was set to charge the BESS when the load is below 10 kW and discharge when the load exceeds 400 kW. Although the control scheme proposed is simple and reasonably effective for peak shaving, the variations in load demands were not analyzed. Also, the methodology did not demonstrate how multiple distributed BESS could interact with the system operators while providing their services.
In distribution grids, most of the distributed RESs comprise PVs and wind generators Kryonidis et al. (2021). PV and wind have common intermittent characteristics, and their output power depends on the availability of the primary energy source, Sun and wind. This intermittency can affect the stability and reliability of power grids as the variations in Sun, and wind availability can lead to technical issues such as voltage fluctuations (flicker), which can affect the performance of voltage regulating devices Islam et al. (2016); Prakash et al. (2017b). The most common traditional approaches for mitigating power smoothing issues are to operate the distributed RESs below the maximum power point (MPP) limit or actively utilize load participation to minimize the output power fluctuation of the renewable generators Sukumar et al. (2018). While these approaches can be reasonably successful for power smoothing, they can increase the energy losses, reduce the efficiency of renewable generators and increase the operating costs. The fast response characteristics of BESS provide a potential power smoothing solution for modern power grids with high renewable energy penetration, where BESS can be deployed to operate as an energy buffer/filter Datta et al. (2021). The following discussion is divided into two parts to describe the recent control strategies for power smoothing using BESS by considering wind and solar generation applications.
An intelligent control strategy for wind output power smoothing was proposed in Lin et al. (2017a). The control strategy has used a recurrent fuzzy neural network to determine the wind power smoothing curve, which was then compared to the actual wind power. Any differences between the actual and the smoothed power were filtered through BESS charging/discharging. A finite-time convergence robust control algorithm of BESS was proposed to reduce wind power fluctuations in Deng et al. (2017). The conservative estimation of maximum uncertainty guaranteed stability but resulted in chattering effects. The chattering effects can damage the system in practical scenarios. Hence, a real-time estimation of uncertainties is required to enhance the dynamic capabilities of the controller. A control strategy for short-term wind output power smoothing using BESS was proposed in Jannati and Foroutan (2020). The methodology aimed to reduce power fluctuation, however, it did not consider the state of health of the batteries and their effects on the power allocation strategies. A combined algorithm for energy management and wind power smoothing using BESS was proposed in Altin and Eyimaya (2018).
A neural network predictive control algorithm to mitigate PV power fluctuations in distribution networks was proposed in Syed and Khalid (2021). The controller optimized the BESS SoC using the input parameters from the neural network model (predicted PV power). Still, the precision of the neural network plant model is dependent on the quality of data. A similar study obtains PV power smoothing using a probabilistic fuzzy neural network and BESS in Lin et al. (2017b), but does not consider the health of the battery in the simulation. Excessive charging or discharging of the batteries or even significant delays could have a negative impact on the battery’s health. The methodology uses Saviztky-Golay filter for PV power smoothing with the help of BESS in Atif and Khalid (2020). The results show that the method leads to unnecessary battery consumption and increases the battery cost if a significant delay is introduced. A BESS control for PV power intermittency smoothing using an optimized two-stage filter was proposed in Nazaripouya et al. (2017). More BESS-based algorithms for power smoothing and improving power quality can be found in Lallu et al. (2017); Islam et al. (2020); Guo et al. (2020).
Table 5 presents a summary of the BESS-based methodologies for long-term ancillary services, which are classified as congestion management, peak shaving, and power smoothing. For each journal article, the method, significant contributions, and limitations are summarized and presented in Table 5.
Several countries and governments have introduced energy storage system policies to motivate higher adoption of clean energy generation and reduce greenhouse gas emissions. The policies from different countries differ as they are developed based on their requirements. For instance, Australia and the United States have introduced policies to improve the power systems’ stability. Japan aims to provide backup power support during emergency shutdowns due to the damages from natural disasters, Germany adopted policies to promote higher renewable energy integration in the grid, while South Korea aims to reduce peak demand using higher adoption of BESS Lee (2015); Sani et al. (2020). Although all policies have a different perspective toward BESS, they are not limited to one specific area and are flexibly operated to provide multiple grid ancillary support. The ESS policies of different countries are described as follows.
Under the ARENA Act 2011, the Australian Renewable Energy Agency (ARENA) was founded in 2012 to lower the cost and increase the use of renewable energy in Australia. ARENA, which now serves as Australia’s primary ESS development support mechanism, has made significant investments in battery storage as they know that the ESS technology can help the agency achieve its goals and objectives Australian renewable energy agency (2022).
A low carbon investment plan policy strategy was developed by the South Australian (SA) government in 2015. The government has made substantial investments toward low-carbon energy and has already attained 52.1 per cent renewable energy penetration Clean energy council (2020). Through this project, ESS will primarily be used to prevent power quality and curtailment problems that could result from the intermittent nature of renewable energy sources H. Britton (2015). Moreover, the Adelaide City Council was the first Australian government to give direct financial incentives for battery storage coupled with solar PV installations in 2015 as part of the sustainable city incentive scheme. The scheme was introduced to target business owners, residential customers, community organizations, and educational institutions. Soon after the program started and its potential became apparent, the SA government matched Adelaide City Council’s funds to construct a 600 kWh battery energy storage S.C. Staff (2016). The SA government introduced the home battery program in October 2018 to offer subsidies to residential customers installing batteries alongside their rooftop solar PV systems Home battery scheme (2020). To provide the necessary grid support services, the SA government erected the Hornsdale power reserve, the largest Li-ion battery in the world (100MW/129 MWh). The details of these projects can be found in Section 5.
The Sustainable Energy Policy Framework, introduced in 2011, supports the Australian Capital Territory’s (ACT) ambitious goal of generating 100 per cent renewable energy by 2025. The government believes battery ESS is essential to attaining some of the policy’s goals ACT Government (2011). One of the government’s schemes, Next Generation Renewables, made it easier for 5,000 families and companies by installing 36 MW of battery storage between 2016 and 2020 Environment Planning and Sustainable Development Directorate (2021a). Another program created to aid the development of the clean economy is the Renewable Energy Industry Development Strategy (REIDS), which aims to develop the ESS and renewable industries in ACT Environment Planning and Sustainable Development Directorate (2021b).
To increase and develop new markets for ESS in the United States, both the federal and state governments have promoted policies that encourage investment, tax reduction, subsidy assistance, and extension of public supply Lee (2015). The primary contribution of the state governments is to encourage higher adoption of ESS and renewable energy by developing policies to provide subsidies for new ESS and renewable installations. In contrast, the federal government’s aim is to promote business investments Lee (2015). A list of most common ESS policies and contributions of the US government are described below.
• Section 1301 of the legislation helped the American Energy Innovation Act to authorize a 5 year fund of
• $1.4 billion for ESS research and development in 2020 American Energy Innovation Act (2020).
• Farm Bill (2019) provided financial support to the programmes run by the Department of Agriculture to promote ESS installations on farms and small businesses in rural communities Energy Storage Association (2020).
• The Advanced Research project’s Agency provided funding for long-term energy innovation in 2018 to support the development of technologies that can utilize ESS to power the US grid for 100 h A Zablocki (2019).
• ESS tax incentive bill S.3159 was introduced in 2016 to provide tax credits for ESS that have a minimum capacity of 5 kWh Congressional Research Service (2016).
• Residential Energy Property Tax Credit provides incentives to the ESS owners with a minimum capacity of 3 kWh Martin Heinrich (2016).
• Storage 2013 Act was introduced to encourage high installations of ESS by providing tax incentives to the owners and businesses United States Senate Committee on Energy & Natural Resources (2013).
• Law HB2193 was imposed in 2015 to ensure that the utilities have a minimum five MWh operational ESS by 2020 P. Maloney (2017).
• California state government announced Bill AB2514 in 2010 to ensure that the state-owned utilities establish 1325 MW ESS by 2020 Hart et al. (2018).
• H.4857 and Clean Energy Standard were passed in 2018 to ensure that Massachusetts and New York install 1000 MWh and 1500 MWh of ESS by 2025, respectively A. Zablocki (2019).
• The New Jersey state passed A3723 in 2018 to ensure 2000 MW ESS installation by 2030 A. Zablocki (2019).
The European Union (EU) significantly promotes clean energy generation in Europe by supporting the development of renewable energy technologies. Several European countries are developing their ESS policies to avoid obstacles that interfere with the deployment of ESS Sani et al. (2020). These policies are introduced to encourage higher ESS installations by providing subsidies, incentives, and research grants and promote the ESS provisions for ancillary grid support in Europe.
The United Kingdom (United Kingdom) does not provide direct subsidies for the deployment of energy storage systems as they believe that the energy industry should not be dependent on the subsidies Sani et al. (2020). However, the government provides a lot of funding for research and development to promote innovation in the sector Potau et al. (2018). The KfW Bank in Germany collaborated with the Federal Ministry of Economic Affairs and Energy to introduce a low-interest subsidy and load scheme for interested ESS and renewable energy buyers International Energy Agency (2016).
Other European countries also have regulations related to ESS. However, some barriers are limiting the speedy adoption of ESS. For example, the ESS facilities do not have business interests in the Netherlands as the government is solely promoting renewable energy generation to achieve the clean energy targets Sani et al. (2020). As a result, no adequate policies or regulations were introduced for ESS. Similarly, the progress on BESS deployment in Italy is plodding as there are no policies to support it Sani et al. (2020).
