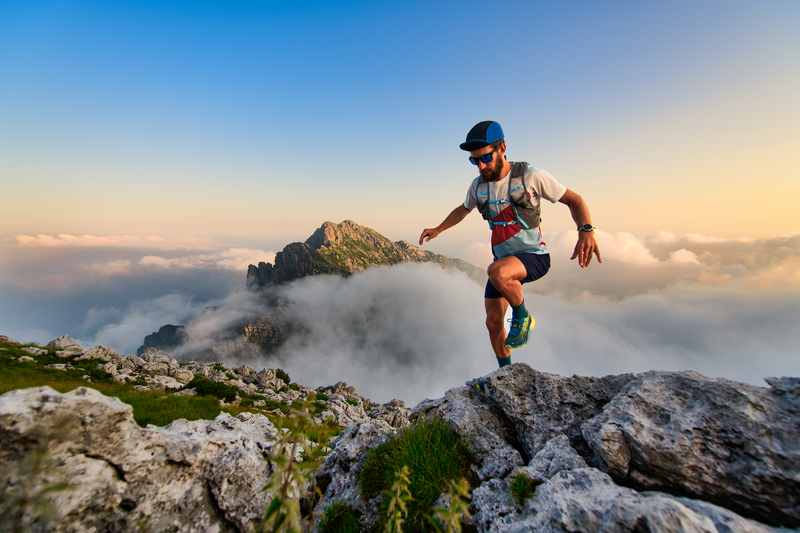
95% of researchers rate our articles as excellent or good
Learn more about the work of our research integrity team to safeguard the quality of each article we publish.
Find out more
BRIEF RESEARCH REPORT article
Front. Energy Res. , 28 June 2022
Sec. Bioenergy and Biofuels
Volume 10 - 2022 | https://doi.org/10.3389/fenrg.2022.912904
This article is part of the Research Topic Non-Conventional Organisms and Methods for Bioenergy Production Processes View all 5 articles
Numerous recent studies have identified microalgae biofuel as one of the major renewable energy sources for sustainable development due to their high biomass productivity, high lipid content, and availability of locally adapted strains in various geographical locations. There have been minimal studies on the fatty acid composition of lipid production on local microalgae species in Sabah, Malaysia. Thus, screening for local microalgae species capable of producing biodiesel can aid in the selection of suitable species. This study aimed to isolate and identify promising local microalga as biodiesel feedstock for mass cultivation. Eight microalgae species, Acutodesmus obliquus, Chaetoceros muelleri, Isochrysis galbana, Ankistrodesmus falcatus, Chlamydomonas monadina, Chlorella emersonii, Nannochloropsis oculata, and Tetraselmis chuii, were successfully isolated and identified from Kota Kinabalu, Sabah. The isolated microalgae were characterized based on the lipid/biomass productivity, lipid content and fatty acid profiles. These isolates had biomass productivity of 0.11–0.78 g/L/day, lipid content of 11.69–39.00% dry weight, and lipid productivity of 21.11–252.64 mg/L/day. According to GC-MS analyses, four isolates produced more than 80% of C14–C18 fatty acids, which were A. falcatus (95%), C. emersonii (93%), A. obliquus (91%), and C. muelleri (81%). Despite its low biomass productivity, C. muelleri was chosen as the best biodiesel species candidate because of its moderately high lipid productivity (42.90 mg/L/day), highest lipid content (39% dry weight), high level of MUFAs and C14–C18 FAs (81.47%), with the highest oleic acid proportion (28.38%), all of which are desirable characteristics for producing high-quality biodiesel.
Research on lipid production from microalgae such as diatoms as feedstock for biofuels began in Göttingen (Germany) during World War II, shortly after other nations (e.g., the United States, Japan, England, and Israel) expressed interest in growing microalgae, particularly species in the genus Chlorella, for a variety of applications including food and wastewater treatment (Borowitzka 2013). There are currently intensive global research efforts to explore and exploit potential microalgae for various applications ranging from nutraceuticals to alternative energy sources (Khan et al., 2018; Koyande et al., 2019). Biofuels have gained significant interest as a viable alternative energy source because, unlike fossil fuels, they are renewable, environmentally friendly, biodegradable, and sustainable (Singh et al., 2015; Jeswani et al., 2020). Biofuel is a fuel derived from biomass using modern techniques such as thermochemical (e.g., gasification, liquefaction, and pyrolysis) and biochemical (e.g., anaerobic digestion, alcoholic fermentation, and transesterification) transformation rather than the comparatively slow geological processes that result in the formation of fossil fuels (Ambaye et al., 2021). Microalgae receive much attention among the numerous potential biofuel feedstocks, and their benefits are well documented in the literature (Sharma et al., 2018; Hossain et al., 2020). In comparison to other biodiesel feedstocks such as rapeseed, soybean crops, and palm oil, microalgae accumulate higher lipid productivities and biomass without compromising the production of foods, fodder, and other crops-derived products, and they require considerably less land area for cultivation (Aratboni et al., 2019; Correa et al., 2020).
Based on energy demand, the most promising countries for microalgal biofuel production are in western South America, the Caribbean, the Middle East, North and East Africa, and Oceania (Correa et al., 2021); and species of the genera Chlorella, Dunaliella, Chlamydomonas, Scenedesmus, Tetraselmis, and Spirulina are among potential microalgae candidates composed of a large fraction of lipid, protein, and carbohydrates resulting in the driving factors of biofuel production (Show et al., 2017; Hossain et al., 2019). Despite significant advances, algae-based biodiesel remains expensive and laborious in industrial applications (Dębowski et al., 2020; Mohsenpour et al., 2021). These constraints could be solved by selecting resistant and productive species, optimizing growth conditions, creating innovative harvesting techniques, converting algal biomass to biodiesel using less energy-intensive processes and bio-refinery strategies (Culaba et al., 2020; Patnaik & Mallick 2021). The first step in producing biodiesel from microalgae is choosing suitable species with relevant traits and properties for possible large-scale cultivation adapted to local climatic conditions (Martín et al., 2020).
