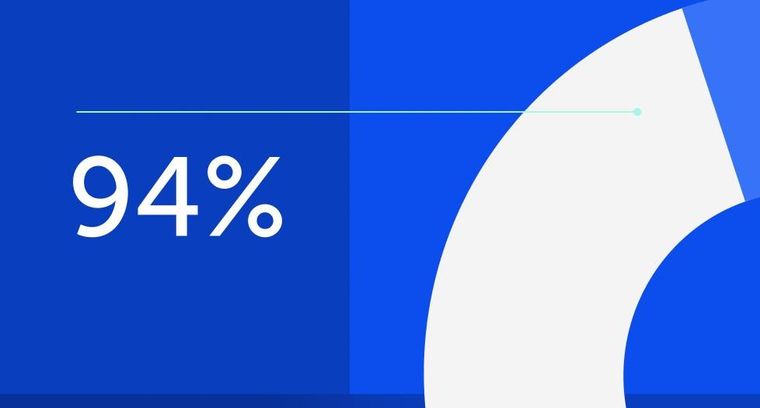
94% of researchers rate our articles as excellent or good
Learn more about the work of our research integrity team to safeguard the quality of each article we publish.
Find out more
MINI REVIEW article
Front. Energy Res., 26 January 2023
Sec. Electrochemical Energy Storage
Volume 10 - 2022 | https://doi.org/10.3389/fenrg.2022.957032
This article is part of the Research TopicMultiphysics, Multiphase and Multiscale Modeling and Characterization of Porous Media in Electrochemical Energy SystemsView all 5 articles
Supercapacitors became more and more important recently in the area of energy storage and conversion. Their large power deliveries abilities, high stability and environmental friendliness characteristics draw tremendous attention in high-power applications such as public transit networks. Carbonaceous materials with unique surface and electrochemical properties were widely used in supercapacitors as electrode materials. This review focuses on the developments in supercapacitor electrodes made from carbonaceous materials recently, their working principle and evaluation parameters were summarized briefly. The preparation methods and electrochemical properties of different carbonaceous materials were compared and classified. It was found that the surface situation (e.g., porous structure, hydrophilic) of carbonaceous materials strongly affect the electrochemical performances of supercapacitor. So far, active carbons would be the most applicable carbonaceous electrode materials owing to their good chemical stability and conductivity, extensive accessibility inexpensiveness. But their energy densities still fall behind practical demands. Both theoretical calculations and experimental studies show that surface modification and doping of carbonaceous materials can not only optimize their pore size, structure, conductivity and surface properties, but also can introduce extra pseudocapacitance into these materials. Considering global environmental pollution and energy shortage problems nowadays, we sincerely suggested that future work should focus on domestic, medical and industrial wastes residues derived carbonaceous materials and scaled production process such as reactors and exhaust gas treatment.
Over the past two decade, the comsumption and cost of gradual exhausted fossil fuels were significantly increased due to industrial developments and international competitions (Sujatha, 2021). Moreover, abuse of fossil fuels have being caused environmental pollution and global warming (Welsby et al., 2021). In order to cope these crises, wind energy, solar energy and other unstable but sustainable energy sources are urgently needed (Zhang et al., 2017; Chen et al., 2020; Shao et al., 2020). Therefore, efficient installation for energy storage, including and supercapacitors (or electrochemical capacitors) and batteries, have attracted tremendous attentions. Supercapacitors have unique advantages especially in terms of wide operating temperature range, no memory effect, good cycling stability and high watt density (Gao et al., 2013; Lee et al., 2017; Song et al., 2022a). The electrochemical performance comparison about supercapacitors, conventional capacitors and batteries were listed in Supplementary Table S1 (Salunkhe et al., 2016). Overall performance of supercapacitors currently lies between traditional dielectric capacitors and batteries. Generally the energy density of supercapacitors is relatively lower than that of many batteries, but the unique electricity storage process enables supercapacitors to store or release substantial amount of electricity in a very short time, which let supercapacitors become a most competitive candidate for the technologies that need are fast to transport and require a quick surge of electrical energy in a very short moment such as wind turbines, grid stabilization system, uninterruptable power supply, forklifts, military weapons, load cranes, hybrid electric vehicles, regenerative braking systems for electric vehicles and rail transit (Taberna et al., 2003; Meng et al., 2004; Chmiola et al., 2010; Wei et al., 2010; Cheng et al., 2011; Lv et al., 2020; Hasan Md et al., 2022).
So far, the working principle of common supercapacitors and electrode materials have been fully studied. Electrical energy can be stored in the form of capacitance by supercapacitors via storing positive and negative charges on their spaced opposing electrodes. Supercapacitors falls into two categories based on different capacitance storage principles: “Electric double layer capacitors (EDLC)” and “Faraday quasi capacitors” (or named pseudocapacitors) (Luryi, 1988; Pekala, 1989; Conway et al., 1997; Frackowiak, 2007; Chmiola et al., 2010; Stoller et al., 2011; Paek et al., 2013; Liang, 2015; Zhu et al., 2016; Chen et al., 2019; Ghosh et al., 2019; Xue et al., 2019; Ghosh et al., 2020; Miao et al., 2021a; Song et al., 2021a; Wang et al., 2021a; Miao et al., 2021b; Song et al., 2021b; Fu et al., 2021; Long et al., 2021; Mansuer et al., 2021; Sakita et al., 2021; Xin et al., 2021; Zhang et al., 2021; Yang et al., 2022a; Du et al., 2022; Mansuera et al., 2022; Qin et al., 2022; Yan et al., 2022; Zheng et al., 2022; Maldonado-Hódar et al., 2000; Haddon, 2002; Lachawiec et al., 2005; Ji et al., 2009; Qin et al., 2009; Li et al., 2011; Lv et al., 2012; Morteza et al., 2015; Mousavi-Khoshdel and Targholi, 2015; Mousavi-Khoshdel et al., 2016; Ping et al., 2016; Wang and Pumera, 2016; Liu et al., 2017; Song et al., 2018; Silva et al., 2020; Kolosov and Glukhova, 2021a; Kolosov and Glukhova, 2021b; Boulanger et al., 2021; Cao et al., 2021; Chakrabarty et al., 2021; Chen et al., 2021; Christians et al., 2021; Song et al., 2021c; Hoa et al., 2021; Nizam et al., 2021; Olabi et al., 2021; Ramirez et al., 2021; Tong et al., 2021; Vermisoglou et al., 2021; Zhai et al., 2021; Zhou et al., 2021; Yang et al., 2022b; Mei, 2022; Rashidi et al., 2022; Teimuri-Mofrad et al., 2022; Liu et al., 2022; Paul1 and Roy, 2021; Basivi et al., 2021; Zhang et al., 2019; Zhai et al., 2011; Rashidi and Yusup, 2020; Yang and Zhou, 2017; Arango et al., 2018; Umezawa et al., 2021; Mandal et al., 2021; Wulandari et al., 2021; Wu et al., 2021; Taer et al., 2021; Kalu-Uka et al., 2022; Yuan et al., 2021; Gopalakrishnan and Badhulika, 2020; Wang et al., 2015; Du et al., 2021; Jin et al., 2019; Hassan et al., 2014; Kakaei et al., 2016; Kumari et al., 2018; Hummers and Offeman, 1958; Becerril et al., 2008; Wang et al., 2009; Kaskela et al., 2010; Liu et al., 2011; Sun et al., 2013; Gao et al., 2014; Long et al., 2015; Baig et al., 2016; Xiao et al., 2016; Li et al., 2017; Tian et al., 2017; Wang et al., 2017; Kaur et al., 2018; Sahoo et al., 2018; Hoang et al., 2019; Tao et al., 2019; Wang et al., 2019; Du et al., 2020; Kakaei et al., 2020; Wang et al., 2021b; Pang et al., 2021; Singh et al., 2021; Tatrari et al., 2021; Zhao et al., 2021; Akin and Zhou, 2022; Balboni et al., 2022; Song et al., 2022b; Elanthamilan et al., 2022; Zhai et al., 2022). As shown in Figure 1, to evaluate the performance of supercapacitors, several Electrochemical analysis methods such as cyclic voltammetry (CV), electrochemical impedance spectroscopy (EIS) and galvanostatic charge-discharge (GCD) test and were widely used. It has been found that important parameters of supercapacitors (e.g., operating potential window, equivalent series resistance and capacitance), are strongly depends on their electrode materials (Frackowiak, 2007; Ghosh et al., 2019). As shown in Figure 1A, capacitance (C) of supercapacitors could be obtained from CV curves using Eq. 1, where ΔV is the total potential bias of the voltage window, dt is the sampled span of time, i is a sampling current. If CV curves are close to ideal rectangles, C could be calculated using Eq. 2, where ν is the potential scan rate. Gravimetric capacitance (Cm) is an important parameter for supercapacitors, when CV curves of a supercapacitor show the characteristics of a non-ideal rectangle (e.g., have pseudocapacitive bulging peaks), Cm of the electrode materials could be calculated from enclosed area between the current and the voltage in the CV curve using Eq. 3, where m is electroactive materials’ mass in electrodes, I(V) denotes the density of response current, Vc and Va are CV curve’s integration limits (Wei et al., 2010). As shown in Figure 1B, the GCD curve can calculate the Cm of the electrode material (4), where Δt is the time of discharge, m is the active materials’ mass, ΔV is the change of voltage which is excluded voltage drop (IR drop) in the process of discharge, I is the current of discharge. After assembling into a symmetrical supercapacitor, the capacitance of single electrode (Cs) can be calculated through Eq. 5, where m represents the gross mass of active materials on two working electrodes, but I, t, and V reflect same meaning as Eq. 4. Volumetric capacitance (CV) and areal-specific capacitance (CA), which can be obtained according to the volume or the area of the single electrode through Supplementary Eqs S1, S2, are also important. Since the thickness of electrode materials are difficult to be measured accurately till now, compare to Cm much fewer studies reported CV or CA (Li et al., 2017; Zhao et al., 2021). EIS provides focused analysis of the resistive behavior. Solution resistance, charge transfer impedance, could be calculated from Nyquist plots’ altofrequency part (Figure 1C), while low frequency part of the curve shows the ion diffusion impedance. In addition, the Bode phase diagrams (Figure 1D) show phases angle as a function of frequency. The capacitance’s real and imaginary parts (C′(ω) and C″(ω)) can be calculated from the impedance data based on Eq. 6, where complex impedance is Z(ω), its real and imaginary parts are Z′(ω) and Z″(ω), and the angular frequency is ω (Taberna et al., 2003). There is a Eq. 7 can also be used to calculate Cm, where m is active materials’ mass in each electrode, f is the operating frequency, and Z″ and Z′ are the imaginary and real parts of total resistance (Cheng et al., 2011). Besides, supercapacitors’ energy density (E), power density (P) and coulombic efficiency (η) can be estimated by Eqs 8–10, where ∆V, Δtd, and Δtc denote the voltage window, discharge time and charge time (Mansuera et al., 2022).