Several countries in Asia have been developing and improving the ESS policies to reduce greenhouse gas emissions and increase clean energy generation. To promote battery technology in Japan, a battery storage project was introduced by the Ministry of Trade and Industry in 2012. Supportive policies and market opportunities were provided by the Ministry of Trade and Industry (2012). In 2014, the government launched the fourth strategic energy plan that aimed to establish a resilient multi-layer energy supply to ensure power system stability Ministry of Trade and Industry (2014). Moreover, the Japanese government provides one-third of the total ESS installations costs from government subsidies and other relevant programs. They aim to ensure that the Japanese power grid is prepared to provide the fast response needed during natural disaster and loss of large generation Lee (2015). Similarly, the Chinese government has been supporting relevant ESS policies since 2005 to meet the clean energy targets. The policies have focused on a variety of areas that might advance and guarantee the quick development of ESS, including market development, grid-connected operation management, development pattern environmental protection, and financial assistance Yang and Zhao (2018).
Battery technology provides a promising solution for ancillary grid services and brings a diverse range of benefits to their owners and utilities Kumar et al. (2020a). However, to demonstrate the feasibility of the widespread adoption of BESS, it is essential to evaluate the cost and benefits of the commissioned BESS projects comprehensively and systematically. In this section, we present a cost-benefit analysis of BESS projects that have been commissioned globally. Only the projects that have publicly released the data are evaluated for this review. The benefits of adopting BESS for grid applications are summarized from the perspective of utility and independent power providers (IPPs). BESS, owned by the utility, usually generates revenue by participating in the wholesale ancillary markets for services such as frequency support and energy arbitrage. In contrast, the BESS owned by the IPPs are mostly used for resource adequacy support, and the revenue for those BESS is generated based on the contracts and agreements with the utilities Lazard (2018). Applications such as using BESS to replace or upgrade the existing infrastructure and utility-scale peak shaving also produce monetized benefits for the utility. Considering that the BESS cost and benefit analysis are particularly interesting for the investors, we divide the discussion into two subsections to evaluate the cost-benefit analysis from the investors’ perspectives (IPPs and utilities).
The IPP-owned BESS generates income for participating in wholesale ancillary markets and providing resource adequacy support to the system operators to improve the grid’s reliability. The IPPs mostly dominate the market share of large-scale BESS. For example, the IPPs in the United States (US) at the end of 2019 owned more than 56 percent of the existing power capacity of large-scale BESS participating in grid ancillary services US Energy Information Administration (2021). A summary of IPP-owned BESS projects around the world with public information is provided in Table 6. For example, a 20MW/80 MWh BESS was developed by AtlaGas Pomona Energy in the US for smoothing demand spikes and maximizing renewable energy generation. The total cost for deploying the BESS was around $40 to $45 million, which includes the cost of the battery pack, power electronic converters, energy management system, and engineering, procurement, and construction costs AltaGas (2017). Since the detailed benefit report for this project is not provided, revenue data from the California Independent System Operator (CAISO) is used to estimate the annual revenue and return of investment Lazard (2018). It is expected that the BESS will generate around $2.8 to $5.6 million in revenue and will take approximately 7–8 years to return its capital investment.
The Hornsdale power reserve project, owned and operated by Neoen, installed a 100MW/129 MWh lithium-ion battery to provide premium contingency frequency control ancillary service through its fast frequency response McLaren et al. (2017). In comparison to $7 million revenue in 2019, the project resulted in a $36 million increase in revenue in the first quarter of 2020. A small portion of this revenue was also provided by other minor BESS projects owned by Neoen. The total revenue of the Hornsdale battery has exceeded its investment cost of $96 million in just over 2 years after it started operations in late 2017 McLaren et al. (2017); Meng (2021). The Marengo project cost $20 million to invest in a 20MW/10 MWh battery unit for frequency regulation. It is estimated that the BESS will generate a revenue of approximately $5.599 million and will recover the total investment cost within 4 years DOE Office of Electricity (2019c). In 2019, the Gannawarra project in Victoria, Australia, installed 25MW/50 MWh BESS to support maximum renewable energy integration and regulate the frequency in the Victorian power grids. The annual operational report indicates that the BESS has generated $3.68 million in revenue in the first year itself and will take approximately 7 years to obtain a return of investment Edify (2021).
The Lake Bonney project invested in a 25MW/52 MWh BESS, which costs $41.6 million. The primary objectives of the BESS are to provide fast response duties and wind power curtailment. In the first year, the total income from the BESS ancillary services exceeded $10 million, which indicates that the BESS can recover its total investment cost in less than 5 years Infigen (2021). Several other field projects worldwide have invested in BESS for ancillary support. However, they have not disclosed the cost-benefit analysis. For instance, the Virtual Power Plant project, led by AGL Energy Limited, installed solar battery storage systems across 1,000 residential and business premises in Adelaide, South Australia. The role of these BESS is to provide RES power smoothing support and achieve peak load shaving to minimize the electricity bills of the customers Energy (2020). Details of similar projects such as AES Kilroot Carmen (2021a), Mt Newman Carmen (2021c), Butleigh Somerset Energy Matters (2018), Rabbit Hill DOE Office of Electricity (2019d) and Bulgana Carmen (2021b) can be found in Table 6.
The utilities invest in BESS to ensure the secure and economic operation of the grid, mainly due to the high integration of RES. The unity-owned BESS also participates in wholesale ancillary market services to generate revenue for their owners Liu et al. (2020). During high RES penetration, the generation exceeds the load demand and causes significant drops in electricity prices, which provides energy arbitrage opportunities for BESS. For instance, the BESS can be charged (purchase energy) from the excess renewable energy generation (during low electricity price) and discharged (sell energy) during peak hours when the electricity price is high to maximize the revenue Liu et al. (2020). A summary of utility-owned BESS projects is given in Table 6. For example, the Stafford Hill project invested in a 4MW/3.4 MWh BESS for peak shaving. The report from the US Department of Energy summarizes the cost-benefit of the project, which indicates that the total investment cost of the project was $5 million, and the annual benefit from the BESS services is estimated to be around $0.35 to $0.7 million US Department of Energy (2018a). As a result, it is estimated that the BESS will cover its investment cost in less than 10 years.
The Sterling BESS was installed in 2016 to provide multiple services such as peak shaving, energy arbitrage, and reliability support US Department of Energy (2018b). The project costs $2.5 million and generates annual revenue of $0.68 million (approximately 27 percent of its capital investment cost). In 2019, a similar project (Ballarat) in Victoria, Australia, invested in a 30MW/30 MWh BESS to provide frequency control, network stability, and congestion management support ARENA (2019). From the operational report, it is found that the project’s capital cost was $25 million, and the revenue from BESS services in the first year (2020) was $6.65 million. It is estimated that the BESS will reach its investment return in less than 4 years.
Other interesting BESS field projects that are owned by the utilities can be found in Table 6. However, the cost-benefit data were not disclosed for those projects. For example, the Willenhall project invested in a 2MW/1 MWh BESS for frequency regulation The University of Sheffield (2016), Snohomish PUD MESA 2 invested in 2.2MW/8 MWh BESS for peak shaving and energy arbitrage DOE Office of Electricity (2019e), Escondido installed 30MW/120 MWh BESS for peak shaving and reliability services DOE Office of Electricity (2019b), SCE LM600 Hybrid EGT - Grapeland added a 10MW/4.3 MWh BESS for spinning reserve, frequency regulation and load leveling DOE Office of Electricity (2019f), the Science and Technology Park project invested in a 10MW/5 MWh BESS for energy arbitrage, demand response and reliability services DOE Office of Electricity (2019g), the Ideal Energy MUM Ideal Energy (2019) and the MidAmerican Energy Storage Pilot Projects MidAmerican Energy Company (2019) invested in 0.35MW/1.05 MWh and 1MW/4 MWh BESS, respectively for improving renewable energy reliability and achieving peak shaving. The Convergent SCE Project installed a 35MW/140 MWh BESS for additional generation support in California DOE Office of Electricity (2019a).
Deploying an efficient BESS is a challenging task as several factors, such as costs, reliability, environmental issues, and their degradation, need to be considered Datta et al. (2021). This section presents the compendium of the literature and highlights the existing challenges associated with deploying and managing BESS in distribution grids. The substantial challenges in deploying BESS are summarized in Figure 6 and explained in the following paragraphs.
• Challenge 1: Economic concern for BESS investment and operation
The most critical factor for deploying BESS is the cost. Over-sizing BESS may not only increase the total investment costs but also lead to technical challenges in the grid Awad et al. (2014). As discussed in the above literature, many researchers have focused on minimizing the costs while planning the BESS for self-consumption, and ancillary support Engels et al. (2019); Zhang et al. (2016); Ramírez et al. (2018), Chua et al. (2016); Prasatsap et al. (2017). BESS costs depend on multiple factors, which include the type of BESS (technology), applications, geographical locations, investment costs, and maintenance requirements Awad et al. (2014). Other factors such as BESS degradation, power losses, and SoC can also have a direct impact on the BESS costs Mohamed et al. (2020).Solution: Developing an efficient BESS that considers economic concerns is a challenge, as various factors must be considered. These factors can be optimized by considering them within the optimization models to obtain lower BESS investment and operation costs. It is also essential for the governments to develop policies to provide incentives for BESS installations and participation. As described in Section 4, several countries are providing subsidies and incentives to encourage higher BESS installations while others are yet to introduce ESS policies.
• Challenge 2: Battery degradation, loss-of-life
Characteristics of an effective BESS are fast charging, slow discharging, and delayed degradation (increased lifetime) Mosca et al. (2019). An efficient design and control of BESS must consider the key factors that deteriorate the battery’s health. According to Sufyan et al. (2019), the four critical factors that impact the deterioration of battery capacity are the depth of discharge (DoD), battery lifetime, temperature, and the charging and discharging current, which are described as follows.
• Battery DoD: represents the percentage of battery discharged with respect to its rated capacity and allows for deep charge and discharge cycles, depending on the characteristic of the battery.
• Battery lifetime: represents the total number of cycles the battery can sustain and is measured as calendric and cyclic degradation. The calendric degradation, also known as constant degradation, is based on the chemical agents of BESS that are active due to the temperature and voltage. In contrast, cyclic degradation is dependent on the charging/discharging rate of BESS Mosca et al. (2019).