The type of lipid and fatty acid composition of the lipid-producing microalgal species affects biofuel quality (Aratboni et al., 2019; Morales et al., 2021). Microalgal lipids typically consist of structural lipids (polar lipids) and storage lipids (non-polar lipids). Triacylglycerols (TAGs), also known as neutral lipids, are esters made up of a glycerol molecule and three fatty acids (FAs), and they are the most common type of storage lipids (Chen and Wang 2021). Triacylglycerols containing lipids produced from microalgae are considered a potential alternative resource of biofuels because they are direct precursors of biodiesel via chemical transesterification (Pal et al., 2019). TAG production is even enhanced in unfavourable conditions that limit cell division and redirect photosynthetic energy to TAG formation (Morales et al., 2021). The three main types of FAs are saturated fatty acids (SFAs), monounsaturated fatty acids (MUFAs), and polyunsaturated fatty acids (PUFAs). They are classified according to the presence and quantity of double bonds in their carbon chain: SFA with no double bonds; MUFA with one double bond; and PUFA with two or more double bonds. When it comes to low temperature fluidity and oxidative stability, MUFAs are the finest components for biodiesel (Suastes-Rivas et al., 2020).
FAs are one of the primary metabolites of microalgae, enhancing their value as food and fuel (Pal et al., 2019; Sathasivam et al., 2019). The ability of microalgae to synthesize lipids, or more precisely, their FA production, should be a primary consideration when selecting microalgae as a source of oil for biodiesel (Aratboni et al., 2019). Most microalgae species contain myristic (C14:0), palmitic (C16:0), stearic (C18:0), oleic (C18:1), linoleic (C18:2) and linolenic (C18:3) acids (Shen et al., 2016; Deshmukh et al., 2019). According to Knothe (2008), most common biodiesel feedstocks consist of C14–C18 FAs, viz. palmitic acid (C16:0), stearic acid (C18:0), oleic acid (C18:1), linoleic acid (C18:2) and linolenic acid (C18:3ω3). Additionally, in later studies, Knothe added that significantly high amount of myristic acid (C14:0), palmitic acid (C16:0) and palmitoleic acid (C16:1) in an algal lipid will help in meeting the biodiesel standard requirement (Knothe 2011; Knothe 2013; Knothe and Razon 2017). Oils high in oleic acid (C18:1), in particular, have been observed to have a good fuel balance, including ignition quality, combustion heat, cold filter plugging point, oxidative stability, viscosity, and lubricity (Pugliese et al., 2020). As a result, the seven types of FAs stated above, which range from C14 to C18, are referred to as the desirable FAs in this study and are utilized as the reference FAs in establishing the suitability of an algal FAs profile for biodiesel generation.
With the proper biological and technological innovations, microalgae can provide renewable resources without causing adverse effects on agricultural land or water supplies. Finding the best species entails screening a wide range of microalgae and assessing their productivity. Tropical environments with high levels of solar radiation and saline water (either from the sea or groundwater) are ideal for mass microalgal cultivation (Hossain et al., 2020; Pugazhendhi et al., 2020). Sabah, located on the east coast of Malaysia, has the ideal climate for commercial microalgae cultivation. Local algal strains obtained from natural habitats and collected at various seasons are predicted to be the most adapted to the unique local conditions, making them ideal for large-scale cultivation. Considering the diversity of microalgae in a natural environment, exploitation of local species is more advantageous to the algal biofuel industry than the non-local species. Compared to the former, which has already evolved to be more adapted to cultivation sites, the latter may result in rapid culture contamination and complication (Guo et al., 2013). Any environmental impact caused by unintentional release from large-scale cultivation can also be reduced using local species (Mohsenpour et al., 2012). Many studies have demonstrated that local species are robust and competitive due to their ability to adapt to the local environmental changes, which are critical attributes for the success of large-scale production (Mutanda et al., 2020; Sero et al., 2021). Specifically, Chlorella spp. and Scenedesmus spp. isolated from water bodies in Minnesota (United States; Zhou et al., 2011), the Northern Territory (Australia; Duong et al., 2015), Faizabad (Pakistan; Kabir et al., 2020), and Owode Ede (Nigeria; Azeez et al., 2021) are among the most promising species that thrive in culture conditions similar to where they are found and adapted, offering an economically viable and environmentally friendly means for sustainable renewable algal energy production.
Expanding microalgae culture from the laboratory to the commercial scale will always face challenges related to the adaptation of operating conditions, including environmental and climatic conditions (Yahya et al., 2020); thus, in the search for potential biofuel candidates, the selection of fast-growing, productive strains adapted to local climatic conditions is critical to expedite the acclimatization period and the success of any algal mass culture (Mutanda et al., 2020). To date, there have been limited studies focusing on identifying and evaluating native microalgae species appropriate for mass production in Sabah, a state of Malaysia with a diverse range of microalgal strains. Microalgae feedstock production necessitates a high lipid content with the desired FA composition suitable for biodiesel conversion. The current study aims to identify the most promising oil-producing microalgae candidate by screening the available isolates native to Sabah on lipid and biomass productivities and FA compositions based on prior knowledge and a hypothesis that microalgae thrive in culture conditions similar to where they are found and adapted. The findings will aid in discovering microalgae species capable of being grown in large-scale cultures, potentially paving the way for commercialization.
All components of the culture media (Supplementary Files 1, 2) were purchased from Merck KgaA, Darmstadt, Germany. Molecular grade reagents were obtained from QIAGEN Biotechnology Sdn. Bhd. (Kuala Lumpur, Malaysia), including Taq DNA polymerase, magnesium chloride (MgCl2), and deoxynucleoside triphosphates (dNTPs). Analytical grade chemical reagents were obtained from local supplier, including sodium sulfate (NaSO4), methanol, chloroform. Reagents used for gas chromatography-mass spectrometry (GC-MS) were purchased from Thermo Fisher Scientific Inc. (United States), including sulphuric acid (H2SO4), sodium chloride solution (NaCl), and hexane. Helium gas used in GC-MS analysis was purchased from local supplier.
Water samples were collected from different freshwaters and coastal ecological areas in Kota Kinabalu, Sabah. In this study, Bold’s Basal Medium (Bischoff and Bold 1963) (Supplementary File 1) was used to culture freshwater algal species (NFW1–NFW4), while Walne’s Medium (Walne 1970) (Supplementary File 2) was used to culture marine species (SW1–SW4). Microalgae were isolated using the micropipette washing technique (Tsuchikane et al., 2018). The isolated microalgae were identified based on the 18S ribosomal RNA gene using the primer pair, 18SCOMF1 (5′-GCTTGTCTCAAAGATTAAGCCATGC-3′) and 18SCOMR1 (5′- CACCTACGGAAACCTTGTTACGAC-3′) (Zhang et al., 2005). The isolated marine species (SW1–SW4) were identified as Chaetoceros muelleri (SW1), Isochrysis galbana (SW2), Nannochloropsis oculata (SW3), and Tetraselmis chuii (SW4), whereas for the freshwater species were Ankistrodesmus falcatus (NFW1), Chlorella emersonii (NFW2), Chlamydomonas monadina (NFW3), and Acutodesmus obliquus (= Scenedesmus obliquus) (NFW4). All experiments were conducted in triplicates (n = 3) in 1 L flasks containing 500 ml of respective culture medium and inoculated with 10% actively growing algal isolates. The experiments were carried out in batch cultures at a temperature of 23 ± 2°C with a 16:8 light/dark photoperiod and a light intensity of 68 μmol/m2/s supplied by cool-white, fluorescent tubes. Table 1 shows the overall details of the microalgae isolated in this study.
