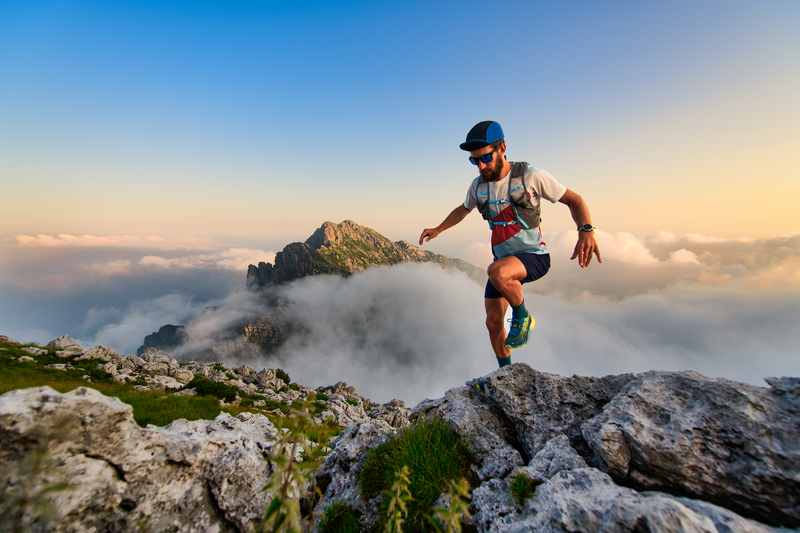
95% of researchers rate our articles as excellent or good
Learn more about the work of our research integrity team to safeguard the quality of each article we publish.
Find out more
ORIGINAL RESEARCH article
Front. Energy Res. , 19 July 2022
Sec. Solar Energy
Volume 10 - 2022 | https://doi.org/10.3389/fenrg.2022.914115
This article is part of the Research Topic Emerging Thin-Film Solar Cell Research View all 6 articles
The current–voltage curves of memristors exhibit significant hysteresis effects of use for information storage and computing. Here, we provide a comparison of different devices based on MAPbI3 perovskite with different contact configurations, from a 15% efficient solar cell to a pure memristor that lacks directional photocurrent. Current–voltage curves and impedance spectroscopy give insights into the different types of hysteresis, photocapacitance, and inductance present in halide perovskites. It is shown that both halide perovskite memristors and solar cells show a large inverted hysteresis effect at the forward bias that is related to the presence of a chemical inductor component in the equivalent circuit. Based on the results, we classify the observed response according to recombination current in devices with selective contacts, to voltage-activated single-carrier device conduction in devices with symmetric contacts. These findings serve to gain an understanding of the mechanism of memristor currents in mixed ionic-electronic conductors such as halide perovskites. We establish the link in the electrical response between solar cells and memristors.
Metal halide perovskite (MHP) is a promising photovoltaic technology that has produced very high efficiencies with solution-processed methodologies (Kim et al., 2020). MHPs can be described using an ABX3 structure, where A = monovalent cations (i.e., methyl ammonium—MA), B = divalent cations (i.e., Pb2+), and X = halide anions (i.e., I−), with MAPbI3 (MAPI) being the most studied configuration. In addition to remarkable electrooptical semiconductor properties, MHP semiconductors show mixed ionic-electronic conduction by ionic defect displacement (Azpiroz et al., 2015; Lopez-Varo et al., 2018; Senocrate and Maier, 2019; Zhang et al., 2020). This causes intrinsic memory effects (hysteresis) in the current–voltage (
While conventional memristors are activated by purely electrical stimuli, photo-stimulation of memristive responses provides new opportunities for a variety of applications such as light-gated and electro-photo-sensitive memristors for opto-neuromorphic and arithmetic computing, (Maier et al., 2016; Tan et al., 2017; John et al., 2018b; Emboras et al., 2020; John et al., 2020; Ma et al., 2021) integrated photonic neural networks, (Stark et al., 2020; Shastri et al., 2021), and preprocessing of image data in artificial vision before the transfer to the computing unit (Yang et al., 2020; Gong et al., 2021; Kim et al., 2021). Direct processing of visual information with such optoelectronic memristors may enable simpler architectures, mitigating the need for additional electro-optical converters for signal transduction and communication. MHPs are attractive materials for developing such novel electrooptical neuromorphic platforms because of their excellent optical absorption coefficient, charge transport, bandgap tunability extending across the UV, visible, and IR spectra, and high photoluminescence (PL) quantum yield, and high extinction coefficients. Combining these outstanding electronic properties with their intrinsic ionic conduction, MHPs would allow easy implementation of electrooptical synaptic elements for information storage and computing.
MHP memristors emerge from solar cells, but their behavior in the current-voltage regime is very different. In solar cells, the inverted hysteresis is a property preferably minimized (Tress et al., 2016; Yang et al., 2017; Wu et al., 2018), while for memristors, it is amplified to permanent and reversible changes of the conductivity (Berruet et al., 2022). These characteristics have not been systematically studied yet, entailing significant attention.