• Temperature: the battery degradation process is also dependent on the ambient temperature, which is known as the capacity fading phenomenon in the literature Sufyan et al. (2019).
• Charging and discharging current: current limits during the charging and discharging process plays an important role in delaying battery degradation. For instance, supplying a large current increases the internal resistance of the battery and reduces the capacity, which can harm the battery lifespan Sufyan et al. (2019).
Solution: To increase the efficiency of the battery and delay the degradation process, it is essential to consider the manufacturer’s recommended specifications, particularly optimum DoD in the design and operation stages Mosca et al. (2019); Sufyan et al. (2019). Additionally, the battery life is the most critical factor in the cost operation process as the battery’s lifetime depends on the number of charging and discharging cycles Mosca et al. (2019). Hence, optimizing the charging and discharging process for delayed battery degradation is essential. Moreover, low temperature increases the internal resistance of the battery, whereas high temperature increases the battery’s chemical reactions, both of which degrade the electrodes Ju and Wang (2016); Smith et al. (2012). Therefore, smart control algorithms are required to maintain the appropriate ambient temperature to improve the battery’s health.
• Challenge 3: Environmental threats imposed by BESS
Although BESS is used to increase the RES penetration in power grids and reduce greenhouse gas emissions, BESS itself may create some environmental threats Pombo et al. (2017) if not recycled properly. Battery recycling is a process of discarding degraded batteries. Since the batteries contain harmful toxic chemicals, dumping them as trash can result in severe environmental concerns Brogan et al. (2018).
Solution: The battery manufacturers should be aware of the health risks associated with the disposal of batteries and should provide appropriate recycling facilities Brogan et al. (2018). Recycling batteries is an ongoing process, and it is necessary to determine strategies for reusing/recycling the degraded BESS. It is essential for the governments to impose strict laws and enforcement related to battery recycling. The governments can also provide funding for research and development so that the researchers can work together to develop environmentally friendly storage technologies and appropriate recycling resources.
• Challenge 4: Availability and coordination of BESS and other DERs
The fast response and flexible characteristics of BESS provide a promising solution for increasing clean energy generation in power grids Qiu et al. (2018). However, BESS alone may not be able to solve all technical issues in a power grid Qiu et al. (2018). BESS should be coordinated with other available RES to provide an effective solution to the existing problems Qiu et al. (2018).
Solution: To achieve this, new technology advancements and control strategies are required. The optimization algorithms must consider the difficulties and restrictions of reducing greenhouse gas emissions while providing valuable support to the power grids. Due to system availability and high installation costs, BESS technology and application are only available in high-income countries. The possibility of introducing these technologies in low- and middle-income countries needs to be explored.
• Challenge 5: Lack of regulatory barriers to clarify the role of BESS
BESS has the technical capabilities for providing multiple grid ancillary services Jayasekara et al. (2015); Wang et al. (2018). However, the network providers and market operators may hesitate to deploy the BESS for those services if no regulations, legislation, or guidelines explicitly declare that BESS may do so Bhatnagar et al. (2013). Additionally, without assurances that BESS projects for ancillary services would be reimbursed, storage owners and system operators may be hesitant to undertake the necessary capital investments Bhatnagar et al. (2013).
Solution: The governments and energy departments need to establish regulations for BESS participation in energy, capacity, and ancillary service markets. The guidelines and rules must, among other things, ensure that BESS has open and equitable access to the market, considering its operating and technical characteristics Bowen et al. (2019). As described in Section 4, few governments have already established these requirements. However, there are several countries that still need to introduce the regulations and policies to promote high installation of BESS.
• Challenge 6: Deep charge or discharge of BESS
Inappropriate scheduling may result in deep charging or discharging of BESS, affecting the battery’s performance, state of health, and state of safety Han et al. (2014). Frequently, the deep discharge of batteries causes mechanical strains in the plates, resulting in shedding, poor conductivity, and a shortened system lifespan. The most frequent mechanical stress causes in active battery materials are large volume and crystallographic structure changes during the BESS charging and discharging process. Excessive voltages can initiate undesirable electrode reactions towards the completion of BESS charge or discharge, which can cause corrosion or gas evolution. As a result, the battery’s actual capacity may be lower than its rated capacity.
Solution: Depending on the measurable outputs such as temperature, voltage, and current, an effective battery management system can protect against deep charge or discharge and precisely calculate the functional status of the battery, including state of charge (SoC), state-of-health (SoH), state-of-function (SoF), and state-of-safety (SoS) Han et al. (2014). For instance, adaptive algorithms and data-driven estimating approaches were employed by the battery management systems, and they were compared to direct and indirect experimental assessments Xiong et al. (2018). The use of big data sets and machine learning as a method to improve these models was investigated by Howey (2019); Severson et al. (2019). It is also critical to reduce the computing burden of the models so that they can be employed in real-time applications Zhang et al. (2019).
• Challenge 7: Dynamic impacts of BESS
The dynamic effect of battery conversion efficiency on grid support is frequently overlooked in the literature. The dynamic model of BESS provides a simple representation of the battery cells and allows for analyzing the effects of battery degradation, dc-to-dc converters, voltage source converters, and the dynamics of the filter and transformer that connects the BESS to the grid Calero et al. (2020).
Solution: Including the dynamic characteristics in the design and planning phase will allow to evaluate the effects of dc-to-dc converter limits, and their dynamic responses on the ac side can be studied. Implementing the dc-to-dc converters will enable a more realistic representation of battery banks in the power grids, which can be helpful in analyzing the impacts of battery degradation, aging, and SOC on the battery cells in existing BESS facilities Calero et al. (2020).
• Challenge 8: BESS market for ancillary support
A lack of market for the services that BESS are specially designed to provide can make it difficult for developers and system operators to incorporate them as prospective sources of revenue. For instance, generators are used for frequency regulation support to provide the inertial, and governor response in most of the United States independent system operator markets Bowen et al. (2019). BESS can offer the same services faster and with better accuracy. Still, the lack of market opportunities to seek compensation for those services has become a significant obstacle for BESS deployment Bhatnagar et al. (2013). Additionally, given the nature of economies of scale and scope in operating the ancillary service markets and the connections between markets, the ultimate allocation of tasks is a matter of debate.
Solution: A centrally coordinated market can provide better opportunities to the interested network service providers and lower the driving information technology costs Pollitt and Anaya (2020). Market participants who wish to engage in simultaneous shares in multiple marketplaces may also favor it. One approach for a storage facility is to enter a single, long-term contract with the system operator and follow the directions from the system operator on how to run the facility and for what service. It is difficult for the DER owners to optimize their bids across several marketplaces at various scales. The capacity to co-optimize between regulated network investments and DER ancillary service solutions is required. It will enable the distribution network operators to award a contract to the DER owners to provide ancillary support such as congestion management or voltage regulation rather than improving its network.
In this paper, an up-to-date review of the role of static or fixed BESS for short-term and long-term ancillary services in the distribution grid was presented. The review process combines two innovative approaches, a PRISMA statement, and VOSviewer experiments, to identify and address the contemporary issues related to BESS provision for ancillary services and highlight the solutions for implementing intelligent and efficient algorithms. The most recent and relevant research papers are discovered using simple search strategies and filtering them based on inclusion and exclusion criteria. Then, the systematic PRISMA approach was used to provide a full review of the current approaches. This paper has utilized the VOSviewer visualization technique to identify significant research clusters from the sparse literature. It has been identified that the common ancillary services provided by BESS can be categorized into short-term and long-term services. As a result, we collected and reviewed the most important contributions and limitations of common short-term ancillary services (voltage control, frequency regulation, and black-start) and the long-term ancillary services (congestion management, peak shaving, and power smoothing). A cost-benefit analysis using commissioned BESS field projects was evaluated and presented in this review. The existing barriers to BESS deployment for ancillary services were also presented. To bridge the existing research gaps, potential future research directions were identified and can be summarized as follows.
• With the increasing renewable energy penetration in the distribution grid, the traditional approaches for ancillary support have become detrimental to the network equipment Qiu et al. (2018). Additionally, the uncoordinated provision of distributed RESs may lead to technical issues such as overvoltage, overloading, and power quality issues Qiu et al. (2018). Further research is needed to develop new control strategies for coordinating BESS with distributed RESs for fast and effective ancillary support.
• Several strategies have been applied to tackle optimal BESS allocation, sizing, and scheduling problems. However, to ensure robustness and reliability of the distribution grids to voltage, frequency, and power quality problems, more research effort is required to optimize and validate the transient and dynamic issues rather than the steady-state characteristics. The environmental constraints and seasonal variations need to be considered as well.
• More detailed analysis of BESS is required to consider BESS’s dynamic impact to accurately analyze the effects of battery degradation, dc-to-dc converters, voltage source inverters, and dynamic behavior of filters and transformers.
• To ensure efficient planning and operation of BESS, a comprehensive techno-economic analysis is required that should consider the capital costs, operational expenses, maintenance requirements, and key factors that affect the BESS aging.
• New control strategies should include the safety and protection of different types of BESS. A safety feature, such as early fault warning or fire protection schemes, can help avoid any occurrences of accidents and faults in the operational environment.
• Better battery management systems can enhance the performance of the battery and improve the state of health, and state-of-safety Han et al. (2014). Artificial intelligence, the internet of things, big data, and cyber protection could be utilized to provide safe, reliable, robust, and intelligent scheduling solutions for BESS-based grid ancillary support.
• Policies and regulations between energy and ancillary markets are required so that the BESS owners are aware of the rewards for participating in grid ancillary services. This may also increase the number of prosumers participating in grid ancillary support.
• Lack of market opportunities is the major obstacle that is delaying BESS deployment for grid ancillary support Bowen et al. (2019). There is an immediate need for the governments and energy departments to create valuable market opportunities so fast, and accurate BESS replaces the slow and less accurate traditional methods for ancillary support.