The estimation of growth characteristics for the isolates was based on number of cells in the culture per volume (ml) after 30 days of cultivation. Counting of cells was carried out on the day of inoculation and every 3 days after the initial inoculation for 30 days using haemocytometer (Sambrook and Russell, 2001). Motile algal cells were immobilized with one to two drops of 10% formalin. Approximately 50 μl of algal culture was slowly dispensed onto both wells of haemacytometer under the cover slip. Both sides of the haemacytometer were counted using a light microscope. Cells density per volume (ml) was calculated based on Eq. 1 and used to plot the growth curve of each isolate.
For determination of biomass productivity, algal cultures were harvested during early (day 3) and mid (day 18) exponential phase. For harvesting, approximately 48 ml of each culture was centrifuged at 3,400 × g for 5 min. The pellet was rinsed twice with distilled water and freeze-dried before being ground into powder. The biomass productivity was measured gravimetrically and determined using Eq. 2 (Nascimento et al., 2012), where the growth was expressed in dry weight gram per liter per day (g/L/day).
Where, X1 and X2 were the biomass dry weight concentrations on day t1 (start point of cultivation) and t2 (end point of cultivation), respectively, and was given as an average productivity.
For determination of lipid content, the algal cultures were harvested during the mid-exponential phase (day 18). The chloroform: methanol (2:1) extraction method was used to extract and determine the lipid content of the microalgal cultures (Folch et al., 1957; Ryckebosch et al., 2011). The solvent was removed using a vacuum rotary evaporator after the extraction for about 20 min. The amount of lipid recovered (% of lipid) was determined gravimetrically using Eq. 3 by Cordeiro et al., 2017. The lipid productivity (mg/L/day) of each microalgae species was determined by multiplying the lipid content value by the biomass productivity value (Nascimento et al., 2012).
The FA compositions were analyzed using the method of Yong et al. (2015). Dried algal biomass (approximately 100 mg) was diluted in 1 ml hexane before being mixed with 3 ml of 5% hydrogen chloride methanol. After agitating the mixture for about 3 h, a drop of water was added to cease the reaction. A biphasic layer was formed, and the upper methyl ester-containing layer (hexane) was collected and dried using a rotary evaporator. After dissolving the transformed FAs in 3 ml of hexane, the transformed FAs were filtered through a 0.2-μm syringe filter. All samples were analyzed using an Agilent 7890A GC system coupled with an Agilent 5975C mass spectrometry detector. A capillary column HP-5MS (30 m × 0.25 mm) of 0.25 µm film thickness of coated material was used. Injector temperature was set at 250°C, whereas the temperature programme was as follow: start at 90°C and hold for 3 min; from 90 to 180°C with 3°C/min, hold for 5 min, from 180 to 290°C with 3°C/min and then hold for 15 min. A post-run of 10 min at 90°C was sufficient for the next injection. Gas chromatography was performed in a split mode with ratio of 100:1. Helium gas was used as carrier gas and maintained at 1.0 ml/min of constant flow rate. The total run time was calculated to be 25 min per sample. Identification of compounds was carried out by comparing with the spectral data obtained from the National Institute of Standards and Technology (NIST) library, whereas the compositions were computed with reference to the abundance of the compounds in chromatogram. The individual peak area was used to calculate the FA compositions, and the percentages of each type of FA (SFA, MUFA, and PUFA) were calculated by adding the total peak areas of each individual peak.
The Statistical Package for the Social Sciences (SPSS) software version 21.0 (Chicago, United States) was used to conduct the statistical analyses. Analyses of variance (ANOVA) were performed on all data sets. Significant differences were defined as those with a p-value of less than 0.05.
The initial and final cell counts, and the growth curves of the microalgae are shown in Figure 1A and Supplementary File 3, respectively. All cultures underwent a short lag phase, followed by an exponential phase from day 3 onwards. The exponential phase lasted up to day 21/24, except for C. emersonii and N. oculata, which experienced a more prolonged phase until day 30. When the algal cultures were harvested on day 18 (mid-exponential phase), the cell density of the isolates had increased 12 to 73-fold compared to the initial cell count of the inoculum, with N. oculata, I. galbana and C. muelleri having the top three highest growth. The top three candidate species with the highest biomass productivity were C. emersonii, A. falcatus, and T. chuii, with 0.78, 0.33, and 0.32 g/L/day, respectively (Figure 1B). The biomass productivity of the other five isolates, C. monadina, A. obliquus, I. galbana, C. muelleri, and N. oculata, was lower, ranging from 0.11 to 0.23 g/L/day. Previous research on the same genera, Ankistrodesmus, Chlorella, Chlamydomonas, Scenedesmus, Chaetoceros, Isochrysis, Nannochloropsis, and Tetraselmis, showed biomass productivity ranging from 0.13 to 0.56 g/L/day (Table 2).
FIGURE 1. (A) Initial and final cell count (cells/ml), (B) biomass productivity (g/L/day), (C) lipid content (in % dry weight), (D) lipid productivity (mg/L/day), and (E) fatty acid profile of the isolates. NFW1: A. falcatus, NFW2: C. emersonii, NFW3: C. monadina, NFW4: A. obliquus, SW1: C. muelleri, SW2: I. galbana, SW3: N. oculata, and SW4: T. chuii. The data represent the mean of the replicate experiments (n = 3), and the error bars represent standard deviation.
TABLE 2. Biomass productivity, lipid content, and lipid productivity of different microalgae species as reported in the literature.