FIGURE 1. An expression of a typical curves of (A) CV and (B) GCD, (C) Nyquist plots, (D) Bode phase diagram (Mansuera et al., 2022); equations for calculations of capacitances (C, Cm, Cs, C′, C″), energy and power density (E, P) as well as coulombic efficiency (η) (Taberna et al., 2003; Wei et al., 2010; Cheng et al., 2011).
Electrode material is a crucial component in supercapacitors. With fascinating characteristics of environmental benignity and abundance, carbonaceous materials equipped with extensive applicability and outstanding physicochemical stability have been one kind of the most popular materials that applied in supercapacitors nowadays. Since a satisfactory capacitance can be generated due to the high ionic accessible surface area of the electrode material in a certain electrolyte, carbonaceous materials with the large surface area have been extensively explored over the past two decades (Chen et al., 2020; Shao et al., 2020). Different dimensional (0D-3D) carbonaceous materials, such as zero-dimensional (0D) carbon quantum dots (CQDs) and carbon nanospheres, one-dimensional (1D) carbon nanotube (CNTs) and nanowires, two-dimensional (2D) graphenes and carbon nanosheets, three-dimensional (3D) carbide-derived carbons (CDCs), activated carbons (ACs) and carbon aerogels, have been developed and tested in supercapacitors’ electrode (Luryi, 1988; Pekala, 1989; Conway et al., 1997; Stoller et al., 2011; Paek et al., 2013; Zhu et al., 2016; Chen et al., 2019; Xue et al., 2019; Ghosh et al., 2020; Miao et al., 2021a; Song et al., 2021a; Wang et al., 2021a; Miao et al., 2021b; Song et al., 2021b; Fu et al., 2021; Long et al., 2021; Mansuer et al., 2021; Sakita et al., 2021; Xin et al., 2021; Zhang et al., 2021; Yang et al., 2022a; Du et al., 2022; Mansuera et al., 2022; Qin et al., 2022; Yan et al., 2022; Zheng et al., 2022).
The main energy storage mechanism of supercapacitors prepared from 3D carbonaceous materials such as ACs and carbon aerogels is EDLCs (Fu et al., 2021). However, it has been found that low-demensional materials (e.g., 1D CNTs and 2D graphene) themselves’ properties can also contribute capacitance to the supercapacitor system. Thus, quantum capacitance (CQ) has been formally proposed (Luryi, 1988). CQ originates from electronic density of states (DOS) which close to the Fermi level of electrode materials. Doping, functionalization and mechanical deformation can be used to adjust materials’ electronic structure and CQ (Stoller et al., 2011). As shown in Supplementary Figure S1, Paek et al. (2013) evaluated interfacial capacitance between graphene and [BMIM][PF6] as a function of applied potential (Фa). Total capacitors (CT) at the interface of graphene/[BMIM][PF6] is give as a range of electric double layer capacitance (CD) and the quantum capacitors (CQ) of graphene. When the DOS of the electrode material is relatively small, the effect of CQ can be ignored.
Nevertheless, insufficient energy density still have being an important defect for carbonaceous materials, which shortens the long-term availability of the energy provided by supercapacitors and fall behind practical demands (e.g., in electric vehicles and rail transit) (Chen et al., 2019). Previous studies have shown that the carbonaceous materials’ performance can be improved via surface functionalization, and it was found that surface-active groups of carbonaceous supercapacitor materials is mechanically important, several recently reports had started to study the surface situations of carbon materials via quantum-chemical computing (Zhu et al., 2016). Recently, considerable progresses have been made in heteroatom doped and/or surface functionalized carbonaceous materials which have potential for improved ions accessibility, material conductivity, faradaic feature, supercapacitive activities as well as energy storage abilities (Ghosh et al., 2020).
Several reviews related with carbonaceous electrode materials have been reported already (Frackowiak, 2007; Wang et al., 2021b; Akin and Zhou, 2022; Song et al., 2022b; Zhai et al., 2022), which gave almost the complete picture of the technologies for supercapacitor. However, there are few reviews that comprehensively introduce the surface functionalization of electrode materials. This mini-review aims to focus recent developments in the supercapacitor electrodes made from carbonaceous materials. The preparation, surface functionalization as well as electrochemical performances of different carbonaceous electrode materials were compared and classified. The challenges and potential prospects for future innovations of carbonaceous electrode materials were also discussed.
Many sources of carbonaceous materials. Various natural resources, such as biomass materials, crude oil, nature gas and coal, artificial polymer such as polyvinylidene chloride (PVDC), can be used as precursors for carbonaceous materials. There are various methodologies for converting carbonic precursors into carbonaceous materials, such as physical or chemical vapour deposition, pyrolytic heating as well as organic redox methodologies (Chmiola et al., 2010; Pekala, 1989; Conway et al., 1997; Maldonado-Hódar et al., 2000; Haddon, 2002; Lachawiec et al., 2005; Ji et al., 2009; Qin et al., 2009; Li et al., 2011; Lv et al., 2012; Liang, 2015; Morteza et al., 2015; Mousavi-Khoshdel and Targholi, 2015; Mousavi-Khoshdel et al., 2016; Ping et al., 2016; Wang and Pumera, 2016; Liu et al., 2017; Song et al., 2018; Silva et al., 2020; Kolosov and Glukhova, 2021a; Boulanger et al., 2021; Cao et al., 2021; Chen et al., 2021; Christians et al., 2021; Hoa et al., 2021; Nizam et al., 2021; Olabi et al., 2021; Ramirez et al., 2021; Zhai et al., 2021; Zhou et al., 2021; Yang et al., 2022b; Mei, 2022; Rashidi et al., 2022; Teimuri-Mofrad et al., 2022; Kolosov and Glukhova, 2021b; Song et al., 2021c; Vermisoglou et al., 2021; Tong et al., 2021; Chakrabarty et al., 2021; Liu et al., 2022; Paul1 and Roy, 2021; Basivi et al., 2021; Zhang et al., 2019; Zhai et al., 2011; Rashidi and Yusup, 2020; Yang and Zhou, 2017; Arango et al., 2018; Umezawa et al., 2021; Mandal et al., 2021; Wulandari et al., 2021; Wu et al., 2021; Taer et al., 2021; Kalu-Uka et al., 2022; Yuan et al., 2021; Gopalakrishnan and Badhulika, 2020; Wang et al., 2015; Du et al., 2021; Jin et al., 2019; Hassan et al., 2014; Kakaei et al., 2016; Kumari et al., 2018; Kaur et al., 2018; Du et al., 2020; Hoang et al., 2019; Sahoo et al., 2018; Kakaei et al., 2020; Gao et al., 2014; Baig et al., 2016; Kaskela et al., 2010; Liu et al., 2011; Balboni et al., 2022; Tao et al., 2019; Singh et al., 2021; Hummers and Offeman, 1958; Becerril et al., 2008; Wang et al., 2009; Long et al., 2015; Pang et al., 2021; Wang et al., 2017; Sun et al., 2013; Tian et al., 2017; Wang et al., 2019; Elanthamilan et al., 2022; Xiao et al., 2016; Tatrari et al., 2021). Through controlling the carbonization process and conditions, carbonaceous materials with different electrochemical performance were prepared. For instance, by altering the heating temperature, pressure and atmosphere in pyrolysis process, the properties of resulted carbonaceous materials can be adjusted. Carbonization process of carbonaceous materials is usually accompanied with graphitization which realize the orderly transformation of carbon atoms from a thermodynamically unstable turbostratic framework to a graphite structure. The degree of graphitization alters the performance of electrode material including comprehensive resistance, power delivery, energy efficiency, power density, cyclic stability and rate capacity. As the graphitization degree increased, carbonaceous materials’ conductivity will be improved, but their relative porous structure may get distorted along with decreased specific area (Zhang et al., 2017).
CQDs are a typical class of 0D carbonaceous materials, which are composed of dispersed, quasi-spherical and ultrafine carbon nanoparticles with an average size below 10 nm (Hassan et al., 2014). CQDs can be obtained through different carbon sources (e.g., graphene feathers, egg, garlic, citric acid, etc.) via different methods (Kakaei et al., 2016; Kaur et al., 2018; Kumari et al., 2018; Du et al., 2020).
Application of pure CQDs as electrode materials for supercapacitors has not received much attention. But more and more CQDs based composites show excellent electrochemical performance for supercapacitors. Because of p–p conjugation, functional groups and strong electrostatic interaction of CQDs, a number of molecules such as organic molecules, nanoparticles, and polymers are able to connect to the surface of CQDs. For example, Hoang et al. (2019) combined CQDs and reduced graphene oxide (RGO) with a 1:2 mass ratio, this CQDs/RGO composite revealed high specific capacities (maximum capacitance of 278 F/g at the current density of 0.2 A/g) as electrode material for supercapacitors. As shown in Supplementary Figure S2, Sahoo et al. (2018) prepared a nanostructured CQDs/NiS composite through a facile hydrothermal method. This composite material exhibited good capacitive performance with specific capacity of 880 F/g at the current density of 2 A/g (Sahoo et al., 2018). Kakaei et al. (2020) presented a CQDs/PANI composite using CQDs as the shell and PANI as the core. This composite material showed good electrochemical performance with specific capacitance of 264.6 F/g at the current density of 2.5 A/g (Kakaei et al., 2020).
The diameter of other 0D carbonaceous materials usually larger than 50 nm. Compare with solid nanospheres which have limited electrochemical performances, hollow nanospheres have gained extensive interest. For instance, Gao et al. (2014) reported carbonaceous hollow spheres with a huge specific surface area (2,106.0 m2/g) based on fermented rice. At a high current density of 15 A/g, this materials exhibited 219 F/g specific capacitance (Gao et al., 2014).