• To validate the developed algorithms on a physical network, experimental testing is required. While it may not be possible to test a developed strategy on an existing network, as it may compromise the operation of the grid, it is still possible through hardware-in-the-loop simulation. Therefore, digital models that replicate the existing power grids and real-world scenarios are required.
• Effective BESS recycling strategies are required to allow appropriate disposal of degraded BESS, as ineffective techniques can impose severe environmental threats Pombo et al. (2017).
• Since the world is experiencing a widespread adoption of EVs, it is necessary to analyse the impacts of mobile or dynamic EV batteries, and discover the solutions from hybrid energy storage systems for grid ancillary support.
KP: Conceptualization, Methodology, Software, Writing—original draft, preparation, Visualization. MA, AC, NK, and MS: Writing—review and editing, DD and HP: Supervision, Writing—review and editing.
This work is supported by the University of New South Wales (UNSW), Canberra, as a part of University International Postgraduate Award (UIPA) scholarship.
The authors declare that the research was conducted in the absence of any commercial or financial relationships that could be construed as a potential conflict of interest.
All claims expressed in this article are solely those of the authors and do not necessarily represent those of their affiliated organizations, or those of the publisher, the editors and the reviewers. Any product that may be evaluated in this article, or claim that may be made by its manufacturer, is not guaranteed or endorsed by the publisher.
Aghamohammadi, M. R., and Abdolahinia, H. (2014). A new approach for optimal sizing of battery energy storage system for primary frequency control of islanded microgrid. Int. J. Electr. Power & Energy Syst. 54, 325–333. doi:10.1016/j.ijepes.2013.07.005
Akhil, A. A., Huff, G., Currier, A. B., Kaun, B. C., Rastler, D. M., Chen, S. B., et al. (2015). Doe/epri electricity storage handbook in collaboration with nreca. Albuquerque, NM, USA: Sandia national laboratories.
Al-Saffar, M., and Musilek, P. (2020). Reinforcement learning-based distributed bess management for mitigating overvoltage issues in systems with high pv penetration. IEEE Trans. Smart Grid 11, 2980–2994. doi:10.1109/tsg.2020.2972208
Ali, M., Macana, C. A., Prakash, K., Islam, R., Colak, I., and Pota, H. (2020a). “Generating open-source datasets for power distribution network using openstreetmaps,” in 2020 9th International Conference on Renewable Energy Research and Application (ICRERA) (Glasgow, UK: IEEE), 301–308. doi:10.1109/ICRERA49962.2020.9242771
Ali, M., Macana, C. A., Prakash, K., Tarlinton, B., Islam, R., and Pota, H. (2020b). “A novel transfer learning approach to detect the location of transformers in distribution network,” in 2020 8th International Conference on Smart Grid (icSmartGrid) (Paris, France: IEEE), 56–60. doi:10.1109/icSmartGrid49881.2020.9144863
Ali, M., Prakash, K., Hossain, M. A., and Pota, H. R. (2021). Intelligent energy management: Evolving developments, current challenges, and research directions for sustainable future. J. Clean. Prod. 314, 127904. doi:10.1016/j.jclepro.2021.127904
Ali, M., Prakash, K., Macana, C., Bashir, A. K., Jolfaei, A., Bokhari, A., et al. (2022a). Modeling residential electricity consumption from public demographic data for sustainable cities. Energies 15, 2163. doi:10.3390/en15062163
Ali, M., Prakash, K., Macana, C., Rabiul, M., Hussain, A., and Pota, H. (2022b). Anonymization of distribution feeder data using statistical distribution and parameter estimation approach. Sustain. Energy Technol. Assessments 52, 102152. doi:10.1016/j.seta.2022.102152
Ali, M., Prakash, K., and Pota, H. (2020c). A bayesian approach based on acquisition function for optimal selection of deep learning hyperparameters: A case study with energy management data. Sci. Prcd. Ser. 2, 22–27. doi:10.31580/sps.v2i1.1232
Altin, N., and Eyimaya, S. E. (2018). A combined energy management algorithm for wind turbine/battery hybrid system. J. Electron. Mat. 47, 4430–4436. doi:10.1007/s11664-018-6159-z
Alyami, S., Wang, Y., Wang, C., Zhao, J., and Zhao, B. (2014). Adaptive real power capping method for fair overvoltage regulation of distribution networks with high penetration of pv systems. IEEE Trans. Smart Grid 5, 2729–2738. doi:10.1109/tsg.2014.2330345
Amrouche, S. O., Rekioua, D., Rekioua, T., and Bacha, S. (2016). Overview of energy storage in renewable energy systems. Int. J. Hydrogen Energy 41, 20914–20927. doi:10.1016/j.ijhydene.2016.06.243
Antoniadou-Plytaria, K. E., Kouveliotis-Lysikatos, I. N., Georgilakis, P. S., and Hatziargyriou, N. D. (2017). Distributed and decentralized voltage control of smart distribution networks: Models, methods, and future research. IEEE Trans. Smart Grid 8, 2999–3008. doi:10.1109/tsg.2017.2679238
Atif, A., and Khalid, M. (2020). Saviztky–golay filtering for solar power smoothing and ramp rate reduction based on controlled battery energy storage. IEEE Access 8, 33806–33817. doi:10.1109/access.2020.2973036
Awad, A. S., El-Fouly, T. H., and Salama, M. M. (2014). Optimal ess allocation for load management application. IEEE Trans. Power Syst. 30, 327–336. doi:10.1109/tpwrs.2014.2326044
Bahramipanah, M., Torregrossa, D., Cherkaoui, R., and Paolone, M. (2016). A decentralized adaptive model-based real-time control for active distribution networks using battery energy storage systems. IEEE Trans. Smart Grid 9, 3406–3418. doi:10.1109/tsg.2016.2631569
Barcellona, S., De Simone, D., and Piegari, L. (2019). Simple control strategy for a pv-battery system. J. Eng. (Stevenage). 2019, 4809–4812. doi:10.1049/joe.2018.9269
Barchi, G., Pierro, M., and Moser, D. (2019). Predictive energy control strategy for peak shaving and shifting using bess and pv generation applied to the retail sector. Electronics 8, 526. doi:10.3390/electronics8050526
Bhatnagar, D., Currier, A. B., Hernandez, J., Ma, O., and Kirby, B. (2013). “Market and policy barriers to energy storage deployment,” in Tech. rep. (Albuquerque, NM, United States: Sandia National Lab.(SNL-NM).
Boicea, V. A. (2014). Energy storage technologies: The past and the present. Proc. IEEE 102, 1777–1794. doi:10.1109/jproc.2014.2359545
Boles, J. D., Ma, Y., Wang, J., Osipov, D., Tolbert, L. M., and Wang, F. (2019). Converter-based emulation of battery energy storage systems (bess) for grid applications. IEEE Trans. Ind. Appl. 55, 4020–4032. doi:10.1109/tia.2019.2914421
Bowen, T., Chernyakhovskiy, I., and Denholm, P. L. (2019). “Grid-scale battery storage: Frequently asked questions,” in Tech. rep. (Golden, CO, United States: National Renewable Energy Lab.(NREL).
Brogan, P. V., Best, R. J., Morrow, D. J., McKinley, K., and Kubik, M. L. (2018). Effect of bess response on frequency and rocof during underfrequency transients. IEEE Trans. Power Syst. 34, 575–583. doi:10.1109/tpwrs.2018.2862147
Bullich-Massagué, E., Aragüés-Peñalba, M., Sumper, A., and Boix-Aragones, O. (2017). Active power control in a hybrid pv-storage power plant for frequency support. Sol. Energy 144, 49–62. doi:10.1016/j.solener.2016.12.033
Calero, F., Cañizares, C. A., and Bhattacharya, K. (2020). Dynamic modeling of battery energy storage and applications in transmission systems. IEEE Trans. Smart Grid 12, 589–598. doi:10.1109/tsg.2020.3016298
Chamana, M., and Chowdhury, B. H. (2018). Optimal voltage regulation of distribution networks with cascaded voltage regulators in the presence of high pv penetration. IEEE Trans. Sustain. Energy 9, 1427–1436. doi:10.1109/tste.2017.2788869
Chand, A. A., Prasad, A. K., Islam, F., Mamun, A. K., Kumar, N. M., Rajput, P., et al. (2020a). “Ac/dc microgrids,” in Microgrids (Boca Raton, FL, USA: CRC Press), 41–58.
Chand, A. A., Prasad, A. K., Mamun, A. K., Islam, F., Manoj, N. K., Rajput, P., et al. (2020b). “Microgrid modeling and simulations,” in Microgrids (Boca Raton, FL, USA: CRC Press), 59–79.
Chand, A. A., Prasad, K. A., Islam, F., Mamun, K. A., Kumar, N. M., and Prakash, K. (2020c). “An overview of various control and stability techniques for power-sharing in microgrids,” in International conference on emerging trends and advances in electrical engineering and renewable energy (Berlin, Germany: Springer), 1–14.
Chand, A. A., Prasad, K. A., Mamun, K. A., Sharma, K. R., and Chand, K. K. (2019). Adoption of grid-tie solar system at residential scale. Clean. Technol. 1, 224–231. doi:10.3390/cleantechnol1010015
Chaudhary, P., and Rizwan, M. (2018). Voltage regulation mitigation techniques in distribution system with high pv penetration: A review. Renew. Sustain. Energy Rev. 82, 3279–3287. doi:10.1016/j.rser.2017.10.017
Chua, K. H., Lim, Y. S., and Morris, S. (2016). Energy storage system for peak shaving. Int. J. Energy Sect. Manag. 10, 3–18. doi:10.1108/ijesm-01-2015-0003
Congressional Research Service (2016). S. 3159 (114th): Energy storage tax incentive and deployment act of 2016. Washington, DC, USA: Congressional Research Service.