Many microalgae species can produce significant amounts of oil, with the average oil content ranging from 1 to 70%, and algae with 30–75% lipid by dry basis can be referred to as oilgae (Demirbas, 2011). Nonetheless, certain species can store up to 90% oil of dry lipid (Tabatabaei et al., 2011; Pal et al., 2019). Lipid accumulation is defined as an increase in the lipid concentrations within the algal cells without considering the overall biomass production (Brennan and Owende, 2010; Liu et al., 2011; Aratboni et al., 2019). Figure 1C depicts the remarkable ability of microalgae to accumulate lipid in concentrations ranging from 11 to 39% dry weight (DW). Three isolates had lipid contents that exceeded 30%, namely C. muelleri (39% DW), C. emersonii (32% DW), and I. galbana (30% DW). Among the top biomass producers (Figure 1D), C. emersonii and I. galbana are top biomass producers. Chaetoceros muelleri, on the other hand, despite having the highest lipid content, produced the least biomass. Previous research has found an inverse relationship between biomass and lipid productivity in microalgae, as observed in Isochrysis zhangjiangensis (Feng et al., 2011) and Chlorella sp. (Cho et al., 2020) when cultured in a nitrogen-rich environment.
Lower growth rates in microalgae species often compensate for high lipid content. While certain algae have oil contents of up to 80% but slow growth rates (Khan et al., 2018), the marine algae Tetraselmis suecica and the freshwater algae Chlorophyta sorokiniana have rapid growth rates but low lipid content (Griffiths and Harrison 2009). Increased lipid content does not always increase lipid productivity; instead, it reduces biomass and lipid productivity. Thus, lipid productivity should be the prerequisite factor before examining FA profile in facilitating species selection for biodiesel production because it is highly correlated with biomass productivity, confirming its effectiveness as a biodiesel production indicator. (Vello et al., 2014; Zhao et al., 2014). The lipid productivities of the isolates in this study ranged from 25 to 253 mg/L/day, with the top four species having high lipid productivities being C. emersonii (252 mg/L/day), I. galbana (87.33 mg/L/day), A. falcatus (56.10 mg/L/day), and C. muelleri (42.90 mg/L/day). These lipid contents and productivities are among the highest values reported in the literature for the microalgae species with biodiesel production potential (Table 2). However, to give a realistic basis for comparison, cultivation and production may need to occur under the same conditions.
Along with high biomass productivity and intrinsic cellular lipid content, the FA profile of microalgae is critical since it ultimately determines the quality of the biodiesel product (Morales et al., 2021). The qualities of biodiesel fuel obtained from any lipid feedstock can be used to assess the viability of any lipid feedstock for biodiesel production (Knothe 2005; Griffiths et al., 2011; Hossain et al., 2020). Fatty acids in the eight isolated microalgae species (A. falcatus, C. monadina, C. emersonii, A. obliquus, C. muelleri, I. galbana, N. oculata, and T. chuii) were primarily esterified, and the main FA composition of each isolate is shown in Table 3. The FA profiles of the isolates revealed the presence of myristic (C14:0), pentadecyclic (C15:0), palmitic (C16:0), margaric (C17:0), stearic (C18:0), palmitoleic (C16:1), oleic (C18:1n9c), α-linolenic (C18:3n3), and linoleic (C18:2) acids, as well as eicosapentaenoic acid (EPA; C20:5ω3), eicosatetraenoic acid (ETA; C20:4ω6), and docosahexaenoic acid (DHA; C22:6ω3). The major FAs in the freshwater isolates were palmitic, linoleic, and linolenic acids, which accounted for 21–32%, 4–14%, and 45–55% of the total FAs, respectively. Statistical analyses revealed significant differences (p < 0.05) in the C14–C18 FAs among the freshwater isolates. Myristic, palmitic, palmitoleic, and oleic acids were the primary FAs discovered in the marine isolates, accounting for 0.5–34%, 16–25%, 2–32%, and 4–28% of the total FAs, respectively, whereas the other FAs were minors. Statistical analysis indicated no significant differences (p > 0.05) in the C14–C18 FAs among the marine isolates. Among all the freshwater and marine species, four species recorded C14–C18 FAs of exceeding 80%, namely A. falcatus (95%), C. emersonii (93%), A. obliquus (91%), and C. muelleri (81%).
TABLE 3. Fatty acid profile of microalgae isolates (% of total fatty acid methyl esters), with the desired MUFA, oleic acid (C18:1), highlighted in bold.
In addition to the amount of C14–C18 FAs, the amount of SFAs, MUFAs, and PUFAs in respective FA profiles is also an essential factor to consider for biodiesel production. An ideal FA profile includes a low level of PUFAs and a high level of MUFAs. Both marine C. muelleri and I. galbana isolates met the criteria by having a comparatively low level of PUFAs and a higher level of MUFAs (Figure 1E). FA profiles with high PUFAs are unfavourable for biodiesel production because PUFAs are highly susceptible to oxidation (Siaut et al., 2011; Halim et al., 2012; Bounnit et al., 2020). Thus, microalgae with a high MUFA content and low PUFA content are desirable for biodiesel production (James et al., 2013; Morales et al., 2021). Among the tested microalgae species, C. muelleri had the highest oleic acid proportion (28.38 ± 1.22%), indicating the most suitable isolate for producing high-quality biodiesel. Other isolates had relatively low levels of oleic acid, ranging from 0.4 to 8.4%. Mahmoud et al. (2015) had evaluated the potential for 15 microalgal species for biodiesel production, and among all the isolates, three microalgae, Chlorella vulgaris, Scenedesmus quadricauda and Trachelomonas oblonga were identified as the most potent isolates. The three isolates had growth rate suitable for high-density cultures, high lipid content of 29–37%, and possessed the recommended unsaturated FAs, such as palmitoleic acid (C16:1), oleic acid (C18:1), culate acid (C18:2) and linolenic (C18:3). However, S. quadricauda was chosen as the most suitable species for the production of good-quality biodiesel because it had the highest oleic acid content (25.97%), as compared to the other two microalgae with only 6.80–8.42%. The high concentration of oleic acid ester is a highly advantageous property that allows for an intriguing trade-off between oxidative stability and low-temperature performance, thus improving biodiesel quality (Wang et al., 2020).
Although C. emersonii (NFW2) produced the highest biomass productivity (0.78 g/L/day) and comparable lipid content (32% dry weight) and highest productivity (252 mg/L/day), the species was not recommended as the suitable candidate due to lower MUFA (11.81%) and higher PUFA (66.51%) levels, a reverse characteristic of biofuel production recommendation, and a relatively low concentration of oleic acid (8.40 ± 0.25% of total fatty acids), which is a critical component of biodiesel for good ignition quality, combustion heat, oxidative stability, and viscosity. Despite having moderately high biomass productivity of 0.29 g/L/day, lipid content of 30.12% dry weight, and lipid productivity of 87.33 mg/L/day (second highest), as well as high MUFA and low PUFA levels, another microalgal species, I. galbana, was not recommended as a potential biodiesel species due to its low C14–C18 level of only 51.50% (second lowest among the other species). As a result, among the eight microalgae, C. muelleri was selected as the desired biodiesel species as it had all the essential advantageous characteristics for producing high-quality biodiesel, particularly having the highest oleic acid level (28.38%). Besides the high level of oleic acid, the selected microalga also has a moderately high lipid productivity (42.90 mg/L/day), the highest lipid content of 39% dry weight, 81.47% of C14–C18 FAs, as well as the recommended FA composition (low PUFA and high MUFA levels).