On account of their structural alignment, CNTs can be divided into two groups: single-walled (SWCNTs) and multi-walled carbon nanotubes (MWCNTs). A SWCNT is composed of a cylindrical-shaped graphene sheet with uniphase and outer phase bonds of sp2 carbon atoms (Haddon, 2002). The MWCNTs is generated by the co-dimensional arrangement of the SWCNTs (Baig et al., 2016). Kaskela et al. (2010) synthesized SWCNTs via thermal decomposition of ferrocene vapor in the atmosphere of carbon monoxide. Liu et al. (2011) pyrolyzed polypropylene into MWCNTs and hydrogen-rich gas. Balboni et al. (2022) prepared vertically aligned CNTs arrays (ACNTA) through photothermal chemical vapour deposition method, and ACNTA-polyaniline/polydimethylsiloxane electrodes were fabricated for a flexible supercapacitor which showed good cyclic stability and electrochemical properties. Its capacitance was able to maintain 80% at different bending angles (Balboni et al., 2022).
Carbon nanofibers (CNFs) are another kind of widely studied 1D carbonaceous materials. One of the most common procedure to synthesize CNFs is electrochemical spinning. Tao et al. (2019) synthesized electrospun CNFs based on waste walnut shells via the procedure of liquefying, electrospinning and carbonizing. Singh et al. (2021) obtained self-standing CNFs at different temperatures, they found the 800°C heat treated CNF exhibits largest specific capacitance (196.63 F/g at a current density of 1 A/g).
Graphene is like a special kind of graphite, but only a single layer atomic thick. Pure graphene is a carbonaceous material with excellent electrical and thermal conductivity, strong physical strength and good chemical stability. Embed these properties into supercapacitors with good cyclic stability and high power density would be advantageous. Many nanocomposites with heteroatoms or functional groups can be formed based on graphene (Olabi et al., 2021). Wang et al. (2009) prepared graphene materials from graphene oxide sheets that synthesized through modified Hummers’ method (Hummers and Offeman, 1958; Becerril et al., 2008) using hydrazine reduction to recover the conductive carbon network. They fabricated supercapacitors based on these graphene materials with maximum energy density of 28.5 Wh/kg, power density of 10 kW/kg and specific capacitance of 205 F/g at the current density of 100 mA/g (Wang et al., 2009). Long et al. (2015) presented the preparation of densely layer-stacking porous carbon nanosheets via hydrothermal treatment of the fungus and the later carbonization process. These 2D materials have 1,103 m2/g surface area, and exhibit a large volumetric capacitance of 360 F/cm3 at 200 mV/s scan rate (Long et al., 2015).
Carbon aerogels usually can be obtained from organic aerogel through pyrolysis process. These highly porous carbonaceous materials exhibit good thermal, electrical and mechanical properties, and has greater compressibility and adsorption. Structure and properties of carbon aerogels can be controlled by different preparation methods, which let carbon aerogels become one of potential candidate materials for supercapacitors (Pekala, 1989; Conway et al., 1997). Liang (2015) investigated the impact of heating rate and pyrolysis temperature on carbon aerogels’ morphologies and electrochemical properties. It was found that carbon aerogels prepared by carbonization in nitrogen at 850°C have large specific capacitance, and the cyclic voltammetry curves are close to an ideal rectangle, with good capacitance characteristics and reversibility (Liang, 2015).
CDCs are a late-model type of porous carbonaceous type of carbon materials obtained through taking carbide as a precursor and trying to remove metal atoms or non-metal atoms in the lattice layer by layer, leaving a skeleton carbonaceous structure (Meng et al., 2004). By adjusting the temperature, atmosphere composition, reaction time and other parameters during the reaction process, nano-scale regulation can be achieved to obtain CDCs coatings with different structures (Chmiola et al., 2010). CDCs have become a kind of potential electrode materials for supercapacitor (Chmiola et al., 2010; Christians et al., 2021). Recently, Pang et al. (2021) obtained CDC@NC using polydopamine as the nitrogen and carbon source. The specific surface area of CDC@NC was up to 1,191 m2/g. As a electrode material, the specific capacitance of CDC@NC reached 255 F/g at 0.5 A/g, and 193 F/g at 20 A/g (Pang et al., 2021).
ACs are prepared from carbon-containing raw materials through pyrolysis and activation processing. These carbonaceous materials usually have developed pore structures and high surface area (Nizam et al., 2021). Many supercapacitor electrodes based on ACs exhibit excellent performances not only owing to their highly porous structure but also due to their redox behavior (Boulanger et al., 2021; Rashidi et al., 2022). Recently, AC materials have been prepared from different biomass materials (e.g., coconut shell, cotton stalk, algae, saussurea involucrata stalk) (Sun et al., 2013; Gao et al., 2014; Tian et al., 2017; Wang et al., 2017; Wang et al., 2019). Elanthamilan et al. (2022) reported a mesoporous ACs made through chemical activation of dried fruit biomass at various temperatures. Their further fabricated symmetric supercapcitor exhibited an remarkable 11.11 Wh/kg energy density with 333.3 W/kg power density (Elanthamilan et al., 2022).
Carbon foams, which could possess good thermal stability, high mechanical strength, open cell structure, adjustable electrical and thermal conductivities, are mainly prepared via three routes: Compaction and exfoliation of graphite, template carbonization of carbon precursor as well as blowing of carbon precursors such as pitches with subsequent carbonization (Li et al., 2011). Hierarchical carbon foams with suitable surface functional groups and considerable surface area are good candidates for advanced supercapacitor electrode materials (Lv et al., 2012; Yang et al., 2022b). Xiao et al. (2016) reported a highly compressible, hydrophilic and free-standing N-doped carbon foam which could not only endure a compressive strain of 80% but also achieve 343 mF/cm2 and 52 F/g specific capacitance at 1 mA/cm2 current density.
Comparisons of different carbonaceous materials for supercapacitor are listed in Table 1. From the view of industrial production, although aerogel, CDCs, CQDs, CNTs, and graphene materials exihited controllable pore structures, large surface area and high chemical stability, all these materials, which may be good candidates for preparing on-chip microsupercapacitors, require time-consuming, complicated and expensive producing process, which probably hinder their large-scale application. On the other hand, ACs have been reported as the most preferable carbonaceous electrode materials (Wang et al., 2015). To further reduce the cost of industrial production of carbonaceous electrode materials, low cost precursors should be preferred. Carbonaceous wastes such as plastic wastes, agricultural biomass wastes, industrial wastes, medical wastes and municipal wastes have great recycling value. Rational design of chemical processes for converting carbonaceous waste into carbonaceous electrode materials can also reduce potential carbon dioxide emissions (Tatrari et al., 2021). On the other hand, it is essential to probe new methods to further enhance electrochemical performances of carbonaceous electrode materials.
The nature of carbonaceous electrode materials can be monitored and adjusted through heteroatom doping and surface functionalization with different methodologies. For instance, the position and performances of surface functional groups can be adjust via thermal heating or chemical treatments. On the other hand, doping of heteroatoms (e.g., O, N, F, S, B, P and metallic atoms into carbon skeleton will often adjust carbonaceous materials’ wettability, electroconductibility and capacitance. As shown in Supplementary Figures S7, S8, those surface functionalization can be either derived from heteroatoms containing precursors or achieved through post-treatment/activation process (Teimuri-Mofrad et al., 2022; Lachawiec et al., 2005; Maldonado-Hódar et al., 2000; Ji et al., 2009; Ramirez et al., 2021; Liu et al., 2017; Qin et al., 2009; Mei, 2022; Ping et al., 2016; Zhai et al., 2021; Hoa et al., 2021; Chen et al., 2021; Cao et al., 2021; Zhou et al., 2021; Wang and Pumera, 2016; Mousavi-Khoshdel et al., 2016; Mousavi-Khoshdel and Targholi, 2015; Silva et al., 2020; Song et al., 2018; Morteza et al., 2015; Kolosov and Glukhova, 2021a; Kolosov and Glukhova, 2021b; Song et al., 2021c; Vermisoglou et al., 2021; Tong et al., 2021; Chakrabarty et al., 2021; Liu et al., 2022; Paul1 and Roy, 2021; Basivi et al., 2021; Zhang et al., 2019; Zhai et al., 2011; Rashidi and Yusup, 2020; Yang and Zhou, 2017; Arango et al., 2018; Umezawa et al., 2021; Mandal et al., 2021; Wulandari et al., 2021; Wu et al., 2021; Taer et al., 2021; Kalu-Uka et al., 2022; Yuan et al., 2021; Gopalakrishnan and Badhulika, 2020; Wang et al., 2015; Du et al., 2021; Jin et al., 2019).
Modification of aerogels is a major trend for carbon aerogels. At present, the modification methods of aerogels mainly include surface modification, doping and composite (Lachawiec et al., 2005; Teimuri-Mofrad et al., 2022). Maldonado-Hódar et al. (2000) discovered that the Co, Ni, doped carbon aerogel system undergoes homomorphic structural reorganization, from the original nano-continuous network structure to the nano-graphite structure. Ji et al. (2009) reported a Ni2+ doped carbon aerogel, which has specific surface area of 441.8 m2/g. At 50 mA/g current density, this material achieve 227.3 F/g specific capacitance, which is higher than that of pure carbon aerogels (93 F/g) and Fe3+ doped carbon aerogel (180.5 F/g) (Ji et al., 2009). Ramirez et al. (2021) fabricated carbon aerogels with various Pd contents. It was found that Pd doping resulted in significantly enhanced power and energy density compared to undoped carbon aerogels. Liu et al. (2017) incorporated the surface of carbon aerogel with size-selected and dispersed WO3 nanoparticles using solvent-immersion process and calcination treatment. This modified carbon aerogels show 1055 F/g specific capacitance at 5 mV/s scanning rate (Liu et al., 2017). Qin et al. (2009) prepared a nanocomposite named ANC/ACA which is composed of carbon aerogel (ACA) and activated nitrogen-enriched carbon (ANC). At the scanning rate of 1 mV/s, ANC/ACA with composite ratio of 12:1 exhibits 312.8 F/g specific capacitance, higher than that of ACA (103.4 F/g) and ANC (230.1 F/g) (Qin et al., 2009).