Here, we aim to provide a clear understanding on the multiple features of the very complex
Since the hysteresis of the current–voltage (Almora et al., 2016; Tress et al., 2016; Rong et al., 2017; Wu et al., 2018; Alvarez et al., 2020; Bisquert et al., 2021; Bisquert and Guerrero, 2022a) is a central characteristic of the memristive devices, we summarize in Figure 2 on the basic idea of capacitive and inductive hysteresis as described recently (Bisquert et al., 2021; Bisquert and Guerrero, 2022a). The type of hysteresis and IS response is highly connected with the presence of capacitive and inductive currents. The hysteresis is denoted “regular” as observed in solar cells when the current of the photovoltaic quadrant is more positive in the forward scan, Figure 1B. Alternatively, hysteresis is “inverted” as observed often in memristors when the positive current of the forward scan is lower than the positive current of the backward scan, Figure 1D (Li et al., 2022; Shen et al., 2017). Regular hysteresis has previously been correlated with the presence of capacitive behavior in the IS response while the inverted hysteresis indicates the presence of chemical inductors in the response. The chemical inductor (Bisquert and Guerrero, 2022a) is a general denomination for a class of dynamical phenomena often based on a chemical reaction that produces a formal inductive response in impedance and transients without an underlying electromagnetic effect. The chemical inductor observed in halide perovskite solar cells is related to the slow ion-controlled electronic phenomena that occur at the interfaces of the devices. More details will be given in the following experiments.
FIGURE 1. Schematic response of impedance spectroscopy, current at constant sweep rate
We note that the hysteresis effects have been already observed in emerging solar cells, where ion motion is significant (Contreras et al., 2016; Elbohy et al., 2019). For example, the dye-sensitized solar cell contains a liquid electrolyte, and the strong capacitive hysteresis in
FIGURE 2. Normalized (reference voltage: −0.1 V) current–voltage curves of DSCs measured in the reverse scan (line) and forward scan (dash line) under a light intensity of 10 mW cm−2 using a green LED. The influence of hysteresis-determining factors is specifically shown: (A) scan rate, (B) temperature, and (C) electrolyte solvent. Reproduced from (Contreras et al., 2016).
FIGURE 3. The forward and reverse current–voltage characteristics for DSCs fabricated with an electrolyte containing (A) NaI at 1V/s scan rate, (B) KI at 1V/s scan rate, (C) CsI at 1V/s scan rate, (D) NaI at 0.5V/s scan rate, (E) KI at 0.5V/s scan rate, (F) CsI at 0.5V/s scan rate. Reproduced with permission from (Elbohy et al., 2019).
It has been reported that the resistive switching mechanism can be modified by using different top contacts (Han et al., 2019). Here, we focus on a low reactivity Au contact which is widely used in solar cells and the switching is controlled by the interface. In halide perovskite solar cells, migrating ions that reach the external contacts generate a capacitive current as the contacts compensate for their charge providing electroneutrality in the form of a capacitor (Figure 4). In MHP, this capacitance also depends on the light intensity with a rising light-induced capacitance, as previously reported (Juarez-Perez et al., 2014; Guerrero et al., 2021). It is known that halides vacancies (VI+), cations A (i.e., methyl ammonium), and metal collectors all can migrate at different rates under an external voltage bias. Halides vacancies are the fastest migrating species in MHP. When we apply a positive voltage at the FTO electrode (Figure 4A), halide vacancies will go toward gold and effectively create I− excess concentration at the FTO/TiO2 interface. The opposite is true if we apply a positive voltage at the gold contact, Figure 4B. The capacitive and inductive effects of these ions at the interfaces can be monitored by IS (Guerrero et al., 2021; Berruet et al., 2022; Taukeer Khan et al., 2022) as discussed in the latter sections.
FIGURE 4. Diagrams that show the TiO2 and gold electrode polarization and direction of ion migration under a negative (A) and positive (B) bias in the dark, respectively, in MHP.
The connection between light stimulation and production of photovoltage and photocurrent is well understood for a typical solar cell, Figure 5A. (Bisquert et al., 2004; Bisquert, 2020) Photogeneration in the semiconductor produces separated electron and hole carriers that diffuse to the metal collectors and the selectivity of contacts causes a directional photocurrent. When the voltage is increased in the forward direction, recombination is enhanced, and the photocurrent decreases until it vanishes at the open-circuit voltage (
FIGURE 5. Schematic of photogeneration of an electron-hole pair under illumination for (A) FTO/c-TiO2/m-TiO2/MAPI/spiro-OMeTAD/Au solar cell (B) optoelectronic FTO/PEDOT:PSS/MAPI/Au memristor device, (C) optoelectronic FTO/c-TiO2/mp-TiO2/MAPI/Au memristor device.
Figure 6 shows the device configuration of the SC device and the electro-optical response. Our PV performance of ∼15% is well aligned with reported values for the chosen formulation (Figure 6A). Hysteresis is typically observed in the current density-voltage (
FIGURE 6. (A) Current–voltage characteristic of the FTO/c-TiO2/m-TiO2/MAPI/spiro-OMeTAD/Au solar cell was measured at 100 mW/cm2 using a halogen-based solar simulator calibrated with a silicon photodiode. The inset table shows the characteristic parameters. Here, the photocurrent is drawn positive. (B) Current−voltage characteristics were measured under a dark condition for 5 different scan rates. Arrows indicate the sweep direction.
Figure 7 shows photovoltaic performance of the solar cell device under a monochromatic blue light at different illumination intensities, with negative current for photovoltaic effect. As expected, the photogenerated current is enhanced with light intensity thanks to the excellent carrier generation and extraction. Very interestingly, in the complete SC there is a crossing in the forward and reverse scans at
FIGURE 7. (A) Current density–voltage characteristic of an FTO/c-TiO2/m-TiO2/MAPI/spiro-OMeTAD/Au solar cell measured from 0 to 100 mW/cm2 using a blue light source (470 nm) and 0.1 cm2 active area. A positive current flow from FTO to the Au electrode. (B) A magnified view of the scale. Lines represent the forward scan, while dots represent the reverse scan. (C) Low-frequency capacitance enhancement with illumination intensity for an FTO/c-TiO2/m-TiO2/MAPI/spiro-OMeTAD/Au solar cell.