In this study, the economic feasibility of microalgal oils for biodiesel production is determined by screening and evaluating possible local microalgal strains for biomass productivity, lipid cell content, and FA composition. The study was limited to only screening of eight cultivable microalgae species. The results of this study indicate that the naturally isolated microalga, C. muelleri, is a valuable candidate for use in biodiesel production. This marine species produced the highest lipid contents (39% dry weight) with C14–C18 FAs (81%), the highest oleic acid proportion (28%), and the ideal composition of MUFAs/PUFAs. Although C. muelleri produced only moderate biomass and lipid productivity levels, its FA profile was significantly more favourable than other isolates, implying that the species possessed desirable characteristics for large-scale biomass production for biodiesel feedstock. This study contributes to the discovery of tropical microalgae species capable of being used for renewable algal energy production, paving the way for future bioprospecting to harness local biodiversity to address sustainable bioindustrial needs.
The original contributions presented in the study are included in the article/Supplementary Material, further inquiries can be directed to the corresponding author.
AA and GC conceived this research and designed experiments; ARA, WY, and MM participated in the design and interpretation of the data; ARA and GC performed experiments and analysis; ARA and WY wrote the first draft of the manuscript, and all authors participated in the revisions of it. All authors read and approved the final manuscript.
This research was funded by the Universiti Malaysia Sabah under the Priority Field Research Grant Scheme (SBK0385-2018) and the Malaysian Ministry of Higher Education (MOHE) under the Fundamental Research Grant Scheme (FRG0335-STWN-1/2013).
The authors declare that the research was conducted in the absence of any commercial or financial relationships that could be construed as a potential conflict of interest.
All claims expressed in this article are solely those of the authors and do not necessarily represent those of their affiliated organizations, or those of the publisher, the editors and the reviewers. Any product that may be evaluated in this article, or claim that may be made by its manufacturer, is not guaranteed or endorsed by the publisher.
The authors gratefully acknowledge the financial support from the Universiti Malaysia Sabah through the Priority Field Research Grant Scheme (SBK0385-2018) and the Malaysian Ministry of Higher Education (MOHE) through the Fundamental Research Grant Scheme (FRG0335-STWN-1/2013).
The Supplementary Material for this article can be found online at: https://www.frontiersin.org/articles/10.3389/fenrg.2022.912904/full#supplementary-material
Supplementary File 1 | Bold’s Basal Medium (BBM) Recipe (Bischoff and Bold, 1963).
Supplementary File 2 | Walne’s Medium Recipe (Walne, 1970).
Supplementary File 3 | Growth curves of the isolated microalgae.
Abou-Shanab, R. A. I., Ji, M.-K., Kim, H.-C., Paeng, K.-J., and Jeon, B.-H. (2013). Microalgal Species Growing on Piggery Wastewater as a Valuable Candidate for Nutrient Removal and Biodiesel Production. J. Environ. Manag. 115, 257–264. doi:10.1016/j.jenvman.2012.11.022
Alishah Aratboni, H., Rafiei, N., Garcia-Granados, R., Alemzadeh, A., and Morones-Ramírez, J. R. (2019). Biomass and Lipid Induction Strategies in Microalgae for Biofuel Production and Other Applications. Microb. Cell Fact. 18, 178. doi:10.1186/s12934-019-1228-4
Ambaye, T. G., Vaccari, M., Bonilla-Petriciolet, A., Prasad, S., van Hullebusch, E. D., and Rtimi, S. (2021). Emerging Technologies for Biofuel Production: a Critical Review on Recent Progress, Challenges and Perspectives. J. Environ. Manag. 290, 112627. doi:10.1016/j.jenvman.2021.112627
Azeez, N. A., Oyelami, S., Adekanmi, A. A., Ologunye, O. B., Adedigba, S. A., Akinola, O. J., et al. (2021). Biodiesel Potentials of Microalgal Strains Isolated from Fresh Water Environment. Environ. Challenges 5, 100367. doi:10.1016/j.envc.2021.100367
Bischoff, H. W., and Bold, H. C. (1963). Some Soil Algae from Enchanted Rock and Related Algal speciesPhycological Studies IV. Austin: University of Texas Publication.