Conventional carbon aerogels based on phenolic resins are expensive and generate toxic pollutants during pyrolysis. Recently, several biomass carbon aerogels have been developed. Biomass resources are usually rich in oxygen and nitrogen elements, these characteristics could be used for self-activation to achieve the effect of self-doping (Mei, 2022). Ping et al. (2016) reported a carbon fiber aerogel based on natural cotton. The specific surface area of these carbon aerogels can be adjusted from 1,536 to 2,436 m2/g via controlling the KOH amount in activation process (Ping et al., 2016). Zhai et al. (2021) reported a series of N self-doped carbon aerogel (NSCA) using chitin as carbon and nitrogen sources through microwave hydrothermal reaction and carbonization with Zinc chloride as the activating and dehydrating agent. NSCA-1000 exhibited 249.4 and 164.9 F/g specific capacitances at the current densities of 1.0 and 10 A/g (Zhai et al., 2021). Hoa et al. (2021) combined reduced graphene oxide (RGO) with NiCo2S4 to prepare RGO/NiCo2S4 aerogel, which have good electrical conductivity and high porosity. RGO/NiCo2S4 aerogel exhibit a capacitance of 813 F/g at 1.5 A/g current density. The asymmetric supercapacitor based on RGO/NiCo2S4 aerogel reaches 40.3 Wh/kg energy density with 375.0 W/kg power density (Hoa et al., 2021). Chen et al. (2021) synthesized S- and N-doped 3D porous graphene aerogels (NS-3DPGHs) with thiourea as a reducing agent and additive. The NS-3DPGH-150 sample exhibits 412.9 F/g specific capacitance with remarkable cycling stability at 0.5 A/g current density (Chen et al., 2021). Cao et al. (2021) Reported N, Fe co-doped (FeNC) aerogel, which exhibit up to 3,103 m2/g specific surface area and 170–264 F/g specific capacitances at the current density of 0.5 A/g. With the increase of iron content, the cycling stability and capacitance performance of FeNC aerogel was decreased (Cao et al., 2021). Zhou et al. (2021) developed a facile way to combine carbon aerogels with MnOx nanoparticles and prepare carbon aerogel/MnOx composite. As shown in Supplementary Figure S3, MnOx nanoparticles grow consistently on carbon aerogel surface. The uniform growth of MnOx nanoparticles and cross-linked 3D structure of carbon aerogel are advantageously to the composite’s electrochemical performances. Specific capacitance of carbon aerogel/MnOx reached 557 F/g at 1 A/g current density (Zhou et al., 2021).
Both CNTs and Graphene are low-dimensional electrode material for supercapacitor. Theoretically, CQ of CNTs graphene maybe improved by suitable functionalization (Mousavi-Khoshdel and Targholi, 2015; Mousavi-Khoshdel et al., 2016; Wang and Pumera, 2016). Silva et al. (2020) employed density functional theory (DFT) calculations and molecular dynamics atomistic simulations to determine CT. Their results exhibited that doped electrodes present variations in CQ which is in the range of 0–200 μF/cm2, because of the changes in the pristine graphene’s electronic structure. However, neither vacancies nor doping atoms has appreciable influence on CD (Silva et al., 2020). Song et al. (2018) explored the CQ of graphene oxide (GO) through DFT calculations. As shown in Supplementary Figure S4, it was found that intoducing oxygen-containing groups can enhance the total density of states near the Fermi level. Moreover, increasing the concentrations of hydroxy and epoxy groups will improve CQ of GO (Song et al., 2018).
Mousavi-Khoshdel M. investigated the impact of doping (P, S, Si) and co-doping (P N, S N, Si N) of graphene on structural parameters, electronic properties as well as CQ via DFT and generalized-gradient approximation (GGA). The obtained results indicated that in comparison with pristine graphene sheets, codoping of graphene generates new electronic states and accumulates larger quantity of electrical charge on co-doped graphene sheets, which will enhance their quantum capacitance consequently (Morteza et al., 2015). As shown in the Supplementary Figure S5, Kolosov and Glukhova (2021a) predicted ways to improve the performances of the electrode material on the base of pillared graphene (PGR) and boron clusters B12 through first-principle DFT method. Specific charge density calculation proved the symmetry of positive and negative bias at 5.13 wt. % (two B12 clusters). Moreover, branch asymmetry was observed when the increase in boron concentration, therefore during the addition of B12 clusters, the electronic states density is increased, the electrode conductivity will probably be increased. The electronic properties and CQ of SWCNTs decorated with B12 clusters were also explored. As shown in Supplementary Figure S6, it was found that the addition of B12 clusters can improved the CQ of SWCNTs (Kolosov and Glukhova, 2021b).
Numerous reported experimental studies also demonstrated that functionalizing graphene and carbon nanotubes can effectively improve their electrochemical performances. Song et al. (2021c) synthesized N-doped graphene sheets (NGS) through a solid-state microwave-mediated process. Subsequently, experimental electrodes employing NGS were fabricated. At 0.5 A/g current density, the electrode’s specific capacitance reached 208.17 F/g (Song et al., 2021c). Vermisoglou et al. (2021) utilized fluorographene, which was uniformly linked with amino acid moieties on graphene’s both sides. By adding non-toxic pore formers and adjusting conditions of reactions, the hierarchical porosity and optimal degree of functionalization were achieved. At 0.25 A/g current density, this material exhibited high capacitance of about 390 F/g. The assembled supercapacitor device was tested for 30,000 cycles of charge-discharge at a current density of 2 A/g, showing 82.3% capacitance retention (Vermisoglou et al., 2021). Tong et al. (2021) synthesized poly-3,4-ethylenedioxythiophene (PEDOT) on graphene nanosheet-deposited open-pored polyurethane (PU) sponge by vapor-phase polymerization (VPP) way to prepare porous electrodes. Under 0.1 mA/cm2 current density, the obtained electrodes showed high areal specific capacitance of 798.2 mF/cm2 (Tong et al., 2021). Chakrabarty et al. (2021) prepared RGO sheets of different weights decorated with CeO2/Ce2O3 quantum dots (CRGO3). In tests, CRGO3 showed a capacitance of 1027 F/g at 1 A/g (Chakrabarty et al., 2021). Liu et al. (2022) prepared graphene hydrogel (GH) nanocomposites with graphitic carbon nitride (g-C3N4) quantum dots (CNQDs), in which GH are decorated with CNQDs through a 3D layered interconnected configuration. The obtained CNQ/GH nanocomposites exhibited 243.2 Fg−1 specific capacitance at 0.2 Ag−1 current density (Liu et al., 2022). Paul and Roy (2021) developed a spray pyrolysis process for the synthesis of radially grown nanoporous brush-like CNTs on carbon cloth (CC) substrates with boron (B) and nitrogen (N) simultaneously co-doped and a composite BNCNT-CC was obtained. Based on BNCNT-CC, highly flexible all-solid-state symmetric supercapacitors was further integrated, which have high capacitance retention (86.4%) after 5,000 charge-discharge cycles at 5 mA/cm2 (Paul1 and Roy, 2021). Basivi et al. (2021) synthesized a novel composite, N-doped MWCNT/carboxymethyl cellulose (N-MWCNT/CMC) for electrodes of supercapacitors. The synthesized composite exhibited 274 F/g specific capacitance at 2 A/g. After 4,000 cycles of the composite electrode, the cycling stability is as high as 96%, which is beneficial for supercapacitor applications (Basivi et al., 2021).
Hydrophobic nature of unmodified ACs coursed weak infiltration of electrolyte to electrode, and ion-accessible surface limitation (Zhang et al., 2019). Zhai et al. (2011) concluded that porous structure, conductivity and surface properties optimization are required to ensure maximum capacitance of ACs. Functionalization of ACs as supercapacitor electrode materials has attracted more and more attentions nowadays (Rashidi and Yusup, 2020) Yang and Zhou (2017) reported N atoms inclusion into the structure of ACs to provide an extra redox reaction for pseudocapacitor. Arango et al. (2018) reported a HNO3 modified ACs with 1.8 wt. % N content, compared with only 0.4 wt. % of the unmodified AC. After 1,000 operating cycles at 0.27 A/g, this modified AC maintains 75.3% capacitance retention (Arango et al., 2018). Rashidi and Yusup (2020) sorted out recent researches about N modification on different ACs. From 2015 to 2020, nitric acid, ammonia and poly (ethylene imine) were reported as post-modification chemical agents to functionalize the surface of ACs. After functionalization, the capitance value of obtain nitrogen-functionalized ACs are at least doubled compare with unmodified AC. Melamine and urea are often used as in situ nitrogen doptant, and biomass raw materials (e.g., swim bladder, corncob, sakura et al.) can be used to prepare nitrogen-self doped ACs for supercapacitors (Rashidi and Yusup, 2020). Umezawa et al. (2021) prepared B-doped ACs based on B-based covalent organic frameworks (COF-5). The supercapacitor electrodes made from it show 15.3 μF/cm2 specific capacitance at 40 mA/g, which is much larger than that of pristine ACs (Umezawa et al., 2021). Mandal et al. (2021) prepared a novel composite of AC/MWCNTs-ZnFe2O4, which exhibit 613 F/g specific capacitance at 5 mV/s scan rate, and after 10,000 cycles, 91% of the capacitance can be retained. Wulandari et al. (2021) used CaCl2 as an active agent to prepare AC from fruit bunches, after carbonization and activation processes, the product was further modified with 1 mol/L nitric acid (HNO3). Wu et al. (2021) developed N, O, S co-doped hierarchical AC, which has 2,864 m2/g specific surface area with 1.461 m3/g total pore volume. This material exhibits 270 F/g specific capacitance at 1 A/g with remarkable long-term cycling stability and 98% capacity retention after 10,000 cycles (Wu et al., 2021). Taer et al. (2021) fabricated a biochar AC using reproducible and environmentally friendly branch and leaf (Syzygiumoleana). The feedstock is converted into biochar AC using ZnCl2 impregnation and one-stage integrated pyrolysis. This biochar AC provides 138.5 F/g specific capacitance at a low scan rate of 1 mV/s in 1 mol/L H2SO4 aqueous electrolyte (Taer et al., 2021). Kalu-Uka et al. (2022) convert termite biomass into AC using KOH as the activator through one-process carbonization activation procedure. The specific capacitance of the best AC is 91.76 F/g at 0.5 A/g in 1 mol/L H2SO4, while its specific capacitance achieved 62.35 F/g at 0.5 A/g in 1 mol/L EMImBF4 (Kalu-Uka et al., 2022). Yuan et al. (2021) reported N-doped AC based on rice paddies using atmospheric pressure blasting, activation and N doping process. The prepared N-doped AC has maximum 417 F/g specific capacitance at 1 A/g (Yuan et al., 2021). Gopalakrishnan and Badhulika (2020) reviewed a self-doped heteroatom ACs for supercapacitors. The impacts of doping (N, S, P) on electrochemical performance were highlighted in their review. They concluded that N doped ACs are beneficial in improving conductivity and hydrophilicity. S doped ACs result in electronic reactivity enhancement of carbon and increase pseudocapacitive contribution. P doped ACs enlarge operating potential of cells thus increase energy density. Dual (N, S or N, P) or multi-heteroatom (N, S, P) doped ACs have synergistic effects of dopants which may yield superior performance (Gopalakrishnan and Badhulika, 2020).