Figure 8 shows the
FIGURE 8. (A) Current density−voltage characteristic measured at 50 mW/cm2 blue light illumination for an FTO/c-TiO2/m-TiO2/MAPI/spiro-OMeTAD/Au solar cell. Arrows indicate sweep direction. (B–C) Corresponding complex plane plot representation of the impedance spectra at various dc voltages, measured from 1MHz to 0.1 Hz. Inset corresponds to higher dc voltages applied.
The model systems which do not contain spiro-OMeTAD have been fully characterized with two types of selective contacts, PEDOT:PSS (selective to holes), and TiO2 (selective to electrons). The dark
FIGURE 9. Current−voltage characteristics of the (A) FTO/PEDOT:PSS/MAPI/Au and (B) FTO/c-TiO2/m-TiO2/MAPI/Au memristor measured under dark conditions for 5 different scan rates.
It is also important to highlight that the response in the dark is only inductive at positive voltages, there is no crossing for both model systems as compared to the SC for measurements in the dark. This result clearly points to the capacitive currents due to the migrating I− ions with Spiro-OMeTAD in the dark of the SC configuration. Otherwise, only PEDOT memristors show inverted hysteresis when the voltage is negative. For TiO2 memristors, the capacitive hysteresis is found in this region, as shown in solar cell devices in Figure 7B. This difference in PEDOT memristor performance is due to irreversible reactions taking place between ions and TiO2 at this interface. For example, for the interface TiO2/MAPI it has been reported that Ti−I−Pb bonds may form, as identified by Raman Spectroscopy, which can easily accommodate excess ionic charge when a negative bias is applied (Carrillo et al., 2016). This configuration could lead to neutral interfaces that would be in agreement with the low capacitance measured in the current work for the memristor containing TiO2. Comparing these characterizations with the one previously discussed in Figure 6B, it is possible to affirm that the TiO2 device shows closer performance to an MHP solar cell than the PEDOT device in dark conditions.
To investigate the effect of light on the memristor devices, we performed the current-voltage characterization at different illumination levels and the results are shown in Figure 10. In principle, the looping curves are like those in the dark in Figure 9. But there are also significant differences. The PEDOT device shows a symmetric opening of the curves
FIGURE 10. (A) Current density–voltage characteristic of an FTO/PEDOT:PSS/MAPI/Au memristor measured from 0 to 100 mW/cm2 using a blue light source (470 nm) and 0.1 cm2 active area. (B) A magnified view of the scale. (C) Low-frequency capacitance enhancement with illumination intensity for an FTO/PEDOT:PSS/MAPI/Au memristor. (D) The current density–voltage characteristic of an FTO/c-TiO2/m-TiO2/MAPI/Au memristor was measured at different illumination intensities. Panel. (E) corresponds to a magnified view of the scale. Lines represent the forward scan and dots represent the reverse scan. (F) Corresponding low-frequency capacitance variation with illumination intensity.
For further analysis, we focus on a particular illumination, Figure 11. For PEDOT devices, it is observed that there are two loops on right and left of Figure 11A, where the current in forward and backward makes crossing pathways. As mentioned earlier in Figure 3 this property indicates a change from regular to inverted hysteresis caused by the onset of dominant inductive property in the impedance spectra (Bisquert and Guerrero, 2022a; Berruet et al., 2022). The presence of the chemical inductor (the loop in the fourth quadrant of the complex plane) is in fact obtained in the measurements of impedance spectroscopy in Figures 11B,C. While this change from capacitive to inductive behavior is shown in Figure 11E at high positive voltages, this is not obtained when negative voltages are studied in TiO2 memristors (Figure 9B), corresponding with the loops in Figure 8D. This trend is similar to the one shown by the solar cell device, Figures 8B,C, with inductive properties significant only at high dc voltages.
FIGURE 11. (A) Current density−voltage characteristic measured at 50 mW/cm2 blue light illumination for an FTO/PEDOT:PSS/MAPI/Au memristor. Arrows indicate sweep direction. (B–C) Corresponding complex plane plot representation of the impedance spectra at various dc voltages, measured from 1MHz to 0.1 Hz. Inset corresponds to higher dc voltages applied. (D) Current−voltage characteristic measured at 50 mW/cm2 blue light illumination for an FTO/c-TiO2/m-TiO2/MAPI/Au memristor. (E–F) Corresponding complex plane plot representation of the impedance spectra at various dc voltages.
In order to explain the experimental observations in a unified framework, we present a dynamical model (Berruet et al., 2022) that includes several capacitive mechanisms and a chemical inductor feature. It is formed by the system of equations
The model has three independent variables:
Eq. 1 describes the components of
If the time derivative in Eq. 2 is suppressed, we obtain the following steady-state solution:
Eq. 2 is a delay equation that states that the formation of the conduction state at a certain voltage,
The steady-state current is
In the solar cell a saturation regime
where
The equivalent circuit is shown in Figure 12.
FIGURE 12. Equivalent circuit model. The impedance model is obtained by a small perturbation of Eqs. (1, 2). It has the expression (Berruet et al., 2022).
Based on this model we can form several regimes of responses observed in the experimental evidence of the preceding Section.
In Figure 13 we show the effect of a constant capacitor
FIGURE 13. Calculation of a memristor/solar cell model. (A) Current at forward and backward scan at different rates as indicated. The grey line is the total equilibrium dc current (B) Impedance spectrum at
FIGURE 14. Calculation of a memristor/solar cell model. (A) Current at forward and backward scan at different rates as indicated. The grey line is the total equilibrium dc current (B) Impedance spectrum
Figure 15A shows the inductive hysteresis loop that is observed at a large injection current in the measuring devices. To provide a more complete description of the inductive hysteresis we extend the model as follows:
FIGURE 15. Calculation of a memristor/solar cell model. (A) Current at forward and backward scan at different rates as indicated. The grey line is the total equilibrium dc current (B) Impedance spectrum at
This more general three-dimensional model has four independent variables:
As described before (Berruet et al., 2022), Eq. 12 represents a diffusion or migration time of ions that introduces a delay
Finally, in Figure 16 the capacitive hysteresis is combined with an inductive hysteresis at high voltage. There is a crossing point from capacitive to inductive hysteresis in Figure 16, as observed in Figure 7A. In the impedance spectra, the low-frequency capacitive arc is transformed into an inductive feature in the fourth quadrant of the complex plane.