Cortés, A., Mazón, J., and Merino, J. (2018). Strategy of management of storage systems integrated with photovoltaic systems for mitigating the impact on lv distribution network. Int. J. Electr. Power & Energy Syst. 103, 470–482. doi:10.1016/j.ijepes.2018.06.012
Danish, S. M. S., Ahmadi, M., Danish, M. S. S., Mandal, P., Yona, A., and Senjyu, T. (2020). A coherent strategy for peak load shaving using energy storage systems. J. Energy Storage 32, 101823. doi:10.1016/j.est.2020.101823
Das, C. K., Bass, O., Kothapalli, G., Mahmoud, T. S., and Habibi, D. (2018). Overview of energy storage systems in distribution networks: Placement, sizing, operation, and power quality. Renew. Sustain. Energy Rev. 91, 1205–1230. doi:10.1016/j.rser.2018.03.068
Datta, U., Kalam, A., and Shi, J. (2021). A review of key functionalities of battery energy storage system in renewable energy integrated power systems. Energy Storage 3, e224. doi:10.1002/est2.224
Datta, U., Kalam, A., and Shi, J. (2020). Smart control of bess in pv integrated ev charging station for reducing transformer overloading and providing battery-to-grid service. J. Energy Storage 28, 101224. doi:10.1016/j.est.2020.101224
de Mattos Affonso, C., and Kezunovic, M. (2018). Technical and economic impact of pv-bess charging station on transformer life: A case study. IEEE Trans. Smart Grid 10, 4683–4692. doi:10.1109/tsg.2018.2866938
de Siqueira, L. M. S., and Peng, W. (2021). Control strategy to smooth wind power output using battery energy storage system: A review. J. Energy Storage 35, 102252. doi:10.1016/j.est.2021.102252
Deng, Z., Xu, Y., Gu, W., and Fei, Z. (2017). Finite-time convergence robust control of battery energy storage system to mitigate wind power fluctuations. Int. J. Electr. Power & Energy Syst. 91, 144–154. doi:10.1016/j.ijepes.2017.03.012
Divshali, P. H., and Söder, L. (2017). Improving hosting capacity of rooftop pvs by quadratic control of an lv-central bss. IEEE Trans. Smart Grid 10, 919–927. doi:10.1109/tsg.2017.2754943
Divshali, P. H., and Söder, L. (2018). Improving pv dynamic hosting capacity using adaptive controller for statcoms. IEEE Trans. Energy Convers. 34, 415–425. doi:10.1109/tec.2018.2873057
DOE Office of Electricity (2019a). DOE global energy storage database - convergent 35 MW/140 MWh - SCE.
DOE Office of Electricity (2019d). DOE global energy storage database - Rabbit Hill energy storage project.
DOE Office of Electricity (2019f). DOE global energy storage database - SCE LM6000 hybrid EGT - Grapeland.
DOE Office of Electricity (2019g). DOE global energy storage database - university of Arizona science and technology Park/TEP - E.ON.
Engels, J., Claessens, B., and Deconinck, G. (2019). Optimal combination of frequency control and peak shaving with battery storage systems. IEEE Trans. Smart Grid 11, 3270–3279. doi:10.1109/tsg.2019.2963098
Environment Planning and Sustainable Development Directorate (2021b). Growth in the clean economy. Dickson: Environment Planning and Sustainable Development Directorate.
Environment Planning and Sustainable Development Directorate (2021a). Next generation energy storage (next gen) program. Dickson: Environment Planning and Sustainable Development Directorate.
Farhadi, M., and Mohammed, O. (2015). Energy storage technologies for high-power applications. IEEE Trans. Ind. Appl. 52, 1953–1961. doi:10.1109/tia.2015.2511096
Farivar, M., Zho, X., and Chen, L. (2015). “Local voltage control in distribution systems: An incremental control algorithm,” in 2015 IEEE international conference on smart grid communications (SmartGridComm) (Miami, FL, USA: IEEE), 732–737. doi:10.1109/SmartGridComm.2015.7436388
Farsadi̇, M., Sattarpur, T., and Nejadi̇, A. Y. (2016). Simultaneously optimal placement and operation scheduling of besss and dgs in distribution networks in order to minimizing net present value related to power losses. IU-Journal Electr. Electron. Eng. 16, 2081–2089.
Ferreira, H. L., Garde, R., Fulli, G., Kling, W., and Lopes, J. P. (2013). Characterisation of electrical energy storage technologies. Energy 53, 288–298. doi:10.1016/j.energy.2013.02.037
Guo, T., Liu, Y., Zhao, J., Zhu, Y., and Liu, J. (2020). A dynamic wavelet-based robust wind power smoothing approach using hybrid energy storage system. Int. J. Electr. Power & Energy Syst. 116, 105579. doi:10.1016/j.ijepes.2019.105579
Gupta, M., Kumar, V., Banerjee, G. K., and Sharma, N. (2017). Mitigating congestion in a power system and role of facts devices. Adv. Electr. Eng. 2017, 1–7. doi:10.1155/2017/4862428
Han, X., Ouyang, M., Lu, L., Li, J., Zheng, Y., and Li, Z. (2014). A comparative study of commercial lithium ion battery cycle life in electrical vehicle: Aging mechanism identification. J. Power Sources 251, 38–54. doi:10.1016/j.jpowsour.2013.11.029
Hannan, M., Wali, S., Ker, P., Abd Rahman, M., Mansor, M., Ramachandaramurthy, V., et al. (2021). Battery energy-storage system: A review of technologies, optimization objectives, constraints, approaches, and outstanding issues. J. Energy Storage 42, 103023. doi:10.1016/j.est.2021.103023
Hashemi, S., and Østergaard, J. (2016). Efficient control of energy storage for increasing the pv hosting capacity of lv grids. IEEE Trans. Smart Grid 9, 1–2303. doi:10.1109/tsg.2016.2609892
Hemmati, R., Saboori, H., and Jirdehi, M. A. (2017). Stochastic planning and scheduling of energy storage systems for congestion management in electric power systems including renewable energy resources. Energy 133, 380–387. doi:10.1016/j.energy.2017.05.167
Hesse, H. C., Schimpe, M., Kucevic, D., and Jossen, A. (2017). Lithium-ion battery storage for the grid—A review of stationary battery storage system design tailored for applications in modern power grids. Energies 10, 2107. doi:10.3390/en10122107
Howey, D. A. (2019). Tools for battery health diagnostics and prediction. Electrochem. Soc. Interface 28, 55–56. doi:10.1149/2.f06191if
Hu, J., You, S., Lind, M., and Østergaard, J. (2014). Coordinated charging of electric vehicles for congestion prevention in the distribution grid. IEEE Trans. Smart Grid 5, 703–711. doi:10.1109/tsg.2013.2279007
International Energy Agency (2016). Subsidy for solar PV with storage installations (Programm zur Förderung von PV-Batteriespeichern). Paris: International Energy Agency.
IRENA (2015a). Battery storage for renewables: Market status and technology outlook. Abu Dhabi, UAE: IRENA.
IRENA (2015b). REmap 2030 - renewable energy prospects: United States of America. Abu Dhabi, UAE: IRENA.
Islam, F., Lallu, A., Mamun, K., Prakash, K., and Roy, N. (2020). Power quality improvement of distribution network using bess and capacitor bank. J. Mod. Power Syst. Clean Energy 9, 625–632. doi:10.35833/mpce.2019.000304
Islam, F., Mamun, K., Prakash, K., and Lallu, A. (2017a). Aromatic network for power distribution system. IP Australia Grant Certificate.
Islam, F., Mamun, K., Prakash, K., Lallu, A., and Rattan, A. (2018). Impact of wind generators in power system stability. WSEAS Trans. Power Syst. 13, 235–248.
Islam, F., Prakash, K., Mamun, K. A., Lallu, A., and Pota, H. R. (2017b). Aromatic network: A novel structure for power distribution system. IEEE Access 5, 25236–25257. doi:10.1109/access.2017.2767037
Islam, F., Prakash, K., Mamun, K., Lallu, A., and Mudliar, R. R. (2016). Design a optimum MPPT controller for solar energy system. Indonesian J. Electr. Eng. Comput. Sci. 2, 545–553. doi:10.11591/ijeecs.v2.i3.pp545-553
Izadkhast, S., Cossent, R., Frías, P., García-González, P., and Rodríguez-Calvo, A. (2022). Performance evaluation of a bess unit for black start and seamless islanding operation. Energies 15, 1736. doi:10.3390/en15051736
Jannati, M., and Foroutan, E. (2020). Analysis of power allocation strategies in the smoothing of wind farm power fluctuations considering lifetime extension of bess units. J. Clean. Prod. 266, 122045. doi:10.1016/j.jclepro.2020.122045
Jayasekara, N., Masoum, M. A., and Wolfs, P. J. (2015). Optimal operation of distributed energy storage systems to improve distribution network load and generation hosting capability. IEEE Trans. Sustain. Energy 7, 250–261. doi:10.1109/tste.2015.2487360
Ju, C., and Wang, P. (2016). “Energy management system for microgrids including batteries with degradation costs,” in 2016 IEEE International Conference on Power System Technology (POWERCON) (Wollongong, NSW, Australia: IEEE), 1–6.