This review proposes a brief description about the operating principle and evaluation parameters for supercapacitor. Since both EDLCs and pseudocapacitors occur on surface electrodes, carbonaceous electrode materials’ surface strongly affect the electrochemical performances of supercapacitor, which could be further improved through heteroatom doping and surface functionalization. Theoretically, CQ of CNTs and graphene could be improved via heteroatom doping, which were demonstrated by experimental studies. As a major trend for carbon aerogels and ACs, surface modification, doping and composite can not only improve their optimization of pore size and structure, conductivity and surface properties, but also introduce extra pseudocapacitance to these materials.
Different carbonaceous materials were roughly classified and their preparation process and properties was discussed briefly. Compared with other carbonaceous materials, ACs and aerogels from biomass have drawn more and more attention nowadays owing to its inexpensive cost and renewablity. Especially, the production and utilization of domestic, medical and industrial wastes residues derived carbonaceous materials should be of great significance for controlling environmental pollution and reducing CO2 emissions, which we think will be worth to further studies.
Articles on the manufacturing technology and equipments for carbonaceous materials are relatively rare. Moreover, articles discussed waste gas composition and disposal methods in different carbon material industrialization processes (carbonization, activation) are very few. Which we think should be further study in future.
Conceptualization, YL and JW; methodology, XH and XS; investigation, DJ and SZ; writing-original draft preparation, YL, JL, and YZ; writing-review and editing, YL and JW; supervision,YL and XS; funding acquisition, YL, XS, and YZ. All authors contributed significantly to the manuscript and have read and agreed to the published version of the manuscript.
The authors thank Project of Zhejiang Provincial Department of Education (No. Y202043207), Science and Technology Project of Zhejiang Province (No.2022C01182) and Zhejiang Provincial Natural Science Foundation of China (No. LY19B010003) for supporting.
JL was employed by Hangzhou Plastics Industry Co., LTD.
The remaining authors declare that the research was conducted in the absence of any commercial or financial relationships that could be construed as a potential conflict of interest.
All claims expressed in this article are solely those of the authors and do not necessarily represent those of their affiliated organizations, or those of the publisher, the editors and the reviewers. Any product that may be evaluated in this article, or claim that may be made by its manufacturer, is not guaranteed or endorsed by the publisher.
The Supplementary Material for this article can be found online at: https://www.frontiersin.org/articles/10.3389/fenrg.2022.957032/full#supplementary-material
Akin, M., and Zhou, X. (2022). Recent advances in solid-state supercapacitors: From emerging materials to advanced applications. Int. J. Energy Res. 46, 10389–10452. doi:10.1002/er.7918
Arango, D. I., Zapata-Benabithe, Z., Arenas, E. C., and Perez-Osorno, J. C. (2018). Influence of surface modification with nitric acid on electrochemical performance of agroindustrial waste-based activated carbon. J. Mat. Sci. Mat. Electron. 29, 15557–15569. doi:10.1007/s10854-018-9132-y
Baig, Z., Mamat, O., and Mustapha, M. (2016). Recent progress on the dispersion and the strengthening effect of carbon nanotubes and graphene-reinforced metal nanocomposites: A review. Crit. Rev. Solid State Mater. Sci. 43, 1–46. doi:10.1080/10408436.2016.1243089
Balboni, R. D. C., Maron, G. K., Masteghin, M. G., Tas, M. O., Rodrigues, L. S., Gehrke, V., et al. (2022). An easy to assemble PDMS/CNTs/PANI flexible supercapacitor with high energy-to-power density. Nanoscale 14, 2266–2276. doi:10.1039/d1nr06914d
Basivi, P. K., Ramesh, S., Kakani, V., Yadav, H. M., Bathula, C., Afsar, N., et al. (2021). Ultrasonication-mediated nitrogen-doped multiwalled carbon nanotubes involving carboxy methylcellulose composite for solid-state supercapacitor applications. Sci. Rep. 11, 9918. doi:10.1038/s41598-021-89430-x
Becerril, H. A., Mao, J., Liu, Z., Stoltenberg, R. M., Bao, Z., and Chen, Y. (2008). Evaluation of solution-processed reduced graphene oxide films as transparent conductors. ACS Nano 2, 463–470. doi:10.1021/nn700375n
Boulanger, N., Skrypnychuk, V., Nordenström, A., Moreno-Fernández, G., Granados-Moreno, M., Carriazo, Mysyk D. R., et al. (2021). Spray deposition of supercapacitor electrodes using environmentally friendly aqueous activated graphene and activated carbon dispersions for industrial implementation. Chemelectrochem 8, 1349–1361. doi:10.1002/celc.202100235
Cao, Q., Gu, Y. X., Liu, Y. Q., Xiao, L., and Wang, D. H. (2021). Praperation of magnetic Fe, N co-doped nano-sized graphitized carbon aerogel with hierarchical porous structure and its electrochemical and adsorption performance. Chin. Sci. Bull. 66, 4129–4143. doi:10.1360/tb-2021-0396
Chakrabarty, N., Dey, A., Krishnamurthy, S., and Chakraborty, A. K. (2021). CeO2/Ce2O3 quantum dot decorated reduced graphene oxide nanohybrid as electrode for supercapacitor. Appl. Surf. Sci., 137345.
Chen, D. D., Yang, L. J., Li, J. F., and Wu, Q. S. (2019). Effect of self-doped heteroatoms in biomass-derived activated carbon for supercapacitor applications. ChemistrySelect 4 (5), 1586–1595. doi:10.1002/slct.201803413
Chen, Y., Hao, X., and Chen, G. Z. (2020). Nanoporous versus nanoparticulate carbon-based materials for capacitive charge storage. Energy Environ. Mat. 3 (3), 247–264. doi:10.1002/eem2.12101
Chen, Y. N., Hao, H., Lu, X., Li, W., He, G., Shen, W., et al. (2021). Porous 3D graphene aerogel co-doped with nitrogen and sulfur for high-performance supercapacitors. Nanotechnology 32, 195405. doi:10.1088/1361-6528/abdf8d
Cheng, P., Li, T., Yu, H., Zhi, L., Liu, Z., and Lei, Z. (2011). Biomass-derived carbon fiber aerogel as a binder-free electrode for high-rate supercapacitors. Adv. Energy Mat. 1, 356–361.
Chmiola, J., Largeot, C., Taberna, P. L., Simon, P., and Gogotsi, Y. (2010). Monolithic carbide-derived carbon films for micro-supercapacitors. Science 328 (5977), 480–483. doi:10.1126/science.1184126
Christians, H., Brunnengräber, K., Gläsel, J., and Etzold, B. J. (2021). Mesoporous and crystalline carbide-derived carbons: Towards a general correlation on synthesis temperature and precursor structure influence. Carbon 175, 215–222. doi:10.1016/j.carbon.2021.01.003
Conway, B. E., Birss, V., and Wojtowicz, J. (1997). The role and utilization of pseudocapacitance for energy storage by supercapacitors. J. Power Sources 66 (1/2), 1–14. doi:10.1016/s0378-7753(96)02474-3
Du, J., Cao, Q., Tang, X., Xu, X., Long, X., Ding, J., et al. (2021). 3D printing-assisted gyroidal graphite foam for advanced supercapacitors. Chem. Eng. J. 416, 127885. doi:10.1016/j.cej.2020.127885
Du, W., Miao, L., Song, Z., Zheng, X., Lv, Y., Zhu, D., et al. (2022). Kinetics-driven design of 3D VN/MXene composite structure for superior zinc storage and charge transfer. J. Power Sources 536, 231512. doi:10.1016/j.jpowsour.2022.231512
Du, Y., Xiao, P., Yuan, J., and Chen, J. (2020). Research progress of graphene-based materials on flexible supercapacitors. Coatings 109, 892. doi:10.3390/coatings10090892
Elanthamilan, E., Jennifer, S. J., Wang, S. F., and Merlin, J. P. (2022). Effective conversion of Cassia fistula dry fruits biomass into porous activated carbon for supercapacitors. Mater. Chem. Phys. 286, 126188. doi:10.1016/j.matchemphys.2022.126188
Frackowiak, E. (2007). Carbon materials for supercapacitor application. Phys. Chem. Chem. Phys. 9, 1774–1785. doi:10.1039/b618139m
Fu, G., Li, H., Bai, Q., Li, C., and Uyama, H. (2021). Dual-doping activated carbon with hierarchical pore structure derived from polymeric porous monolith for high performance EDLC. Electrochimica Acta 2, 137927. doi:10.1016/j.electacta.2021.137927
Gao, M. R., Xu, Y. F., Jiang, J., and Yu, S. H. (2013). Nanostructured metal chalcogenides: Synthesis, modification, and applications in energy conversion and storage devices. Chem. Soc. Rev. 42, 2986–3017. doi:10.1039/c2cs35310e
Gao, S., Chen, Y., Fan, H., Wei, X., Hu, C., Luo, H., et al. (2014). Large scale production of biomass-derived N-doped porous carbon spheres for oxygen reduction and supercapacitors. J. Mat. Chem. A Mat. 2, 3317–3324. doi:10.1039/c3ta14281g
Ghosh, S., Barg, S., Jeong, S. M., and Ostrikov, K. (2020). Heteroatom doped and oxygen functionalized nanocarbons for high-performance supercapacitors. Adv. Energy Mat. 10 (32), 2001239. doi:10.1002/aenm.202001239
Ghosh, S., Santhosh, R., Jeniffer, S., Raghavan, V., Jacob, G., Nanaji, K., et al. (2019). Natural biomass derived hard carbon and activated carbons as electrochemical supercapacitor electrodes. Sci. Rep. 9, 16315–15. doi:10.1038/s41598-019-52006-x
Gopalakrishnan, A., and Badhulika, S. (2020). Effect of self-doped heteroatoms on the performance of biomass-derived carbon for supercapacitor applications. J. Power Sources 480, 228830. doi:10.1016/j.jpowsour.2020.228830
Hasan Md, M., Islam, T., Shah, S. S., Awal, A., Aziz Md, A., and Ahammad, A. J. S. (2022). Recent advances in carbon and metal based supramolecular technology for supercapacitor applications. Chem. Rec. 22, e202200041. doi:10.1002/tcr.202200041
Hassan, M., Haque, E., Reddy, K. R., Minett, A. I., Chen, J., and Gomes, V. G. (2014). Edge-enriched graphene quantum dots for enhanced photo-luminescence and supercapacitance. Nanoscale 6, 11988–11994. doi:10.1039/c4nr02365j
Hoa, N. V., Dat, P. A., Chi, N. V., and Quan, L. H. (2021). A hierarchical porous aerogel nanocomposite of graphene/NiCo2S4 as an active electrode material for supercapacitors. J. Sci-Adv. Mat. Dev. 6, 569–577.