Borowitzka, M. A. (2013). “Energy from Microalgae: a Short History,” in Algae For Biofuels And Energy, Developments in Applied Phycology. Editors M. A. Borowitzka, and N. R. Moheimani (Dordrecht: Springer), Vol. 5, 1–15. doi:10.1007/978-94-007-5479-9_1
Bounnit, T., Saadaoui, I., Rasheed, R., Schipper, K., Al Muraikhi, M., and Al Jabri, H. (2020). Sustainable Production of Nannochloris Atomus Biomass towards Biodiesel Production. Sustainability 12 (5), 2008. doi:10.3390/su12052008
Brennan, L., and Owende, P. (2010). Biofuels from Microalgae-A Review of Technologies for Production, Processing, and Extractions of Biofuels and Co-products. Renew. Sustain. Energy Rev. 14 (2), 557–577. doi:10.1016/j.rser.2009.10.009
Chen, H., and Wang, Q. (2021). Regulatory Mechanisms of Lipid Biosynthesis in Microalgae. Biol. Rev. 96 (5), 2373–2391. doi:10.1111/brv.12759
Cho, J. M., Oh, Y.-K., Park, W.-K., and Chang, Y. K. (2020). Effects of Nitrogen Supplementation Status on CO2 Biofixation and Biofuel Production of the Promising Microalga Chlorella Sp. ABC-001. J. Microbiol. Biotechnol. 30 (8), 1235–1243. doi:10.4014/jmb.2005.05039
Cordeiro, R. S., Vaz, I. C. D., Magalhães, S. M. S., and Barbosa, F. A. R. (2017). Effects of Nutritional Conditions on Lipid Production by Cyanobacteria. Acad Bras Cienc 89 (3), 2021–2031. doi:10.1590/0001-3765201720150707
Correa, D. F., Beyer, H. L., Possingham, H. P., Fargione, J. E., Hill, J. D., and Schenk, P. M. (2021). Microalgal Biofuel Production at National Scales: Reducing Conflicts with Agricultural Lands and Biodiversity within Countries. Energy 215 (A), 119033. doi:10.1016/j.energy.2020.119033
Correa, D. F., Beyer, H. L., Possingham, H. P., García-Ulloa, J., Ghazoul, J., and Schenk, P. M. (2020). Freeing Land from Biofuel Production through Microalgal Cultivation in the Neotropical Region. Environ. Res. Lett. 15 (9), 094094. doi:10.1088/1748-9326/ab8d7f
Culaba, A. B., Ubando, A. T., Ching, P. M. L., Chen, W.-H., and Chang, J.-S. (2020). Biofuel from Microalgae: Sustainable Pathways. Sustainability 12 (19), 8009. doi:10.3390/su12198009
De Morais, M. G., and Costa, J. A. V. (2007). Biofixation of Carbon Dioxide by Spirulina Sp. And Scenedesmus Obliquus Cultivated in a Three-Stage Serial Tubular Photobioreactor. J. Biotechnol. 129 (3), 439–445. doi:10.1016/j.jbiotec.2007.01.009
Dębowski, M., Zieliński, M., Kazimierowicz, J., Kujawska, N., and Talbierz, S. (2020). Microalgae Cultivation Technologies as an Opportunity for Bioenergetic System Development — Advantages and Limitations. Sustainability 12, 9980. doi:10.3390/su12239980
Demirbas, A. (2011). Biodiesel from Oilgae, Biofixation of Carbon Dioxide by Microalgae: a Solution to Pollution Problems. Appl. Energy 88 (10), 3541–3547. doi:10.1016/j.apenergy.2010.12.050
Deshmukh, S., Bala, K., and Kumar, R. (2019). Selection of Microalgae Species Based on Their Lipid Content, Fatty Acid Profile and Apparent Fuel Properties for Biodiesel Production. Environ. Sci. Pollut. Res. 26 (24), 24462–24473. doi:10.1007/s11356-019-05692-z
Duong, V. T., Ahmed, F., Thomas-Hall, S. R., Quigley, S., Nowak, E., and Schenk, P. M. (2015). High Protein- and High Lipid-Producing Microalgae from Northern Australia as Potential Feedstock for Animal Feed and Biodiesel. Front. Bioeng. Biotechnol. 3, 53. doi:10.3389/fbioe.2015.00053
Feng, D., Chen, Z., Xue, S., and Zhang, W. (2011). Increased Lipid Production of the Marine Oleaginous Microalgae Isochrysis Zhangjiangensis (Chrysophyta) by Nitrogen Supplement. Bioresour. Technol. 102 (12), 6710–6716. doi:10.1016/j.biortech.2011.04.006
Folch, J., Lees, M., and Stanley, G. H. S. (1957). A Simple Method for the Isolation and Purification of Total Lipides from Animal Tissues. J. Biol. Chem. 226, 497–509. doi:10.1016/s0021-9258(18)64849-5
George, B., Pancha, I., Desai, C., Chokshi, K., Paliwal, C., Ghosh, T., et al. (2014). Effects of Different Media Composition, Light Intensity and Photoperiod on Morphology and Physiology of Freshwater Microalgae Ankistrodesmus Falcatus - A Potential Strain for Bio-Fuel Production. Bioresour. Technol. 171, 367–374. doi:10.1016/j.biortech.2014.08.086
Griffiths, M. J., and Harrison, S. T. L. (2009). Lipid Productivity as a Key Characteristic for Choosing Algal Species for Biodiesel Production. J. Appl. Phycol. 21 (5), 493–507. doi:10.1007/s10811-008-9392-7
Griffiths, M. J., van Hille, R. P., and Harrison, S. T. L. (2011). Lipid Productivity, Settling Potential and Fatty Acid Profile of 11 Microalgal Species Grown under Nitrogen Replete and Limited Conditions. J. Appl. Phycol. 24 (5), 989–1001. doi:10.1007/s10811-011-9723-y
Guo, H., Daroch, M., Liu, L., Qiu, G., Geng, S., and Wang, G. (2013). Biochemical Features and Bioethanol Production of Microalgae from Coastal Waters of Pearl River Delta. Bioresour. Technol. 127, 422–428. doi:10.1016/j.biortech.2012.10.006
Halim, R., Danquah, M. K., and Webley, P. A. (2012). Extraction of Oil from Microalgae for Biodiesel Production: a Review. Biotechnol. Adv. 30 (3), 709–732. doi:10.1016/j.biotechadv.2012.01.001
Hossain, N., Hasan, M. H., Mahlia, T. M. I., Shamsuddin, A. H., and Silitonga, A. S. (2020). Feasibility of Microalgae as Feedstock for Alternative Fuel in Malaysia: a Review. Energy Strategy Rev. 32, 100536. doi:10.1016/j.esr.2020.100536
Hossain, N., Mahlia, T. M. I., and Saidur, R. (2019). Latest Development in Microalgae-Biofuel Production with Nano-Additives. Biotechnol. Biofuels 12, 125. doi:10.1186/s13068-019-1465-0
Abdelkhalek, E. A. I., Mohamed, B., Mohammed, A. M., and Lotfi, A. (2016). Growth Performance and Biochemical Composition of Nineteen Microalgae Collected from Different Moroccan Reservoirs. Medit. Mar. Sci. 17, 323–332. doi:10.12681/mms.1320
James, G. O., Hocart, C. H., Hillier, W., Price, G. D., and Djordjevic, M. A. (2013). Temperature Modulation of Fatty Acid Profiles for Biofuel Production in Nitrogen Deprived Chlamydomonas Reinhardtii. Bioresour. Technol. 127, 441–447. doi:10.1016/j.biortech.2012.09.090