Kang, W., Chen, M., Guan, Y., Wei, B., Vasquez, J. C., and Guerrero, J. M. (2022). Event-triggered distributed voltage regulation by heterogeneous bess in low-voltage distribution networks. Appl. Energy 312, 118597. doi:10.1016/j.apenergy.2022.118597
Kerdphol, T., Fuji, K., Mitani, Y., Watanabe, M., and Qudaih, Y. (2016). Optimization of a battery energy storage system using particle swarm optimization for stand-alone microgrids. Int. J. Electr. Power & Energy Syst. 81, 32–39. doi:10.1016/j.ijepes.2016.02.006
Krata, J., and Saha, T. K. (2018). Real-time coordinated voltage support with battery energy storage in a distribution grid equipped with medium-scale pv generation. IEEE Trans. Smart Grid 10, 3486–3497. doi:10.1109/tsg.2018.2828991
Kryonidis, G. C., Kontis, E. O., Papadopoulos, T. A., Pippi, K. D., Nousdilis, A. I., Barzegkar-Ntovom, G. A., et al. (2021). Ancillary services in active distribution networks: A review of technological trends from operational and online analysis perspective. Renew. Sustain. Energy Rev. 147, 111198. doi:10.1016/j.rser.2021.111198
Kumar, N. M., Chand, A. A., Malvoni, M., Prasad, K. A., Mamun, K. A., Islam, F., et al. (2020a). Distributed energy resources and the application of ai, iot, and blockchain in smart grids. Energies 13, 5739. doi:10.3390/en13215739
Kumar, N. M., Chopra, S. S., Chand, A. A., Elavarasan, R. M., and Shafiullah, G. (2020b). Hybrid renewable energy microgrid for a residential community: A techno-economic and environmental perspective in the context of the sdg7. Sustainability 12, 3944. doi:10.3390/su12103944
Kundur, P., Paserba, J., Ajjarapu, V., Andersson, G., Bose, A., Canizares, C., et al. (2004). Definition and classification of power system stability ieee/cigre joint task force on stability terms and definitions. IEEE Trans. Power Syst. 19, 1387–1401.
Lakshmi, S., and Ganguly, S. (2019). Multi-objective planning for the allocation of pv-bess integrated open upqc for peak load shaving of radial distribution networks. J. Energy Storage 22, 208–218. doi:10.1016/j.est.2019.01.011
Lallu, A., Islam, F., Mamun, K., Prakash, K., and Cirrincione, M. (2017). “Power quality improvement of distribution network using optimum combination of battery energy storage system and capacitor banks,” in 2017 4th Asia-Pacific World Congress on Computer Science and Engineering (APWC on CSE) (Mana Island, Fiji: IEEE), 193–199. doi:10.1109/APWConCSE.2017.00043
Lazard (2018). Lazard’s levelized cost of storage analysis - version 4.0. New Orleans, Louisiana, USA: Lazard.
Lee, S. I. (2015). Plans for energy storage system market creation. Ulsan, South Korea: Korea Energy Economics Institute.
Li, C., Zhang, S., Li, J., Zhang, H., You, H., Qi, J., et al. (2020a). Coordinated control strategy of multiple energy storage power stations supporting black-start based on dynamic allocation. J. Energy Storage 31, 101683. doi:10.1016/j.est.2020.101683
Li, J., Hu, D., Mu, G., Wang, S., Zhang, Z., Zhang, X., et al. (2020b). Optimal control strategy for large-scale vrb energy storage auxiliary power system in peak shaving. Int. J. Electr. Power & Energy Syst. 120, 106007. doi:10.1016/j.ijepes.2020.106007
Li, J., You, H., Qi, J., Kong, M., Zhang, S., and Zhang, H. (2019). Stratified optimization strategy used for restoration with photovoltaic-battery energy storage systems as black-start resources. IEEE Access 7, 127339–127352. doi:10.1109/access.2019.2937833
Lin, F.-J., Chiang, H.-C., Chang, J.-K., and Chang, Y.-R. (2017a). Intelligent wind power smoothing control with bess. IET Renew. Power Gener. 11, 398–407. doi:10.1049/iet-rpg.2015.0427
Lin, F.-J., Lu, S.-Y., Chao, J.-Y., and Chang, J.-K. (2017b). Intelligent pv power smoothing control using probabilistic fuzzy neural network with asymmetric membership function. Int. J. Photoenergy 2017, 1–15. doi:10.1155/2017/8387909
Liu, J., Hu, C., Kimber, A., and Wang, Z. (2020). Uses, cost-benefit analysis, and markets of energy storage systems for electric grid applications. J. Energy Storage 32, 101731. doi:10.1016/j.est.2020.101731
Liu, L., Wu, J., Mi, Z., and Sun, C. (2016). “A feasibility study of applying storage-based wind farm as black-start power source in local power grid,” in 2016 International Conference on Smart Grid and Clean Energy Technologies (ICSGCE) (Chengdu, China: IEEE), 257–261. doi:10.1109/ICSGCE.2016.7876065
Liu, W., and Liu, Y. (2019). Energy storage sizing by copula modelling joint distribution for wind farm to be black-start source. IET Renew. Power Gener. 13, 1882–1890. doi:10.1049/iet-rpg.2018.6154
Lucas, A., and Chondrogiannis, S. (2016). Smart grid energy storage controller for frequency regulation and peak shaving, using a vanadium redox flow battery. Int. J. Electr. Power & Energy Syst. 80, 26–36. doi:10.1016/j.ijepes.2016.01.025
Maeyaert, L., Vandevelde, L., and Döring, T. (2020). Battery storage for ancillary services in smart distribution grids. J. Energy Storage 30, 101524. doi:10.1016/j.est.2020.101524
Mahlia, T., Saktisahdan, T., Jannifar, A., Hasan, M., and Matseelar, H. (2014). A review of available methods and development on energy storage; technology update. Renew. Sustain. Energy Rev. 33, 532–545. doi:10.1016/j.rser.2014.01.068
Malhotra, A., Battke, B., Beuse, M., Stephan, A., and Schmidt, T. (2016). Use cases for stationary battery technologies: A review of the literature and existing projects. Renew. Sustain. Energy Rev. 56, 705–721. doi:10.1016/j.rser.2015.11.085
Mamun, K. A., Islam, F., Haque, R., Chand, A. A., Prasad, K. A., Goundar, K. K., et al. (2022). Systematic modeling and analysis of on-board vehicle integrated novel hybrid renewable energy system with storage for electric vehicles. Sustainability 14, 2538. doi:10.3390/su14052538
Manganelli, M., Nicodemo, M., D’Orazio, L., Pimpinella, L., and Falvo, M. C. (2018). Restoration of an active mv distribution grid with a battery ess: A real case study. Sustainability 10, 2058. doi:10.3390/su10062058
Manojkumar, R., Kumar, C., Ganguly, S., and Catalão, J. P. (2021). Optimal peak shaving control using dynamic demand and feed-in limits for grid-connected pv sources with batteries. IEEE Syst. J. 15, 5560–5570. doi:10.1109/jsyst.2020.3045020
Marra, F., Yang, G., Træholt, C., Østergaard, J., and Larsen, E. (2014). A decentralized storage strategy for residential feeders with photovoltaics. IEEE Trans. Smart Grid 5, 974–981. doi:10.1109/tsg.2013.2281175
Martin Heinrich (2016). Heinrich introduces bipartisan bill to create tax credit for energy storage. Fallon, Nevada, USA: Martin Heinrich.
McLaren, J. A., Gagnon, P. J., and Mullendore, S. (2017). “Identifying potential markets for behind-the-meter battery energy storage: A survey of US demand charges,” in Tech. rep. (Golden, CO, United States: National Renewable Energy Lab.(NREL).
Meng, Y. (2021). Economic analysis of centralized energy storage and impact on nem. IOP Conf. Ser. Earth Environ. Sci. 680, 012004. doi:10.1088/1755-1315/680/1/012004
Meng, Y., Li, X., Liu, X., Cui, X., Xu, P., and Li, S. (2021). A control strategy for battery energy storage systems participating in primary frequency control considering the disturbance type. IEEE Access 9, 102004–102018. doi:10.1109/access.2021.3094309
Mexis, I., and Todeschini, G. (2020). Battery energy storage systems in the United Kingdom: A review of current state-of-the-art and future applications. Energies 13, 3616. doi:10.3390/en13143616
MidAmerican Energy Company (2019). Battery storage in knoxville Iowa. Des Moines, Iowa, USA: MidAmerican Energy Company.
Ministry of Trade and Industry (2014). 4th strategic energy plan. Singapore: Ministry of Trade and Industry.
Ministry of Trade and Industry (2012). METI establishes project team to promote storage battery industry. Singapore: Ministry of Trade and Industry.
Mishra, A., Irwin, D., Shenoy, P., and Zhu, T. (2013). “Scaling distributed energy storage for grid peak reduction,” in Proceedings of the Fourth International Conference on Future Energy Systems, 3–14.