Hoang, V. C., Nguyen, L. H., and Gomes, V. G. (2019). High efficiency supercapacitor derived from biomass based carbon dots and reduced graphene oxide composite. J. Electroanal. Chem. (Lausanne). 832, 87–96. doi:10.1016/j.jelechem.2018.10.050
Hummers, W. S., and Offeman, R. E. (1958). Preparation of graphitic oxide. J. Am. Chem. Soc. 80, 1339. doi:10.1021/ja01539a017
Ji, Y., He, J., Zhou, J., Dang, W., and Liu, X. (2009). Synthesis and property of carbon aerogel under addition of transition Metal element catalysis. Mater. Science& Technol. 17 (4), 556–560.
Jin, H., Feng, X., Li, J., Li, M., Xia, Y., Yuan, Y., et al. (2019). Heteroatom-doped porous carbon materials with unprecedented high volumetric capacitive performance. Angew. Chem. Int. Ed. Engl. 58 (8), 2419–2423. doi:10.1002/ange.201813686
Kakaei, K., Javan, H., Khamforoush, M., and Zarei, S. A. (2016). Fabrication of new gas diffusion electrode based on carbon quantum dot and its application for oxygen reduction reaction. Int. J. Hydrogen Energy 41, 14684–14691. doi:10.1016/j.ijhydene.2016.06.093
Kakaei, K., Khodadoost, S., Gholipour, M., and Shouraei, N. (2020). Core-shell polyaniline functionalized carbon quantum dots for supercapacitor. J. Phys. Chem. Solids 148, 109753. doi:10.1016/j.jpcs.2020.109753
Kalu-Uka, G. M., Kumar, S., Kalu-Uka, A. C., Vikram, S., Ihekweme, G. O., Ranjan, N., et al. (2022). Production of activated carbon electrode for energy storage application in supercapacitors via KOH activation of waste termite biomass. Waste Biomass Valorization 13, 2689–2704. doi:10.1007/s12649-022-01680-6
Kaskela, A., Nasibulin, A. G., Timmermans, M. Y., Aitchison, B., Papadimitratos, A., Tian, Y., et al. (2010). Aerosol-synthesized SWCNT networks with tunable conductivity and transparency by a dry transfer technique. Nano Lett. 10 (11), 4349–4355. doi:10.1021/nl101680s
Kaur, M., Kaur, M., and Sharma, V. K. (2018). Nitrogen-doped graphene and graphene quantum dots: A review onsynthesis and applications in energy, sensors and environment. Adv. Colloid Interface Sci. 259, 44–64. doi:10.1016/j.cis.2018.07.001
Kolosov, D. A., and Glukhova, O. E. (2021). A new composite material on the base of carbon nanotubes and boron clusters B12 as the base for high-performance supercapacitor electrodes. C (Basel). 7 (1), 26. doi:10.3390/c7010026
Kolosov, D. A., and Glukhova, O. E. (2021). Boron-decorated pillared graphene as the basic element for supercapacitors: An ab initio study. Appl. Sci. (Basel). 1, 3496. doi:10.3390/app11083496
Kumari, A., Kumar, A., Sahu, S. K., and Kumar, S. (2018). Synthesis of green fluorescent carbon quantum dots using waste polyolefins residue for Cu2+ ion sensing and live cell imaging. Sensors Actuators B Chem. 254, 197–205. doi:10.1016/j.snb.2017.07.075
Lachawiec, A. J., Lee, G., and Yang, R. T. (2005). Hydrogen storage in nanostructured carbons by spillover:Bridge-building enhancement. Langmuir 21, 11418–11424. doi:10.1021/la051659r
Lee, Y. W., Kim, B. S., Hong, J., Choi, H., Jang, H. S., Hou, B., et al. (2017). Hierarchically assembled tubular shell-core-shell heterostructure of hybrid transition metal chalcogenides for high-performance supercapacitors with ultrahigh cyclability. Nano Energy 37, 15–23. doi:10.1016/j.nanoen.2017.05.006
Li, H., Hou, Y., Wang, F., Lohe, M. R., Zhuang, X., Niu, L., et al. (2017). Flexible all-solid-state supercapacitors with high volumetric capacitances boosted by solution processable MXene and electrochemically exfoliated graphene. Adv. Energy Mat. 7, 1601847. doi:10.1002/aenm.201601847
Li, S., Tian, Y., Zhong, Y., Xi, Y., Yan, S., Guo, Q., et al. (2011). Formation mechanism of carbon foams derived from mesophase pitch. Carbon 49 (2), 618–624. doi:10.1016/j.carbon.2010.10.007
Liang, X. (2015). Preparation and performance of carbon aerogels for capacitors. J. Henan Inst. Sci. Technol. 43 (6), 36–46.
Liu, D., Tam, T. V., and Choi, W. M. (2022). Facile synthesis of g-C3N4 quantum dots/graphene hydrogel nanocomposites for high-performance supercapacitor. RSC Adv. 12, 3561–3568. doi:10.1039/d1ra08962e
Liu, J., Jiang, Z., Yu, H., and Tang, T. (2011). Catalytic pyrolysis of polypropylene to synthesize carbon nanotubes and hydrogen through a two-stage process. Polym. Degrad. Stab. 96, 1711–1719. doi:10.1016/j.polymdegradstab.2011.08.008
Liu, X., Sheng, G., Zhong, M., and Zhou, X. (2017). Dispersed and size-selected WO3 nanoparticles in carbon aerogel for supercapacitor applications. Mater. Des. 141, 220–229. doi:10.1016/j.matdes.2017.12.038
Long, C., Chen, X., Jiang, L., Zhi, L., and Fan, Z. (2015). Porous layer-stacking carbon derived from in-built template in biomass for high volumetric performance supercapacitors. Nano Energy 12, 141–151. doi:10.1016/j.nanoen.2014.12.014
Long, C., Miao, L., Zhu, D., Duan, H., Lv, Y., Li, L., et al. (2021). Adapting a kinetics-enhanced carbon nanostructure to Li/Na hybrid water-in-salt electrolyte for high-energy aqueous supercapacitors. ACS Appl. Energy Mat. 4, 5727–5737. doi:10.1021/acsaem.1c00566
Lv, Y., Gan, L., Liu, M., Xiong, W., Xu, Z., Zhu, D., et al. (2012). A self-template synthesis of hierarchical porous carbon foams based on banana peel for supercapacitor electrodes. J. Power Sources 209, 152–157. doi:10.1016/j.jpowsour.2012.02.089
Lv, Y., Yang, X., Du, W., Ma, P., Wang, H., Bonnefont, A., et al. (2020). An efficient electrochromic supercapacitor based on solution-processable nanoporous poly{tris[4-(3, 4-ethylenedioxythiophene)phenyl]amine}. ChemSusChem 13, 3844–3854. doi:10.1002/cssc.202000941
Maldonado-Hódar, F. J., Moreno-Castilla, C., Rivera-Utrilla, J., Hanzawa, Y., and Yamada, Y. (2000). Catalytic graphitization of carbon aerogels by transition metals. Catal. Graph. carbon aerogels by transition metals Langmuir 16 (9), 4367–4373. doi:10.1021/la991080r
Mandal, M., Subudhi, S., Alam, I., Subramanyam, B. V. R. S., Patra, S., Raiguru, J., et al. (2021). Facile synthesis of new hybrid electrode material based on activated carbon/multiwalled carbon nanotubes@ZnFe2O4 for supercapacitor applications. Inorg. Chem. Commun. 123, 108332. doi:10.1016/j.inoche.2020.108332
Mansuer, M., Miao, L., Zhu, D., Duan, H., Lv, Y., Li, L., et al. (2021). Facile construction of highly redox active carbons with regular micropores and rod-like morphology towards high-energy supercapacitors. Mat. Chem. Front. 5, 3061–3072. doi:10.1039/d0qm01101k
Mansuera, M., Miao, L., Qin, Y., Song, Z., Zhu, D., Duan, H., et al. (2022). Trapping precursor-level functionalities in hierarchically porous carbons prepared by a pre-stabilization route for superior supercapacitors. Chin. Chem. Lett. doi:10.1016/j.cclet.2022.03.027
Mei, Y. Y. (2022). Activation types of biomass carbon aerogels and its application progress. Jiangxi Chem. Ind. 38 (2), 15–17.
Meng, Qinghan, Liu, Ling, Song, Huaihe, and Ling, Licheng (2004). Study on supercapacitors with carbon aerogels as electrodes. Funct. Mater. 35 (004), 457–459.