FIGURE 16. Calculation of a memristor/solar cell model. (A) Current at forward and backward scan at different rates as indicated. The grey line is the total equilibrium dc current (B) Impedance spectrum at
The previous analysis shows that a voltage-activated current with capacitive and inductive kinetic properties provides a satisfactory explanation of the current-voltage hysteresis and impedance properties of the different devices studied. We obtained a good description of the experimental results. Models with very few variables describe a large span of observed phenomena. The question is to give a definitive interpretation to the transformation equations in terms of physical mechanisms. One recent example is the combination of bidirectional photocurrent and hysteresis that has been discussed by modification of the contact layer. (Li et al. 2022).
We considered three types of devices: a solar cell with excellent selective contacts, a PEDOT memristor with both contacts selective to holes, and a hybrid TiO2 device with partially selective contacts. In Figure 5 we showed elementary models to explain the mechanism under light conditions close to zero applied voltage that leads to the generation of a photocurrent. In the experimental figures, we have observed the transition to a large current when the Au electrode is positively biased. This large current and the associated memory effect is the key operational characteristic of a memristor. In addition, we showed that the presence of regular/capacitive hysteresis under illumination is connected with the surface ionic polarization by the high capacitance measured by IS and the large capacitive current that scales with the light intensity.
Alternatively, for measurements in the dark, large inductive currents have been observed in all types of devices measured. We can discuss the origin of the large current rise in the dark injection regime based on Figure 17.
FIGURE 17. Scheme of electrical current under strong injection regime. (A) In perfect selective contacts the current is completely due to recombination. (B) For similar contacts the device becomes a single carrier in which the current goes through from one contact to the other.
In the case of a solar cell with very good selective contacts, the large current is due to recombination as shown in Figure 17A. This current regime is usually not analyzed in solar cells as the regime beyond
For the operation of memristors, the injection domain is an important feature of the device operation. It is therefore essential to obtain a physical description of the variable
1) The memristor device injects electrons and holes by partially selective contacts as in Figure 17A, producing a recombination current and
But another possibility is that there is no contact selectivity and the current is due to just one carrier going through the sample as in Figure 17B, i.e., electron-only devices. (Li et al., 2022) In the current work both PEDOT:PSS and Au are contacted more selective to holes than electrons due to their energy levels and have often been used for this purpose in solar cells and LEDs research. Therefore, it is likely that if the second possibility is responsible for the inductive currents and the device would be hole-only. The question is to determine the physical origin of the activation function
2) The activation of surface conduction sites by reaction of the incoming ions, Figure 18A. Here is the interpretation of the function
3) The decrease of an electronic surface barrier between the perovskite layer and the contacts, Figure 18B (Yang et al., 2013). This is caused by changes in doping ions around the interface, in a Schottky barrier diode mechanism.
4) A filamentary conductive pathway through the perovskite layer, is the standard mechanism of high current in memristors (Fang et al., 2021).
FIGURE 18. Schematics of effects of incoming ions. (A) Formation of conductive sites by the local reaction. (B) Reduction of an electrostatic barrier by increased doping.
The present experimental results and modeling do not distinguish between these options, or between models in Figure 17 and Figure 18, and additional discriminatory experimental work is needed.
The operation of solar cells and memristors depends crucially on the structure of contacts. We found different phenomena like directional photocurrent, photocapacitance at low bias voltage, and alternation of capacitive and inductive hysteresis domains can be explained by a model in which the rising current is activated by voltage. In the case of a solar cell, this current is well explained by the ionic-dependent recombination model. But for the memristor, different options appear since the devices are less affected by photogeneration and may become single carrier dominated. We conclude that solar cells and memristors can have very different underlying mechanisms, but more investigation is needed for the attribution of the rising current and the memory effect.
All materials and solvents were used as received. FTO glass (Pilkington TEC 15), PEDOT:PSS (Heraeus CLEVIOS™ P VP AI 4083), titanium diisopropoxide bis(acetylacetonate) (Merk, 75% solution in 2-propanol), absolute ethanol (Sigma Aldrich, anhydrous 99.8%), acetylacetone (Sigma Aldrich, 99%), TiO2 paste (Dyesol, DSL 18NR-T),CH3NH3I (MAI, Greatcellsolar), PbI2 (TCI, 99.99%), DMF (Sigma Aldrich, anhydrous 99.8%), DMSO (Sigma Aldrich, anhydrous 99.9%), chlorobenzene (Sigma Aldrich, 99.8%), spiro-OMeTAD (Sigma Aldrich, anhydrous 99%), LITBSF (Sigma Aldrich, anhydrous 99.95%), Acetonitrile (Sigma Aldrich, anhydrous 99.9%), 4-tert-butylpyridine (TBP) (Sigma Aldrich, 99.8%). The precursor solution for the compact layer of TiO2 (c-TiO2) was prepared by mixing 0.4 ml of acetylacetone and 0.6 ml of titanium diisopropoxide bis(acetylacetonate) in 9 ml of absolute ethanol. In order to prepare mesoporous TiO2 layer precursor, 150 mg of TiO2 paste is required for each milliliter of ethanol. The MAPbI3 precursor solution is prepared by preparation of DMF solutions (50 wt%) containing MAI and PbI2 (1:1 mol%) and MAI, PbI2, and DMSO (1:1:1 mol%) as reported previously. Briefly, MAI (235 mg) and PbI2 (681.5 mg) were mixed in DMF (1 ml) and DMSO (95 μL). Spiro-OMeTAD solution requires 72.3 mg of spiro-OMeTAD for each milliliter of chlorobenzene. Then, 17.5 µL of LITBSF solution (520 mg of Li* salt per milliliter of acetonitrile) and 28.8 µL of TBP must be added.