Mishra, S., and Palanisamy, P. (2018). “Efficient power flow management and peak shaving in a microgrid-pv system,” in 2018 IEEE Energy Conversion Congress and Exposition (ECCE) (Portland, OR, USA: IEEE), 3792–3798. doi:10.1109/ECCE.2018.8558312
Mohamed, A. A. R., Morrow, D. J., Best, R. J., Bailie, I., Cupples, A., and Pollock, J. (2020). “Battery energy storage systems allocation considering distribution network congestion,” in 2020 IEEE PES Innovative Smart Grid Technologies Europe (ISGT-Europe) (The Hague, Netherlands: IEEE), 1015–1019. doi:10.1109/ISGT-Europe47291.2020.9248982
Mosca, C., Arrigo, F., Mazza, A., Bompard, E., Carpaneto, E., Chicco, G., et al. (2019). Mitigation of frequency stability issues in low inertia power systems using synchronous compensators and battery energy storage systems. IET Gener. Transm. &. Distrib. 13, 3951–3959. doi:10.1049/iet-gtd.2018.7008
Nair, U. R., Sandelic, M., Sangwongwanich, A., Dragičević, T., Costa-Castelló, R., and Blaabjerg, F. (2020). Grid congestion mitigation and battery degradation minimisation using model predictive control in pv-based microgrid. IEEE Trans. Energy Convers. 36, 1500–1509. doi:10.1109/tec.2020.3032534
Nazaripouya, H., Chu, C.-C., Pota, H. R., and Gadh, R. (2017). Battery energy storage system control for intermittency smoothing using an optimized two-stage filter. IEEE Trans. Sustain. Energy 9, 664–675. doi:10.1109/tste.2017.2754478
Nour, A. M., Hatata, A. Y., Helal, A. A., and El-Saadawi, M. M. (2019). Review on voltage-violation mitigation techniques of distribution networks with distributed rooftop pv systems. IET Gener. Transm. &. Distrib. 14, 349–361. doi:10.1049/iet-gtd.2019.0851
Nourai, A., Kogan, V., and Schafer, C. M. (2008). Load leveling reduces t&d line losses. IEEE Trans. Power Deliv. 23, 2168–2173. doi:10.1109/tpwrd.2008.921128
Olatomiwa, L., Mekhilef, S., Ismail, M. S., and Moghavvemi, M. (2016). Energy management strategies in hybrid renewable energy systems: A review. Renew. Sustain. Energy Rev. 62, 821–835. doi:10.1016/j.rser.2016.05.040
Page, M. J., and Moher, D. (2017). Evaluations of the uptake and impact of the preferred reporting items for systematic reviews and meta-analyses (prisma) statement and extensions: A scoping review. Syst. Rev. 6, 263–314. doi:10.1186/s13643-017-0663-8
Pillay, A., Karthikeyan, S. P., and Kothari, D. (2015). Congestion management in power systems–a review. Int. J. Electr. Power & Energy Syst. 70, 83–90. doi:10.1016/j.ijepes.2015.01.022
Podder, A. K., Islam, S., Kumar, N. M., Chand, A. A., Rao, P. N., Prasad, K. A., et al. (2020). Systematic categorization of optimization strategies for virtual power plants. Energies 13, 6251. doi:10.3390/en13236251
Pollitt, M. G., and Anaya, K. L. (2020). Competition in markets for ancillary services? The implications of rising distributed generation. Energy J. 41. doi:10.5547/01956574.42.si1.mpol
Pombo, A. V., Murta-Pina, J., and Pires, V. F. (2017). Multiobjective formulation of the integration of storage systems within distribution networks for improving reliability. Electr. Power Syst. Res. 148, 87–96. doi:10.1016/j.epsr.2017.03.012
Potau, X., Leistner, S., and Morrison, G. (2018). “Battery promoting policies in selected member states,” in European commission-N° ENER C2/2015-410, support to R&D strategy for battery based energy storage, battery promoting strategies in selected member states.
Prajapati, V. K., and Mahajan, V. (2021). Reliability assessment and congestion management of power system with energy storage system and uncertain renewable resources. Energy 215, 119134. doi:10.1016/j.energy.2020.119134
Prakash, K., Ali, M., Islam, M. R., Mo, H., Dong, D., and Pota, H. (2022a). “Optimal coordination of photovoltaics and electric vehicles for ancillary services in low voltage distribution networks,” in 2021 24th International Conference on Electrical Machines and Systems (ICEMS) (Gyeongju, Korea: IEEE), 1008–1013. doi:10.23919/ICEMS52562.2021.9634429
Prakash, K., Ali, M., Siddique, M., Karmaker, A., Macana, C., Dong, D., et al. (2022b). Bi-level planning and scheduling of electric vehicle charging stations for peak shaving and congestion management in low voltage distribution networks. Comput. Electr. Eng. 102, 108235. doi:10.1016/j.compeleceng.2022.108235
Prakash, K., Islam, F., Mamun, K., and Ali, S. (2017a). “Optimal generators placement techniques in distribution networks: A review,” in 2017 Australasian Universities Power Engineering Conference (AUPEC) (Melbourne, VIC, Australia: IEEE), 1–6. doi:10.1109/AUPEC.2017.8282381
Prakash, K., Islam, F., Mamun, K., Lallu, A., and Cirrincione, M. (2017b). “Reliability of power distribution networks with renewable energy sources,” in 2017 4th Asia-Pacific World Congress on Computer Science and Engineering (APWC on CSE) (Mana Island, Fiji: IEEE), 187–192. doi:10.1109/APWConCSE.2017.00042
Prakash, K., Islam, F., Mamun, K., and Pota, H. (2020). Configurations of aromatic networks for power distribution system. Sustainability 12, 4317. doi:10.3390/su12104317
Prakash, K., Lallu, A., Islam, F., and Mamun, K. (2016). “Review of power system distribution network architecture,” in 2016 3rd Asia-Pacific World Congress on Computer Science and Engineering (APWC on CSE) (Nadi, Fiji: IEEE), 124–130. doi:10.1109/APWC-on-CSE.2016.030
Prakash, K., Mamun, K., Islam, F., Chand, A. A., Prasad, K. A., Goundar, K. K., et al. (2019). “Hybrid electric vehicle: Designing a control of solar/wind/battery/capacitor/fuel cell hybrid system,” in 2019 29th Australasian Universities Power Engineering Conference (AUPEC) (Nadi, Fiji: IEEE), 1–6. doi:10.1109/AUPEC48547.2019.211802
Prasatsap, U., Kiravittaya, S., and Polprasert, J. (2017). Determination of optimal energy storage system for peak shaving to reduce electricity cost in a University. Energy Procedia 138, 967–972. doi:10.1016/j.egypro.2017.10.091
Procopiou, A. T., Petrou, K., Ochoa, L. F., Langstaff, T., and Theunissen, J. (2018). Adaptive decentralized control of residential storage in pv-rich mv–lv networks. IEEE Trans. Power Syst. 34, 2378–2389. doi:10.1109/tpwrs.2018.2889843
Qiu, J., Xu, Z., Zheng, Y., Wang, D., and Dong, Z. Y. (2018). Distributed generation and energy storage system planning for a distribution system operator. IET Renew. Power Gener. 12, 1345–1353. doi:10.1049/iet-rpg.2018.5115
Rajan, R., Fernandez, F. M., and Yang, Y. (2021). Primary frequency control techniques for large-scale pv-integrated power systems: A review. Renew. Sustain. Energy Rev. 144, 110998. doi:10.1016/j.rser.2021.110998
Ramírez, M., Castellanos, R., Calderón, G., and Malik, O. (2018). Placement and sizing of battery energy storage for primary frequency control in an isolated section of the mexican power system. Electr. Power Syst. Res. 160, 142–150. doi:10.1016/j.epsr.2018.02.013
Rana, M. M., Uddin, M., Sarkar, M. R., Shafiullah, G., Mo, H., and Atef, M. (2022). A review on hybrid photovoltaic–battery energy storage system: Current status, challenges, and future directions. J. Energy Storage 51, 104597. doi:10.1016/j.est.2022.104597
Roberts, B. (2009). Capturing grid power. IEEE Power Energy Mag. 7, 32–41. doi:10.1109/mpe.2009.932876
Rotella Junior, P., Rocha, L. C. S., Morioka, S. N., Bolis, I., Chicco, G., Mazza, A., et al. (2021). Economic analysis of the investments in battery energy storage systems: Review and current perspectives. Energies 14, 2503. doi:10.3390/en14092503
Sabihuddin, S., Kiprakis, A. E., and Mueller, M. (2014). A numerical and graphical review of energy storage technologies. Energies 8, 172–216. doi:10.3390/en8010172
Samadi, A., Eriksson, R., Söder, L., Rawn, B. G., and Boemer, J. C. (2014). Coordinated active power-dependent voltage regulation in distribution grids with pv systems. IEEE Trans. Power Deliv. 29, 1454–1464. doi:10.1109/tpwrd.2014.2298614
Sani, S. B., Celvakumaran, P., Ramachandaramurthy, V. K., Walker, S., Alrazi, B., Ying, Y. J., et al. (2020). Energy storage system policies: Way forward and opportunities for emerging economies. J. Energy Storage 32, 101902. doi:10.1016/j.est.2020.101902
Severson, K. A., Attia, P. M., Jin, N., Perkins, N., Jiang, B., Yang, Z., et al. (2019). Data-driven prediction of battery cycle life before capacity degradation. Nat. Energy 4, 383–391. doi:10.1038/s41560-019-0356-8
Shayani, R. A., and de Oliveira, M. A. G. (2010). Photovoltaic generation penetration limits in radial distribution systems. IEEE Trans. Power Syst. 26, 1625–1631. doi:10.1109/tpwrs.2010.2077656
Smith, K., Earleywine, M., Wood, E., and Pesaran, A. (2012). “Battery wear from disparate duty-cycles: Opportunities for electric-drive vehicle battery health management,” in Tech. rep. (Golden, CO, United States: National Renewable Energy Lab.(NREL).
Stecca, M., Elizondo, L. R., Soeiro, T. B., Bauer, P., and Palensky, P. (2020). A comprehensive review of the integration of battery energy storage systems into distribution networks. IEEE Open. J. Ind. Electron. Soc. 1, 1–65. doi:10.1109/ojies.2020.2981832
Strunck, C., Albrecht, M., Meindl, G., and Rehtanz, C. (2019). A study on the black start process of a real distribution network with chp plants and bess. EPJ Web Conf. 217, 01015. doi:10.1051/epjconf/201921701015
Strunck, C., Jablonowski, D., and Rehtanz, C. (2021). “Development of an algorithm for black start capability assessment of distribution grids with high penetration of renewable energies,” in ETG Congress 2021 (Frankfurt am Main: VDE), 1–6.