Miao, L., Duan, H., Zhu, D., Lv, Y., Gan, L., Li, L., et al. (2021). Boron “gluing” nitrogen heteroatoms in a prepolymerized ionic liquid-based carbon scaffold for durable supercapacitive activity. J. Mat. Chem. A 9, 2714–2724. doi:10.1039/d0ta09985f
Miao, L., Song, Z., Zhu, D., Li, L., Gan, L., and Liu, M. (2021). Ionic liquids for supercapacitive energy storage: A mini-review. Energy fuels. 35, 8443–8455. doi:10.1021/acs.energyfuels.1c00321
Morteza, M., Ehsan, T., and Mohammad, J. M. (2015). First-principles calculation of quantum capacitance of codoped graphenes as supercapacitor electrodes. J. Phys. Chem. C 119 (47), 26290–26295. doi:10.1021/acs.jpcc.5b07943
Mousavi-Khoshdel, S. M., Jahanbakhsh-bonab, P., and Targholi, E. (2016). Structural, electronic properties, and quantum capacitance of B, N and P-doped armchair carbon nanotubes. Phys. Lett. A 380 (41), 3378–3383. doi:10.1016/j.physleta.2016.07.067
Mousavi-Khoshdel, S. M., and Targholi, E. (2015). Exploring the effect of functionalization of graphene on the quantum capacitance by first principle study. Carbon 89, 148–160. doi:10.1016/j.carbon.2015.03.013
Nizam, N. U. M., Hanafiah, M. M., Mahmoudi, E., Halim, A. A., and Mohammad, A. W. (2021). The removal of anionic and cationic dyes from an aqueous solution using biomass-based activated carbon. Sci. Rep. 11 (1), 8623. doi:10.1038/s41598-021-88084-z
Olabi, A. G., Abdelkareem, M. A., Wilberforce, T., and Sayed, E. T. (2021). Application of graphene in energy storage device-A review. Renew. Sustain. Energy Rev. 135, 110026. doi:10.1016/j.rser.2020.110026
Paek, E., Pak, A. J., and Hwang, G. S. A. (2013). Computational study of the interfacial structure and capacitance of graphene in [BMIM] [PF6] ionic liquid. J. Electrochem. Soc. 160, A1–A10. doi:10.1149/2.019301jes
Pang, Z., Li, G., Zou, X., Sun, C., Hu, C., Tang, W., et al. (2021). An integrated strategy towards the facile synthesis of core-shell SiC-derived carbon@N-doped carbon for high-performance supercapacitors. J. Energy Chem. 56, 512–521. doi:10.1016/j.jechem.2020.08.042
Paul, R., and Roy, A. K. (2021). BN-codoped CNT based nanoporous brushes for all-solid-state flexible supercapacitors at elevated temperatures. Electrochim. Acta 365, 137345. doi:10.1016/j.electacta.2020.137345
Pekala, R. W. (1989). Organic aerogels from the polycondensation of resorcinol with formaldehyde. J. Mat. Sci. 24, 3221–3227. doi:10.1007/bf01139044
Ping, C., Li, T., Hang, Y., Lei, Z., Liu, Z., and Lei, Z. (2016). Biomass-derived carbon fiber aerogel as a binder-free electrode for high-rate supercapacitors. J. Phys. Chem. C 120 (4), 2079–2086. doi:10.1021/acs.jpcc.5b11280
Qin, C. L., Xing, L. U., Yin, G. P., Bai, X. D., and Jin, Z. (2009). Activated nitrogen-enriched carbon/carbon aerogel nanocomposites for supercapacitor applications. Trans. Nonferrous Metals Soc. China 19, s738–s742. doi:10.1016/s1003-6326(10)60142-2
Qin, Y., Miao, L., Mansuer, M., Hu, C., Lv, Y., Gan, L., et al. (2022). Spatial confinement strategy for micelle-size-mediated modulation of mesopores in hierarchical porous carbon nanosheets with an efficient capacitive response. ACS Appl. Mat. Interfaces 14, 33328–33339. doi:10.1021/acsami.2c08342
Ramirez, N., Zámbó, D., Sardella, F., Kibling, P. A., Schlosser, A., Graf, R. T., et al. (2021). Pd-doped cellulose carbon aerogels for energy storage applications. Adv. Mat. Interfaces 8, 2100310. doi:10.1002/admi.202100310
Rashidi, N. A., and Yusup, S. (2020). Recent methodological trends in nitrogen-functionalized activated carbon production towards the gravimetric capacitance: A mini review. J. Energy Storage 32, 101757. doi:10.1016/j.est.2020.101757
Rashidi, N. A., Chai, Y. H., Ismail, I. S., Othman, M. F. H., and Yusup, S. (2022). Biomass as activated carbon precursor and potential in supercapacitor applications. Biomass Convers. biorefin., 1–15. doi:10.1007/s13399-022-02351-1
Sahoo, S., Satpati, A. K., Sahoo, P. K., and Naik, P. D. (2018). Incorporation of carbon quantum dots for improvement of supercapacitor performance of nickel sulfide. ACS Omega 3, 17936–17946. doi:10.1021/acsomega.8b01238
Sakita, A., Noce, R. D., and Lavall, R. L. (2021). Potential-dependent electrochemical impedance spectroscopy as a powerful tool for evaluating supercapacitor electrode performance. J. Electrochem. Soc. 168 (8), 080525. doi:10.1149/1945-7111/ac1cfc
Salunkhe, R. R., Kaneti, Y. V., Kim, J., and Yamauchi, Y. (2016). Nanoarchitectures for metal-organic framework-derived nanoporous carbons toward supercapacitor applications. Acc. Chem. Res. 49, 2796–2806. doi:10.1021/acs.accounts.6b00460
Shao, H., Wu, Y. C., Lin, Z., Taberna, P. L., and Simon, P. (2020). Nanoporous carbon for electrochemical capacitive energy storage. Chem. Soc. Rev. 49 (10), 3005–3039. doi:10.1039/d0cs00059k
Silva, D., Paulista, N., Antenor, J., Pascon, A., Fileti, E. E., Fonseca, L. R. C., et al. (2020). Exploring doped or vacancy-modified graphene-based electrodes for applications in asymmetric supercapacitors. Phys. Chem. Chem. Phys. 22, 3906–3913. doi:10.1039/c9cp06495h
Singh, M., Gupta, A., Sundriyal, S., Jain, K., and Dhakate, S. R. (2021). Kraft lignin-derived free-standing carbon nanofibers mat for high-performance all-solid-state supercapacitor. Mater. Chem. Phys. 264, 124454. doi:10.1016/j.matchemphys.2021.124454
Song, C., Wang, J., Meng, Z., Hu, F., and Jian, X. (2018). Density functional theory calculations of the quantum capacitance of graphene oxide as a supercapacitor electrode. ChemPhysChem 19 (13), 1579–1583. doi:10.1002/cphc.201800070
Song, J. Y., Wang, S., Wang, X., and Wang, K. (2021). Characterization of the electrochemical properties of nitrogen-doped graphene. Int. J. Electrochem. Sci. 16, 150541. doi:10.20964/2021.01.35
Song, Z., Miao, L., Ruhlmann, L., Lv, Y., Zhu, D., Li, L., et al. (2022). Lewis pair interaction self-assembly of carbon superstructures harvesting high-energy and ultralong-life zinc-ion storage. Adv. Funct. Mat. 32, 2208049. doi:10.1002/adfm.202208049
Song, Z., Miao, L., Duan, H., Ruhlmann, L., Lv, Y., Zhu, D., et al. (2022). Anionic Co-insertion charge storage in dinitrobenzene cathodes for high-performance aqueous zinc-organic batteries. Angew. Chem. Int. Ed. Engl. 61, e202208821. doi:10.1002/anie.202208821
Song, Z., Miao, L., Li, L., Zhu, D., Gan, L., and Liu, M. (2021). A robust strategy of solvent choice to synthesize optimal nanostructured carbon for efficient energy storage. Carbon 180, 135–145. doi:10.1016/j.carbon.2021.04.078
Song, Z., Miao, L., Ruhlmann, L., Lv, Y., Zhu, D., Li, L., et al. (2021). Self-assembled carbon superstructures achieving ultra-stable and fast proton-coupled charge storage kinetics. Adv. Mat. 33, 202104148. doi:10.1002/adma.202104148
Stoller, M. D., Magnuson, C. W., Zhu, Y. W., Murali, S., Suk, J. W., Piner, R., et al. (2011). Interfacial capacitance of single layer graphene. Energy Environ. Sci. 4, 4685–4689. doi:10.1039/c1ee02322e
Sujatha, K. A. (2021). Study on petromodernity, the socio-cultural implications of oil and the disintegration of human behaviour in james howard kunstler's world made by hand. Rupkatha J. Interdiscip. Stud. Humanit. 13 (3), 1–13.