Devices were prepared following previously reported methods. (Aranda et al., 2017) All devices were prepared starting from FTO glass substrates. Etching was carried out with zinc powder and HCl solution (2 M). Afterward, samples were brushed, cleaned with Hellmanex solution, and rinsed with Milli-Q water. For a complete cleaning, the substrates were sonicated in acetone for 15 min and this step was repeated with a mixed 50:50 ethanol-isopropanol solution. Finally, substrates were dried with nitrogen and treated in a UV−O3 chamber for 15 min. For PEDOT-based memristors, PEDOT:PSS solution was filtered using PTFE 0.45 μm syringe filter and spin-coated on the substrate at 3000 rpm for 30 s. This was followed by annealing at 120°C for 10 min. Alternatively, for TiO2-based devices, a compact TiO2 layer was deposited at 450°C by aerosol spray pyrolysis. The solution was sprayed using pure oxygen as a carrying gas and annealing was carried out at 450°C for 30 min. The mesoporous TiO2 layer was spin-coated at 2000 rpm for 10 s and annealed following several temperature steps. Devices were transferred to a nitrogen-filled glovebox, samples are heated at 100°C for 5 min to avoid residual humidity on their surface. The perovskite precursor solution was spin-coated at 4000 rpm for 50 s, using chlorobenzene as an antisolvent. Afterward, the substrate was annealed at 100°C for 10 min. For solar cell devices, the doped spiro-OMeTAD solution was spin-coated at 4000 rpm for 30 s. At last, Au electrodes were thermally evaporated to define an active area of 0.25 cm2 for measurements in the dark.
Dark
For IS measurements Autolab was configured to apply sinusoidal signals with a 10 mV amplitude from 1 MHz to 0.1 Hz under several illumination intensities and dc voltages, ranging from low to high illuminations and from 0 V to upper voltages. Chronoamperometry measurements during 20 s were carried out before and after IS.
The raw data supporting the conclusions of this article will be made available by the authors, without undue reservation.
LD, AR, RS, and AG made the measurements, AB and RJ were involved in conceptualization, BR, MK, AG, and JB planned the work, and AG and JB wrote the manuscript.
The authors declare that the research was conducted in the absence of any commercial or financial relationships that could be construed as a potential conflict of interest.
The handling editor MF declared a shared affiliation with the author(s) RE and MK at the time of review.
All claims expressed in this article are solely those of the authors and do not necessarily represent those of their affiliated organizations, or those of the publisher, the editors, and the reviewers. Any product that may be evaluated in this article, or claim that may be made by its manufacturer, is not guaranteed or endorsed by the publisher.
The authors thank Generalitat Valenciana for the project PROMETEO/2020/028. A.B. acknowledges FPI studentship funding from Ministerio de Ciencia e Innovación of Spain (BES-2017–080351). R.A.J. acknowledges the support from the ETH Zurich Postdoctoral Fellowship scheme.
Almora, O., Aranda, C., Zarazua, I., Guerrero, A., and Garcia-Belmonte, G. (2016). Noncapacitive Hysteresis in Perovskite Solar Cells at Room Temperature. ACS Energy Lett. 1 (1), 209–215. doi:10.1021/acsenergylett.6b00116
Alvarez, A. O., Arcas, R., Aranda, C. A., Bethencourt, L., Mas-Marzá, E., Saliba, M., et al. (2020). Negative Capacitance and Inverted Hysteresis: Matching Features in Perovskite Solar Cells. J. Phys. Chem. Lett. 11 (19), 8417–8423. doi:10.1021/acs.jpclett.0c02331
Aranda, C., Cristobal, C., Shooshtari, L., Li, C., Huettner, S., and Guerrero, A. (2017). Formation Criteria of High Efficiency Perovskite Solar Cells under Ambient Conditions. Sustain. Energy Fuels 1, 540–547. doi:10.1039/c6se00077k
Azpiroz, J. M., Mosconi, E., Bisquert, J., and De Angelis, F. (2015). Defect Migration in Methylammonium Lead Iodide and its Role in Perovskite Solar Cell Operation. Energy Environ. Sci. 8, 2118–2127. doi:10.1039/c5ee01265a
Berruet, M., Pérez-Martínez, J. C., Romero, B., Gonzales, C., Al-Mayouf, A. M., Guerrero, A., et al. (2022). Physical Model for the Current-Voltage Hysteresis and Impedance of Halide Perovskite Memristors. ACS Energy Lett. 7, 1214–1222. doi:10.1021/acsenergylett.2c00121
Bisquert, J., Cahen, D., Hodes, G., Rühle, S., and Zaban, A. (2004). Physical Chemical Principles of Photovoltaic Conversion with Nanoparticulate, Mesoporous Dye-Sensitized Solar Cells. J. Phys. Chem. B 108, 8106–8118. doi:10.1021/jp0359283