Jeswani, H. K., Chilvers, A., and Azapagic, A. (2020). Environmental Sustainability of Biofuels: a Review. Proc. R. Soc. A 476, 20200351. doi:10.1098/rspa.2020.0351
Kabir, F., Gulfraz, M., Raja, G. K., Inam-ul-Haq, M., Awais, M., Mustafa, M. S., et al. (2020). Screening of Native Hyper-Lipid Producing Microalgae Strains for Biomass and Lipid Production. Renew. Energy 160, 1295–1307. doi:10.1016/j.renene.2020.07.004
Khan, M. I., Shin, J. H., and Kim, J. D. (2018). The Promising Future of Microalgae: Current Status, Challenges, and Optimization of a Sustainable and Renewable Industry for Biofuels, Feed, and Other Products. Microb. Cell Fact. 17, 36. doi:10.1186/s12934-018-0879-x
Knothe, G. (2008). "Designer" Biodiesel: Optimizing Fatty Ester Composition to Improve Fuel Properties. Energy fuels. 22 (2), 1358–1364. doi:10.1021/ef700639e
Knothe, G. (2011). A Technical Evaluation of Biodiesel from Vegetable Oils vs. Algae. Will Algae-Derived Biodiesel Perform? Green Chem. 13 (11), 3048–3065. doi:10.1039/C0GC00946F
Knothe, G. (2005). Dependence of Biodiesel Fuel Properties on the Structure of Fatty Acid Alkyl Esters. Fuel Process. Technol. 86 (10), 1059–1070. doi:10.1016/j.fuproc.2004.11.002
Knothe, G. (2013). “Production and Properties of Biodiesel from Algal Oils,” in Algae for Biofuels and EnergyDevelopments in Applied Phycology,. Editors M. Borowitzka, and N. Moheimani (Dordrecht: Springer), Vol. 5. doi:10.1007/978-94-007-5479-9_12
Knothe, G., and Razon, L. F. (2017). Biodiesel Fuels. Prog. Energy Combust. Sci. 58, 36–59. doi:10.1016/j.pecs.2016.08.001
Koyande, A. K., Chew, K. W., Rambabu, K., Tao, Y., Chu, D.-T., and Show, P.-L. (2019). Microalgae: a Potential Alternative to Health Supplementation for Humans. Food Sci. Hum. Wellness 8 (1), 16–24. doi:10.1016/j.fshw.2019.03.001
Kumar, V., Kumar, R., Rawat, D., and Nanda, M. (2018). Synergistic Dynamics of Light, Photoperiod and Chemical Stimulants Influences Biomass and Lipid Productivity in Chlorella Singularis (UUIND5) for Biodiesel Production. Appl. Biol. Chem. 61 (1), 7–13. doi:10.1007/s13765-017-0332-6
Liu, J., Huang, J., and Chen, F. (2011). “Microalgae as Feedstocks for Biodiesel Production,” in Biodiesel – Feedstocks and Processing Technologies. Editors M. Stoytcheva, and G. Montero (IntechOpen, 133–160. doi:10.5772/25600
Mahmoud, E. A., Farahat, L. A., Abdel Aziz, Z. K., Fatthallah, N. A., and Salah El Din, R. A. (2015). Evaluation of the Potential for Some Isolated Microalgae to Produce Biodiesel. Egypt. J. Petroleum 24 (1), 97–101. doi:10.1016/j.ejpe.2015.02.010
Martín, L. A., Popovich, C. A., Damiani, M. C., and Leonardi, P. I. (2020). A Practical Tool for Selecting Microalgal Species for Biodiesel Production. J. Renew. Sustain. Energy 12, 063101. doi:10.1063/5.0010668
Metsoviti, M. N., Papapolymerou, G., Karapanagiotidis, I. T., and Katsoulas, N. (2019). Comparison of Growth Rate and Nutrient Content of Five Microalgae Species Cultivated in Greenhouses. Plants 8 (8), 279. doi:10.3390/plants8080279
Mohsenpour, S. F., Hennige, S., Willoughby, N., Adeloye, A., and Gutierrez, T. (2021). Integrating Micro-algae into Wastewater Treatment: a Review. Sci. Total Environ. 752, 142168. doi:10.1016/j.scitotenv.2020.142168
Mohsenpour, S. F., Richards, B., and Willoughby, N. (2012). Spectral Conversion of Light for Enhanced Microalgae Growth Rates and Photosynthetic Pigment Production. Bioresour. Technol. 125, 75–81. doi:10.1016/j.biortech.2012.08.072
Morales, M., Aflalo, C., and Bernard, O. (2021). Microalgal Lipids: a Review of Lipids Potential and Quantification for 95 Phytoplankton Species. Biomass Bioenergy 150, 106108. doi:10.1016/j.biombioe.2021.106108
Mutanda, T., Naidoo, D., Bwapwa, J. K., and Anandraj, A. (2020). Biotechnological Applications of Microalgal Oleaginous Compounds: Current Trends on Microalgal Bioprocessing of Products. Front. Energy Res. 8, 598803. doi:10.3389/fenrg.2020.598803
Nascimento, I. A., Marques, S. S. I., Cabanelas, I. T. D., Pereira, S. A., Druzian, J. I., de Souza, C. O., et al. (2012). Screening Microalgae Strains for Biodiesel Production: Lipid Productivity and Estimation of Fuel Quality Based on Fatty Acids Profiles as Selective Criteria. Bioenerg. Res. 6 (1), 1–13. doi:10.1007/s12155-012-9222-2
Pal, P., Chew, K. W., Yen, H.-W., Lim, J. W., Lam, M. K., and Show, P. L. (2019). Cultivation of Oily Microalgae for the Production of Third-Generation Biofuels. Sustainability 11 (19), 5424. doi:10.3390/su11195424
Patnaik, R., and Mallick, N. (2021). Microalgal Biodiesel Production: Realizing the Sustainability Index. Front. Bioeng. Biotechnol. 9, 620777. doi:10.3389/fbioe.2021.620777
Pugazhendhi, A., Nagappan, S., Bhosale, R. R., Tsai, P.-C., Natarajan, S., Devendran, S., et al. (2020). Various Potential Techniques to Reduce the Water Footprint of Microalgal Biomass Production for Biofuel-A Review. Sci. Total Environ. 749, 142218. doi:10.1016/j.scitotenv.2020.142218
Pugliese, A., Biondi, L., Bartocci, P., and Fantozzi, F. (2020). Selenastrum Capricornutum a New Strain of Algae for Biodiesel Production. Fermentation 6 (2), 46. doi:10.3390/fermentation6020046
Renaud, S. M., Thinh, L. V., Lambrinidis, G., and Parry, D. L. (2002). Effect of Temperature on Growth, Chemical Composition and Fatty Acid Composition of Tropical Australian Microalgae Grown in Batch Cultures. Aquaculture 211 (1-4), 195–214. doi:10.1016/s0044-8486(01)00875-4
Rodolfi, L., Chini Zittelli, G., Bassi, N., Padovani, G., Biondi, N., Bonini, G., et al. (2009). Microalgae for Oil: Strain Selection, Induction of Lipid Synthesis and Outdoor Mass Cultivation in a Low-Cost Photobioreactor. Biotechnol. Bioeng. 102 (1), 100–112. doi:10.1002/bit.22033
Ryckebosch, E., Muylaert, K., and Foubert, I. (2011). Optimization of an Analytical Procedure for Extraction of Lipids from Microalgae. J. Am. Oil Chem. Soc. 89 (2), 189–198. doi:10.1007/s11746-011-1903-z
Sambrook, J., and Russell, D. W. (2001). Molecular Cloning: A Laboratory Manual. New York, USA: Cold Spring Harbour Laboratory Press.