Subramani, G., Ramachandaramurthy, V. K., and Vijyakumar, K. N. (2018). Optimal sizing of battery energy storage system (bess) for peak shaving under malaysian electricity tariff. Adv. Sci. Lett. 24, 1861–1865. doi:10.1166/asl.2018.11177
Sufyan, M., Rahim, N. A., Aman, M. M., Tan, C. K., and Raihan, S. R. S. (2019). Sizing and applications of battery energy storage technologies in smart grid system: A review. J. Renew. Sustain. Energy 11, 014105. doi:10.1063/1.5063866
Sukumar, S., Mokhlis, H., Mekhilef, S., Karimi, M., and Raza, S. (2018). Ramp-rate control approach based on dynamic smoothing parameter to mitigate solar pv output fluctuations. Int. J. Electr. Power & Energy Syst. 96, 296–305. doi:10.1016/j.ijepes.2017.10.015
Syed, M. A., and Khalid, M. (2021). Neural network predictive control for smoothing of solar power fluctuations with battery energy storage. J. Energy Storage 42, 103014. doi:10.1016/j.est.2021.103014
Tan, X., Li, Q., and Wang, H. (2013). Advances and trends of energy storage technology in microgrid. Int. J. Electr. Power & Energy Syst. 44, 179–191. doi:10.1016/j.ijepes.2012.07.015
Tan, Z., Li, X., He, L., Li, Y., and Huang, J. (2020). Primary frequency control with bess considering adaptive soc recovery. Int. J. Electr. Power & Energy Syst. 117, 105588. doi:10.1016/j.ijepes.2019.105588
Telaretti, E., and Dusonchet, L. (2017). “Stationary battery systems in the main world markets: Part 1: Overview of the state-of-the-art,” in 2017 IEEE International Conference on Environment and Electrical Engineering and 2017 IEEE Industrial and Commercial Power Systems Europe (EEEIC/I&CPS Europe) (Milan, Italy: IEEE), 1–5. doi:10.1109/EEEIC.2017.7977799
Tewari, T., Mohapatra, A., and Anand, S. (2020). Coordinated control of oltc and energy storage for voltage regulation in distribution network with high pv penetration. IEEE Trans. Sustain. Energy 12, 262–272. doi:10.1109/tste.2020.2991017
The University of Sheffield (2016). Willenhall: 2MW battery energy storage demonstrator. Sheffield, England: The University of Sheffield.
Todorovski, M. (2014). Transformer voltage regulation—Compact expression dependent on tap position and primary/secondary voltage. IEEE Trans. Power Deliv. 29, 1516–1517. doi:10.1109/tpwrd.2014.2311959
Uddin, M., Romlie, M. F., Abdullah, M. F., Abd Halim, S., Kwang, T. C., and Chia Kwang, T. (2018). A review on peak load shaving strategies. Renew. Sustain. Energy Rev. 82, 3323–3332. doi:10.1016/j.rser.2017.10.056
United States Senate Committee on Energy & Natural Resources (2013). Summary of the storage technology for renewable and green energy act of 2013, or the “STORAGE 2013 Act,”2013.
US Department of Energy (2018a). Spotlight: Solving challenges in energy storage. Washington, DC, USA: US Department of Energy.
US Department of Energy (2018b). U.S. Department of energy and Sandia national laboratories, One year in: Energy storage proves its worth in sterling. Washington, DC, USA: US Department of Energy.
US Energy Information Administration (2021). Battery storage in the United States: An update on market trends. Washington, DC, USA: US Energy Information Administration.
van Westering, W., and Hellendoorn, H. (2020). Low voltage power grid congestion reduction using a community battery: Design principles, control and experimental validation. Int. J. Electr. Power & Energy Syst. 114, 105349. doi:10.1016/j.ijepes.2019.06.007
Wang, L., Bai, F., Yan, R., and Saha, T. K. (2018). Real-time coordinated voltage control of pv inverters and energy storage for weak networks with high pv penetration. IEEE Trans. Power Syst. 33, 3383–3395. doi:10.1109/tpwrs.2018.2789897
Wang, L., Liang, D. H., Crossland, A. F., Taylor, P. C., Jones, D., and Wade, N. S. (2015a). Coordination of multiple energy storage units in a low-voltage distribution network. IEEE Trans. Smart Grid 6, 2906–2918. doi:10.1109/tsg.2015.2452579
Wang, Y., Tan, K., Peng, X. Y., and So, P. L. (2015b). Coordinated control of distributed energy-storage systems for voltage regulation in distribution networks. IEEE Trans. Power Deliv. 31, 1132–1141. doi:10.1109/tpwrd.2015.2462723
Wang, Y., Zhang, N., Kang, C., Miao, M., Shi, R., and Xia, Q. (2017). An efficient approach to power system uncertainty analysis with high-dimensional dependencies. IEEE Trans. Power Syst. 33, 2984–2994. doi:10.1109/tpwrs.2017.2755698
Wankmüller, F., Thimmapuram, P. R., Gallagher, K. G., and Botterud, A. (2017). Impact of battery degradation on energy arbitrage revenue of grid-level energy storage. J. Energy Storage 10, 56–66. doi:10.1016/j.est.2016.12.004
Wu, D., Tang, F., Dragicevic, T., Guerrero, J. M., and Vasquez, J. C. (2015). Coordinated control based on bus-signaling and virtual inertia for islanded dc microgrids. IEEE Trans. Smart Grid 6, 2627–2638. doi:10.1109/tsg.2014.2387357
Wu, X., Zhao, J., and Conejo, A. J. (2021). Optimal battery sizing for frequency regulation and energy arbitrage. IEEE Trans. Power Deliv. 37, 2016. doi:10.1109/TPWRD.2021.3102420
Wu, Z., Gao, D. W., Zhang, H., Yan, S., and Wang, X. (2017). Coordinated control strategy of battery energy storage system and pmsg-wtg to enhance system frequency regulation capability. IEEE Trans. Sustain. Energy 8, 1330–1343. doi:10.1109/tste.2017.2679716
Xiong, R., Li, L., and Tian, J. (2018). Towards a smarter battery management system: A critical review on battery state of health monitoring methods. J. Power Sources 405, 18–29. doi:10.1016/j.jpowsour.2018.10.019
Xu, J., Liu, B., Mo, H., and Dong, D. (2021). Bayesian adversarial multi-node bandit for optimal smart grid protection against cyber attacks. Automatica 128, 109551. doi:10.1016/j.automatica.2021.109551
Yang, F.-f., and Zhao, X.-g. (2018). Policies and economic efficiency of China’s distributed photovoltaic and energy storage industry. Energy 154, 221–230. doi:10.1016/j.energy.2018.04.135
Yang, Y., Bremner, S., Menictas, C., and Kay, M. (2018). Battery energy storage system size determination in renewable energy systems: A review. Renew. Sustain. Energy Rev. 91, 109–125. doi:10.1016/j.rser.2018.03.047
Zecchino, A., Yuan, Z., Sossan, F., Cherkaoui, R., and Paolone, M. (2021). Optimal provision of concurrent primary frequency and local voltage control from a bess considering variable capability curves: Modelling and experimental assessment. Electr. Power Syst. Res. 190, 106643. doi:10.1016/j.epsr.2020.106643
Zeraati, M., Golshan, M. E. H., and Guerrero, J. M. (2017). A consensus-based cooperative control of pev battery and pv active power curtailment for voltage regulation in distribution networks. IEEE Trans. Smart Grid 10, 670–680. doi:10.1109/tsg.2017.2749623
Zeraati, M., Golshan, M. E. H., and Guerrero, J. M. (2016). Distributed control of battery energy storage systems for voltage regulation in distribution networks with high pv penetration. IEEE Trans. Smart Grid 9, 3582–3593. doi:10.1109/tsg.2016.2636217
Zhang, Y. J. A., Zhao, C., Tang, W., and Low, S. H. (2016). Profit-maximizing planning and control of battery energy storage systems for primary frequency control. IEEE Trans. Smart Grid 9, 712–723. doi:10.1109/tsg.2016.2562672
Zhang, Y., Xiong, R., He, H., Qu, X., and Pecht, M. (2019). Aging characteristics-based health diagnosis and remaining useful life prognostics for lithium-ion batteries. ETransportation 1, 100004. doi:10.1016/j.etran.2019.100004
Zhang, Z., Dou, C., Yue, D., Zhang, Y., Zhang, B., and Zhang, Z. (2022). Event-triggered hybrid voltage regulation with required bess sizing in high-pv-penetration networks. IEEE Trans. Smart Grid 13, 2614–2626. doi:10.1109/tsg.2022.3168440
Keywords: ancillary services, battery energy storage system, distribution grids, long-term ancillary services, short-term ancillary services, renewable energy sources
Citation: Prakash K, Ali M, Siddique MNI, Chand AA, Kumar NM, Dong D and Pota HR (2022) A review of battery energy storage systems for ancillary services in distribution grids: Current status, challenges and future directions. Front. Energy Res. 10:971704. doi: 10.3389/fenrg.2022.971704
Received: 17 June 2022; Accepted: 16 August 2022;
Published: 16 September 2022.
Edited by:
Sudhakar Babu Thanikanti, Chaitanya Bharathi Institute of Technology, IndiaReviewed by:
Palanisamy K, Vellore Institute of Technology, IndiaCopyright © 2022 Prakash, Ali, Siddique, Chand, Kumar, Dong and Pota. This is an open-access article distributed under the terms of the Creative Commons Attribution License (CC BY). The use, distribution or reproduction in other forums is permitted, provided the original author(s) and the copyright owner(s) are credited and that the original publication in this journal is cited, in accordance with accepted academic practice. No use, distribution or reproduction is permitted which does not comply with these terms.
*Correspondence: Krishneel Prakash, a3Jpc2huZWVscHJha2FzaF83QHlhaG9vLmNvbQ==
Disclaimer: All claims expressed in this article are solely those of the authors and do not necessarily represent those of their affiliated organizations, or those of the publisher, the editors and the reviewers. Any product that may be evaluated in this article or claim that may be made by its manufacturer is not guaranteed or endorsed by the publisher.
Research integrity at Frontiers
Learn more about the work of our research integrity team to safeguard the quality of each article we publish.