Bisquert, J., and Guerrero, A. (2022). Chemical Inductor. J. Am. Chem. Soc. 144, 5996–6009. doi:10.1021/jacs.2c00777
Bisquert, J., and Guerrero, A. (2022). Dynamic Instability and Time Domain Response of a Model Halide Perovskite Memristor for Artificial Neurons. J. Phys. Chem. Lett. 13, 3789–3795. doi:10.1021/acs.jpclett.2c00790
Bisquert, J., Guerrero, A., and Gonzales, C. (2021). Theory of Hysteresis in Halide Perovskites by Integration of the Equivalent Circuit. ACS Phys. Chem. Au 1, 25–44. doi:10.1021/acsphyschemau.1c00009
Bou, A., and Bisquert, J. (2021). Impedance Spectroscopy Dynamics of Biological Neural Elements: From Memristors to Neurons and Synapses. J. Phys. Chem. B 125, 9934–9949. doi:10.1021/acs.jpcb.1c03905
Bowring, A. R., Bertoluzzi, L., O'Regan, B. C., and McGehee, M. D. (2018). Reverse Bias Behavior of Halide Perovskite Solar Cells. Adv. Energy Mat. 8 (8), 1702365. doi:10.1002/aenm.201702365
Caprioglio, P., Stolterfoht, M., Wolff, C. M., Unold, T., Rech, B., Albrecht, S., et al. (2019). On the Relation between the Open‐Circuit Voltage and Quasi‐Fermi Level Splitting in Efficient Perovskite Solar Cells. Adv. Energy Mat. 9 (33), 1901631. doi:10.1002/aenm.201901631
Carrillo, J., Guerrero, A., Rahimnejad, S., Almora, O., Zarazua, I., Mas-Marza, E., et al. (2016). Ionic Reactivity at Contacts and Aging of Methylammonium Lead Triiodide Perovskite Solar Cells. Adv. Energy Mat. 6 (9), 1502246. doi:10.1002/aenm.201502246
Christensen, D. V., Dittmann, R., Linares-Barranco, B., Sebastian, A., and Le Gallo, M. (2022). 2022 Roadmap on Neuromorphic Computing and Engineering. Neuromorphic Comput. Eng. 2, 022501. doi:10.1088/2634-4386/ac4a83
Contreras, L., Idígoras, J., Todinova, A., Salado, M., Kazim, S., Ahmad, S., et al. (2016). Specific Cation Interactions as the Cause of Slow Dynamics and Hysteresis in Dye and Perovskite Solar Cells: A Small-Perturbation Study. Phys. Chem. Chem. Phys. 18 (45), 31033–31042. doi:10.1039/c6cp05851e
Elbohy, H., El-Mahalawy, H., El-Ghamaz, N. A., and Zidan, H. (2019). Hysteresis Analysis in Dye-Sensitized Solar Cell Based on Different Metal Alkali Cations in the Electrolyte. Electrochimica Acta 319, 110–117. doi:10.1016/j.electacta.2019.06.160
Emboras, A., Alabastri, A., Lehmann, P., Portner, K., Weilenmann, C., Ma, P., et al. (2020). Opto-Electronic Memristors: Prospects and Challenges in Neuromorphic Computing. Appl. Phys. Lett. 117 (23), 230502. doi:10.1063/5.0028539
Fang, Y., Zhai, S., Chu, L., and Zhong, J. (2021). Advances in Halide Perovskite Memristor from Lead-Based to Lead-Free Materials. ACS Appl. Mat. Interfaces 13 (15), 17141–17157. doi:10.1021/acsami.1c03433
Gogoi, H. J., Bajpai, K., Mallajosyula, A. T., and Solanki, A. (2021). Advances in Flexible Memristors with Hybrid Perovskites. J. Phys. Chem. Lett. 12 (36), 8798–8825. doi:10.1021/acs.jpclett.1c02105
Gong, J., Wei, H., Ni, Y., Zhang, S., Du, Y., and Xu, W. (2021). Methylammonium Halide-Doped Perovskite Artificial Synapse for Light-Assisted Environmental Perception and Learning. Mater. Today Phys. 21, 100540. doi:10.1016/j.mtphys.2021.100540
Guerrero, A., Bisquert, J., and Garcia-Belmonte, G. (2021). Impedance Spectroscopy of Metal Halide Perovskite Solar Cells from the Perspective of Equivalent Circuits. Chem. Rev. 121, 14430–14484. doi:10.1021/acs.chemrev.1c00214
Han, J. S., Le, Q. V., Choi, J., Kim, H., Kim, S. G., Hong, K., et al. (2019). Lead-Free All-Inorganic Cesium Tin Iodide Perovskite for Filamentary and Interface-Type Resistive Switching toward Environment-Friendly and Temperature-Tolerant Nonvolatile Memories. ACS Appl. Mat. Interfaces 11 (8), 8155–8163. doi:10.1021/acsami.8b15769
John, R. A., Acharya, J., Zhu, C., Surendran, A., Bose, S. K., Chaturvedi, A., et al. (2020). Optogenetics Inspired Transition Metal Dichalcogenide Neuristors for In-Memory Deep Recurrent Neural Networks. Nat. Commun. 11 (1), 3211. doi:10.1038/s41467-020-16985-0
John, R. A., Liu, F., Chien, N. A., Kulkarni, M. R., Zhu, C., Fu, Q., et al. (2018). Synergistic Gating of Electro‐Iono‐Photoactive 2D Chalcogenide Neuristors: Coexistence of Hebbian and Homeostatic Synaptic Metaplasticity. Adv. Mat. 30 (25), 1800220. doi:10.1002/adma.201800220
John, R. A., Shah, N., Vishwanath, S. K., Ng, S. E., Febriansyah, B., Jagadeeswararao, M., et al. (2021). Halide Perovskite Memristors as Flexible and Reconfigurable Physical Unclonable Functions. Nat. Commun. 12 (1), 3681. doi:10.1038/s41467-021-24057-0