Sun, L., Tian, C., Li, M., Meng, X., Wang, L., Wang, R., et al. (2013). From coconut shell to porous graphene-like nanosheets for high-power supercapacitors. J. Mat. Chem. A 1, 6462–6470. doi:10.1039/c3ta10897j
Taberna, P. L., Simon, P., and Fauvarque, J. F. (2003). Electrochemical characteristics and impedance spectroscopy studies of carbon-carbon supercapacitors. J. Electrochem. Soc. 150, A292. doi:10.1149/1.1543948
Taer, E., Susanti, A., Taslim, R., and Apriwandi, (2021). Renewable and environmentally friendly of “red shoots” leaves biomass-based carbon electrode materials for supercapacitor energy storage. J. Phys. Conf. Ser. 1811, 012135. doi:10.1088/1742-6596/1811/1/012135
Tao, L., Huang, Y., Zheng, Y., Yang, X., Liu, C., Di, M., et al. (2019). Porous carbon nanofiber derived from a waste biomass as anode material in lithium-ion batteries. J. Taiwan Inst. Chem. Eng. 95, 217–226. doi:10.1016/j.jtice.2018.07.005
Tatrari, G., Karakoti, M., Tewari, C., Pandey, S., Bohra, S. B., Dandapat, A., et al. (2021). Solid waste-derived carbon nanomaterials for supercapacitor applications: A recent overview. Mat. Adv. 2, 1454–1484. doi:10.1039/d0ma00871k
Teimuri-Mofrad, R., Payami, E., Piriniya, A., and Hadi, R. (2022). Green synthesis of ferrocenyl-modified MnO2/carbon-based nanocomposite as an outstanding supercapacitor electrode material. Appl. Organomet. Chem. 36 (5), e6620. doi:10.1002/aoc.6620
Tian, X., Ma, H., Li, Z., Yan, S., Ma, L., Yu, F., et al. (2017). Flute type micropores activated carbon from cotton stalk for high performance supercapacitors. J. Power Sources 359, 88–96. doi:10.1016/j.jpowsour.2017.05.054
Tong, L., Sonnenberg, L. A., Wu, W., Boyer, S. M., Fox, M. T., Li, B., et al. (2021). Fabrication of high-performance flexible supercapacitor electrodes with poly(3, 4-ethylenedioxythiophene) (PEDOT) grown on carbon-deposited polyurethane sponge. Energies 14, 7393. doi:10.3390/en14217393
Umezawa, S., Douura, T., Yoshikawa, K., Takashima, Y., Yoneda, M., Gotoh, K., et al. (2021). Supercapacitor electrode with high charge density based on boron doped porous carbon derived from covalent organic frameworks. Carbon 184, 418–425. doi:10.1016/j.carbon.2021.08.022
Vermisoglou, E. C., Jakubec, P., Bakandritsos, A., Kupka, V., Pykal, M., Šedajová, V., et al. (2021). Graphene with covalently grafted amino acid as a route toward eco-friendly and sustainable supercapacitors. ChemSusChem 14, 3904–3914. doi:10.1002/cssc.202101039
Wang, C., Huang, J., Qi, H., Cao, L., Xu, Z., Cheng, Y., et al. (2017). Controlling pseudographtic domain dimension of dandelion derived biomass carbon for excellent sodium-ion storage. J. Power Sources 358, 85–92. doi:10.1016/j.jpowsour.2017.05.011
Wang, D., Geng, Z., Li, B., and Zhang, C. (2015). High performance electrode materials for electric double-layer capacitors based on biomass-derived activated carbons. Electrochim. Acta 173, 377–384. doi:10.1016/j.electacta.2015.05.080
Wang, J., Li, Z., Yan, S., Yu, X., Ma, Y., and Ma, L. (2019). Modifying the microstructure of algae-based active carbon and modelling supercapacitors using artificial neural networks. RSC Adv. 9, 14797–14808. doi:10.1039/c9ra01255a
Wang, L., and Pumera, M. (2016). Electrochemical catalysis at low dimensional carbons: Graphene, carbon nanotubes and beyond–a review. Appl. Mater. Today 5, 134–141. doi:10.1016/j.apmt.2016.09.011
Wang, L., Huang, M., Huang, J., Tang, X., Li, L., Peng, M., et al. (2021). Coupling of EDLC and the reversible redox reaction: Oxygen functionalized porous carbon nanosheets for zinc-ion hybrid supercapacitors. J. Mat. Chem. A 9, 15404–15414. doi:10.1039/d1ta03568a
Wang, Y., Shi, Z., Huang, Y., Ma, Y., Wang, Y., Chen, M., et al. (2009). Supercapacitor devices based on graphene materials. J. Phys. Chem. C 113 (30), 13103–13107. doi:10.1021/jp902214f
Wang, Y., Zhang, L., Hou, H., Xu, W., Duan, G., He, S., et al. (2021). Recent progress in carbon-based materials for supercapacitor electrodes: A review. J. Mat. Sci. 56, 173–200. doi:10.1007/s10853-020-05157-6
Wei, L., Sevilla, M., Fuertes, A. B., Mokaya, R., Yushin, G., Feng, J., et al. (2010). A three-dimensional carbon nanotube/graphene sandwich and its application as electrode in supercapacitors. Adv. Mat. 22, 3723–3728. doi:10.1002/adma.201001029
Welsby, D., Price, J., Pye, S., and Ekins, P. (2021). Unextractable fossil fuels in a 1.5 C world. Nature 597 (7875), 230–234. doi:10.1038/s41586-021-03821-8
Wu, S., Zhou, H., Zhou, Y., Wang, H., Li, Y., Liu, X., et al. (2021). Keratin-derived heteroatoms-doped hierarchical porous carbon materials for all-solid flexible supercapacitors. J. Alloys Compd. 859, 157814. doi:10.1016/j.jallcom.2020.157814
Wulandari, N. N., Rustamaji, H., Fibarzy, W. U., Prakoso, T., Rizkiana, J., Devianto, H., et al. (2021). Production of activated carbon from palm empty fruit bunch as supercapacitor electrode material. IOP Conf. Ser. Mat. Sci. Eng. 1143, 012004. doi:10.1088/1757-899x/1143/1/012004
Xiao, K., Ding, L. X., Liu, G., Chen, H., Wang, S., and Freestanding, H. (2016). Freestanding, hydrophilic nitrogen-doped carbon foams for highly compressible all solid-state supercapacitors. Adv. Mat. 28, 5997–6002. doi:10.1002/adma.201601125
Xin, Y., Dai, X., Lv, G., Wei, X., Liu, Y., Li, Z., et al. (2021). Stability-enhanced α-Ni(OH)2 pillared by metaborate anions for pseudocapacitors. ACS Appl. Mat. Interfaces 13 (24), 28118–28128. doi:10.1021/acsami.1c04525
Xue, D., Zhu, D., Duan, H., Wang, Z., Lv, Y., Xiong, W., et al. (2019). Deep-eutectic-solvent synthesis of N/O self-doped hollow carbon nanorods for efficient energy storage. Chem. Commun. 55, 11219–11222. doi:10.1039/c9cc06008a
Yan, J., Miao, L., Duan, H., Zhu, D., Lv, Y., Li, L., et al. (2022). High-energy aqueous supercapacitors enabled by N/O codoped carbon nanosheets and ”water-in-salt” electrolyte. Chin. Chem. Lett. 33, 2681–2686. doi:10.1016/j.cclet.2021.08.123
Yang, M., and Zhou, Z. (2017). Recent Breakthroughs in supercapacitors boosted by nitrogen-rich porous carbon materials. Adv. Sci. 4, 1600408. doi:10.1002/advs.201600408
Yang, N., Ji, L., Fu, H., Shen, Y., Wang, M., Liu, J., et al. (2022). Hierarchical porous carbon derived from coal-based carbon foam for high-performance supercapacitors. Chin. Chem. Lett. 33 (8), 3961–3967. doi:10.1016/j.cclet.2022.03.037
Yang, N., Ji, L., Fu, H., Shen, Y., Wang, M., Liu, J., et al. (2022). Hierarchical porous carbon derived from coal-based carbon foam for high-performance supercapacitors. Chin. Chem. Lett. 209, 152–157.
Yuan, Y. D., Sun, Y., Feng, Z., Li, X., Yi, R., Sun, W., et al. (2021). Nitrogen-doped hierarchical porous activated carbon derived from paddy for high-performance supercapacitors. Materials 14 (2), 318. doi:10.3390/ma14020318
Zhai, Y., Dou, Y., Zhao, D., Fulvio, P. F., Mayes, R. T., and Dai, S. (2011). Carbon materials for chemical capacitive energy storage. Adv. Mat. 23, 4828–4850. doi:10.1002/adma.201100984
Zhai, Z., Zhang, L., Du, T., Ren, B., Xu, Y., Wang, S., et al. (2022). A review of carbon materials for supercapacitors. Mat. Des. 221, 111017. doi:10.1016/j.matdes.2022.111017
Zhai, Z. Z., Ren, B., Xu, Y., Wang, S., Zhang, L., and Liu, Z. (2021). Nitrogen self-doped carbon aerogels from chitin for supercapacitors. J. Power Sources 380, 138237.
Zhang, B., Heb, J., Zheng, G., Huang, Y., Wang, C., Hea, P., et al. (2021). Electrohydrodynamic 3D printing of orderly carbon/nickel composite network as supercapacitor electrodes. J. Mat. Sci. Technol. 82, 135–143. doi:10.1016/j.jmst.2020.12.034
Zhang, S., Shi, X., Wróbel, R., Chen, X., and Mijowska, E. (2019). Low-cost nitrogen-doped activated carbon prepared by polyethylenimine (PEI) with a convenient method for supercapacitor application. Electrochim. Acta 294, 183–191. doi:10.1016/j.electacta.2018.10.111
Zhang, X., Zhang, K., Li, H., Cao, Q., Jin, L., and Li, P. (2017). Porous graphitic carbon microtubes derived from willow catkins as a substrate of MnO 2 for supercapacitors. J. Power Sources 344, 176–184. doi:10.1016/j.jpowsour.2017.01.107
Zhao, J., Lu, H., Zhang, Y., Yu, S., Malyi, O. I., Zhao, X., et al. (2021). Direct coherent multi-ink printing of fabric supercapacitors. Sci. Adv. 7 (3), eabd6978. doi:10.1126/sciadv.abd6978
Zheng, X., Miao, L., Song, Z., Du, W., Zhu, D., Lv, Y., et al. (2022). In-situ nanoarchitecturing of conjugated polyamide network-derived carbon cathodes toward high energy-power Zn-ion capacitors. J. Mat. Chem. A 10, 611–621. doi:10.1039/d1ta07350h
Zhou, H., Zhan, Y., Guo, F., Du, S., Tian, B., Dong, Y., et al. (2021). Synthesis of biomass-derived carbon aerogel/MnO composite as electrode material for high-performance supercapacitors. Electrochimica Acta 390, 390. doi:10.1016/j.electacta.2021.138817
Keywords: supercapacitors, electrode materials, surface functionalization, working principal, EDLC
Citation: Lv Y, Wang J, Ji D, Li J, Zhao S, Zhao Y, Cai Z, He X and Sun X (2023) Carbonaceous electrode materials for supercapacitor: Preparation and surface functionalization. Front. Energy Res. 10:957032. doi: 10.3389/fenrg.2022.957032
Received: 30 May 2022; Accepted: 28 November 2022;
Published: 26 January 2023.
Edited by:
Hongyuan Zhao, Henan Institute of Science and Technology, ChinaReviewed by:
Subramania Angaiah, Pondicherry University, IndiaCopyright © 2023 Lv, Wang, Ji, Li, Zhao, Zhao, Cai, He and Sun. This is an open-access article distributed under the terms of the Creative Commons Attribution License (CC BY). The use, distribution or reproduction in other forums is permitted, provided the original author(s) and the copyright owner(s) are credited and that the original publication in this journal is cited, in accordance with accepted academic practice. No use, distribution or reproduction is permitted which does not comply with these terms.
*Correspondence: Yaokang Lv, eWFva2FuZ2x2QHpqdXQuZWR1LmNu; Xuehua He, aHpoZXhoQHpqdXQuZWR1LmNu; Xiaofang Sun, emdkc3hmQHpqdXQuZWR1LmNu
Disclaimer: All claims expressed in this article are solely those of the authors and do not necessarily represent those of their affiliated organizations, or those of the publisher, the editors and the reviewers. Any product that may be evaluated in this article or claim that may be made by its manufacturer is not guaranteed or endorsed by the publisher.
Research integrity at Frontiers
Learn more about the work of our research integrity team to safeguard the quality of each article we publish.