John, R. A., Yantara, N., Ng, S. E., Patdillah, M. I. B., Kulkarni, M. R., Jamaludin, N. F., et al. (2021). Diffusive and Drift Halide Perovskite Memristive Barristors as Nociceptive and Synaptic Emulators for Neuromorphic Computing. Adv. Mat. 33 (15), 2007851. doi:10.1002/adma.202007851
John, R. A., Yantara, N., Ng, Y. F., Narasimman, G., Mosconi, E., Meggiolaro, D., et al. (2018). Ionotronic Halide Perovskite Drift-Diffusive Synapses for Low-Power Neuromorphic Computation. Adv. Mat. 30 (51), 1805454. doi:10.1002/adma.201805454
Juarez-Perez, E. J., Sanchez, R. S., Badia, L., Garcia-Belmonte, G., Kang, Y. S., Mora-Sero, I., et al. (2014). Photoinduced Giant Dielectric Constant in Lead Halide Perovskite Solar Cells. J. Phys. Chem. Lett. 5, 2390–2394. doi:10.1021/jz5011169
Kang, K., Hu, W., and Tang, X. (2021). Halide Perovskites for Resistive Switching Memory. J. Phys. Chem. Lett. 12 (48), 11673–11682. doi:10.1021/acs.jpclett.1c03408
Kim, J. Y., Lee, J.-W., Jung, H. S., Shin, H., and Park, N.-G. (2020). High-Efficiency Perovskite Solar Cells. Chem. Rev. 120 (15), 7867–7918. doi:10.1021/acs.chemrev.0c00107
Kim, M. S., Kim, M. S., Lee, G. J., Sunwoo, S.-H., Chang, S., Song, Y. M., et al. (2021). Bio-Inspired Artificial Vision and Neuromorphic Image Processing Devices. Adv. Mater. Technol. 7 (2), 2100144. doi:10.1002/admt.202100144
Kwak, K. J., Lee, D. E., Kim, S. J., and Jang, H. W. (2021). Halide Perovskites for Memristive Data Storage and Artificial Synapses. J. Phys. Chem. Lett. 12 (37), 8999–9010. doi:10.1021/acs.jpclett.1c02332
Li, D., Wang, Y., Niu, T., Chao, L., and Chen, Y. (2022). Device Physics of a Metal Halide Perovskite Diode: Decoupling of the Bulk from the Interface. J. Phys. Chem. C 126 (15), 6892–6903. doi:10.1021/acs.jpcc.2c01568
Lopez-Varo, P., Jiménez-Tejada, J. A., García-Rosell, M., Ravishankar, S., Garcia-Belmonte, G., Bisquert, J., et al. (2018). Device Physics of Hybrid Perovskite Solar Cells: Theory and Experiment. Adv. Energy Mat. 8, 1702772. doi:10.1002/aenm.201702772
Ma, C., Chen, H., Yengel, E., Faber, H., Khan, J. I., Tang, M.-C., et al. (2021). Printed Memtransistor Utilizing a Hybrid Perovskite/Organic Heterojunction Channel. ACS Appl. Mat. Interfaces 13 (43), 51592–51601. doi:10.1021/acsami.1c08583
Maier, P., Hartmann, F., Emmerling, M., Schneider, C., Kamp, M., Höfling, S., et al. (2016). Electro-Photo-Sensitive Memristor for Neuromorphic and Arithmetic Computing. Phys. Rev. Appl. 5 (5), 054011. doi:10.1103/physrevapplied.5.054011
Mehonic, A., and Kenyon, A. J. (2016). Emulating the Electrical Activity of the Neuron Using a Silicon Oxide RRAM Cell. Front. Neurosci. 10, 57. doi:10.3389/fnins.2016.00057
Li, D., Wang, Y., Niu, T., Chao, L., and Chen, Y. (2022). Device Physics of a Metal Halide Perovskite Diode: Decoupling of the Bulk From the Interface. J. Phys. Chem. 126, 6892–6903.
Pershin, Y. V., and Di Ventra, M. (2011). Memory Effects in Complex Materials and Nanoscale Systems. Adv. Phys. 60 (2), 145–227. doi:10.1080/00018732.2010.544961
Pockett, A., and Carnie, M. J. (2017). Ionic Influences on Recombination in Perovskite Solar Cells. ACS Energy Lett. 2 (7), 1683–1689. doi:10.1021/acsenergylett.7b00490
Pospisil, J., Guerrero, A., Zmeskal, O., Weiter, M., Gallardo, J. J., Navas, J., et al. (2019). Reversible Formation of Gold Halides in Single-Crystal Hybrid-Perovskite/Au Interface upon Biasing and Effect on Electronic Carrier Injection. Adv. Funct. Mat. 29 (32), 1900881. doi:10.1002/adfm.201900881
Rahimi Azghadi, M., Chen, Y.-C., Eshraghian, J. K., Chen, J., Lin, C.-Y., Amirsoleimani, A., et al. (2020). Complementary Metal‐Oxide Semiconductor and Memristive Hardware for Neuromorphic Computing. Adv. Intell. Syst. 2 (5), 1900189. doi:10.1002/aisy.201900189
Rong, Y., Hu, Y., Ravishankar, S., Liu, H., Hou, X., Sheng, Y., et al. (2017). Tunable Hysteresis Effect for Perovskite Solar Cells. Energy Environ. Sci. 10 (11), 2383–2391. doi:10.1039/c7ee02048a
Senocrate, A., and Maier, J. (2019). Solid-State Ionics of Hybrid Halide Perovskites. J. Am. Chem. Soc. 141 (21), 8382–8396. doi:10.1021/jacs.8b13594
Shastri, B. J., Tait, A. N., Ferreira de Lima, T., Pernice, W. H. P., Bhaskaran, H., Wright, C. D., et al. (2021). Photonics for Artificial Intelligence and Neuromorphic Computing. Nat. Photonics 15 (2), 102–114. doi:10.1038/s41566-020-00754-y
Shen, H., Jacobs, D. A., Wu, Y., Duong, T., Peng, J., Wen, X., et al. (2017). Inverted Hysteresis in CH3NH3PbI3 Solar Cells: Role of Stoichiometry and Band Alignment. J. Phys. Chem. Lett. 8 (12), 2672–2680. doi:10.1021/acs.jpclett.7b00571
Solanki, A., Guerrero, A., Zhang, Q., Bisquert, J., and Sum, T. C. (2020). Interfacial Mechanism for Efficient Resistive Switching in Ruddlesden-Popper Perovskites for Non-Volatile Memories. J. Phys. Chem. Lett. 11 (2), 463–470. doi:10.1021/acs.jpclett.9b03181
Stark, P., Horst, F., Dangel, R., Weiss, J., and Offrein, B. J. (2020). Opportunities for Integrated Photonic Neural Networks. J. Nanophot. 9 (13), 4221–4232. doi:10.1515/nanoph-2020-0297
Tan, H., Liu, G., Yang, H., Yi, X., Pan, L., Shang, J., et al. (2017). Light-Gated Memristor with Integrated Logic and Memory Functions. ACS Nano 11 (11), 11298–11305. doi:10.1021/acsnano.7b05762
Taukeer Khan, M., Khan, F., Al-Ahmed, A., Ahmad, S., and Al-Sulaiman, F. (2022). Evaluating the Capacitive Response in Metal Halide Perovskite Solar Cells. Chem. Rec., e202100330. doi:10.1002/tcr.202100330
Tress, W., Correa Baena, J. P., Saliba, M., Abate, A., and Graetzel, M. (2016). Inverted Current-Voltage Hysteresis in Mixed Perovskite Solar Cells: Polarization, Energy Barriers, and Defect Recombination. Adv. Energy Mat. 6 (19), 1600396. doi:10.1002/aenm.201600396
Tress, W., Yavari, M., Domanski, K., Yadav, P., Niesen, B., Correa Baena, J. P., et al. (2018). Interpretation and Evolution of Open-Circuit Voltage, Recombination, Ideality Factor and Subgap Defect States during Reversible Light-Soaking and Irreversible Degradation of Perovskite Solar Cells. Energy Environ. Sci. 11 (1), 151–165. doi:10.1039/c7ee02415k
Wetzelaer, G.-J. A. H., Scheepers, M., Sempere, A. M., Momblona, C., Ávila, J., and Bolink, H. J. (2015). Trap-Assisted Non-Radiative Recombination in Organic-Inorganic Perovskite Solar Cells. Adv. Mat. 27 (11), 1837–1841. doi:10.1002/adma.201405372
Wu, F., Pathak, R., Chen, K., Wang, G., Bahrami, B., Zhang, W.-H., et al. (2018). Inverted Current-Voltage Hysteresis in Perovskite Solar Cells. ACS Energy Lett. 3 (10), 2457–2460. doi:10.1021/acsenergylett.8b01606
Yang, G., Wang, C., Lei, H., Zheng, X., Qin, P., Xiong, L., et al. (2017). Interface Engineering in Planar Perovskite Solar Cells: Energy Level Alignment, Perovskite Morphology Control and High Performance Achievement. J. Mat. Chem. A 5 (4), 1658–1666. doi:10.1039/c6ta08783c
Yang, J. J., Strukov, D. B., and Stewart, D. R. (2013). Memristive Devices for Computing. Nat. Nanotech 8 (1), 13–24. doi:10.1038/nnano.2012.240
Yang, X., Xiong, Z., Chen, Y., Ren, Y., Zhou, L., Li, H., et al. (2020). A Self-Powered Artificial Retina Perception System for Image Preprocessing Based on Photovoltaic Devices and Memristive Arrays. Nano Energy 78, 105246. doi:10.1016/j.nanoen.2020.105246
Keywords: perovskite, memristor, inverted hysteresis, impedance spectroscopy, solar cell
Citation: Munoz-Diaz L, Rosa AJ, Bou A, Sánchez RS, Romero B, John RA, Kovalenko MV, Guerrero A and Bisquert J (2022) Inductive and Capacitive Hysteresis of Halide Perovskite Solar Cells and Memristors Under Illumination. Front. Energy Res. 10:914115. doi: 10.3389/fenrg.2022.914115
Received: 06 April 2022; Accepted: 30 May 2022;
Published: 19 July 2022.
Edited by:
Moritz H. Futscher, Swiss Federal Laboratories for Materials Science and Technology, SwitzerlandReviewed by:
Mengxia Liu, Yale University, United StatesCopyright © 2022 Munoz-Diaz, Rosa, Bou, Sánchez, Romero, John, Kovalenko, Guerrero and Bisquert. This is an open-access article distributed under the terms of the Creative Commons Attribution License (CC BY). The use, distribution or reproduction in other forums is permitted, provided the original author(s) and the copyright owner(s) are credited and that the original publication in this journal is cited, in accordance with accepted academic practice. No use, distribution or reproduction is permitted which does not comply with these terms.
*Correspondence: Antonio Guerrero, YWd1ZXJyZXJAdWppLmVz; Juan Bisquert, YmlzcXVlcnRAdWppLmVz
Disclaimer: All claims expressed in this article are solely those of the authors and do not necessarily represent those of their affiliated organizations, or those of the publisher, the editors and the reviewers. Any product that may be evaluated in this article or claim that may be made by its manufacturer is not guaranteed or endorsed by the publisher.
Research integrity at Frontiers
Learn more about the work of our research integrity team to safeguard the quality of each article we publish.