Sathasivam, R., Radhakrishnan, R., Hashem, A., and Abd_Allah, E. F. (2019). Microalgae Metabolites: a Rich Source for Food and Medicine. Saudi J. Biol. Sci. 26 (4), 709–722. doi:10.1016/j.sjbs.2017.11.003
Sero, E. T., Siziba, N., Bunhu, T., and Shoko, R. (2021). Isolation and Screening of Microalgal Species, Native to Zimbabwe, with Potential Use in Biodiesel Production. All Life 14 (1), 256–264. doi:10.1080/26895293.2021.1911862
Sharma, A. K., Sahoo, P. K., Singhal, S., and Patel, A. (2016). Impact of Various Media and Organic Carbon Sources on Biofuel Production Potential from Chlorella Spp. 3 Biotech. 6, 116. doi:10.1007/s13205-016-0434-6
Sharma, P. K., Saharia, M., Srivstava, R., Kumar, S., and Sahoo, L. (2018). Tailoring Microalgae for Efficient Biofuel Production. Front. Mar. Sci. 5, 382. doi:10.3389/fmars.2018.00382
Shen, P.-L., Wang, H.-T., Pan, Y.-F., Meng, Y.-Y., Wu, P.-C., and Xue, S. (2016). Identification of Characteristic Fatty Acids to Quantify Triacylglycerols in Microalgae. Front. Plant Sci. 7, 162. doi:10.3389/fpls.2016.00162
Show, P., Tang, M., Nagarajan, D., Ling, T., Ooi, C.-W., and Chang, J.-S. (2017). A Holistic Approach to Managing Microalgae for Biofuel Applications. Ijms 18 (1), 215. doi:10.3390/ijms18010215
Siaut, M., Cuiné, S., Cagnon, C., Fessler, B., Nguyen, M., Carrier, P., et al. (2011). Oil Accumulation in the Model Green Alga Chlamydomonas Reinhardtii: Characterization, Variability between Common Laboratory Strains and Relationship with Starch Reserves. BMC Biotechnol. 11, 7. doi:10.1186/1472-6750-11-7
Singh, B., Guldhe, A., Singh, P., Singh, A., Rawat, I., and Bux, F. (2015). “Sustainable Production of Biofuels from Microalgae Using a Biorefinary Approach,” in Applied Environmental Biotechnology: Present Scenario and Future Trends. Editor G. Kaushik (New Delhi: Springer), 115–128. doi:10.1007/978-81-322-2123-4_8
Song, M., Pei, H., Hu, W., and Ma, G. (2013). Evaluation of the Potential of 10 Microalgal Strains for Biodiesel Production. Bioresour. Technol. 141, 245–251. doi:10.1016/j.biortech.2013.02.024
Suastes-Rivas, J. K., Hernández-Altamirano, R., Mena-Cervantes, V. Y., Barrios Gómez, E. J., and Chairez, I. (2020). Biodiesel Production, through Intensification and Profitable Distribution of Fatty Acid Methyl Esters by a Microalgae-Yeast Co-culture, Isolated from Wastewater as a Function of the Nutrients' Composition of the Culture Media. Fuel 280, 118633. doi:10.1016/j.fuel.2020.118633
Tabatabaei, M., Tohidfar, M., Jouzani, G. S., Safarnejad, M., and Pazouki, M. (2011). Biodiesel Production from Genetically Engineered Microalgae: Future of Bioenergy in Iran. Renew. Sustain. Energy Rev. 15 (4), 1918–1927. doi:10.1016/j.rser.2010.12.004
Talebi, A. F., Mohtashami, S. K., Tabatabaei, M., Tohidfar, M., Bagheri, A., Zeinalabedini, M., et al. (2013). Fatty Acids Profiling: a Selective Criterion for Screening Microalgae Strains for Biodiesel Production. Algal Res. 2 (3), 258–267. doi:10.1016/j.algal.2013.04.003
Tsuchikane, Y., Hamaji, T., Ota, K., and Kato, S. (2018). Establishment of a Clonal Culture of Unicellular Conjugating Algae. JoVE 137, 57761. doi:10.3791/57761
Um, B.-H., and Kim, Y.-S. (2009). Review: A Chance for Korea to Advance Algal-Biodiesel Technology. J. Industrial Eng. Chem. 15 (1), 1–7. doi:10.1016/j.jiec.2008.08.002
Vello, V., Phang, S.-M., Chu, W.-L., Abdul Majid, N., Lim, P.-E., and Loh, S.-K. (2014). Lipid Productivity and Fatty Acid Composition-Guided Selection of Chlorella Strains Isolated from Malaysia for Biodiesel Production. J. Appl. Phycol. 26 (3), 1399–1413. doi:10.1007/s10811-013-0160-y
Walne, P. R. (1970). “Studies On the Food Value Of Nineteen Genera Of Algae To Juvenile Bivalves Of the Genera Ostrea, Crassostrea, Mercenaria and Mytilus,” in Fishery Investigations, Series 2 (London: H. M. Stationery Office), 26. No. 5.
Wang, W., Li, F., and Li, Y. (2020). Effect of Biodiesel Ester Structure Optimization on Low Temperature Performance and Oxidation Stability. J. Mater. Res. Technol. 9 (3), 2727–2736. doi:10.1016/j.jmrt.2020.01.005
Wang, X.-W., Liang, J.-R., Luo, C.-S., Chen, C.-P., and Gao, Y.-H. (2014). Biomass, Total Lipid Production, and Fatty Acid Composition of the Marine Diatom Chaetoceros Muelleri in Response to Different CO2 Levels. Bioresour. Technol. 161, 124–130. doi:10.1016/j.biortech.2014.03.012
Yahya, L., Harun, R., and Abdullah, L. C. (2020). Screening of Native Microalgae Species for Carbon Fixation at the Vicinity of Malaysian Coal-Fired Power Plant. Sci. Rep. 10, 22355. doi:10.1038/s41598-020-79316-9
Yong, Y. S., Yong, W. T. L., Ng, S. E., Anton, A., and Yassir, S. (2015). Chemical Composition of Farmed and Micropropagated Kappaphycus Alvarezii (Rhodophyta, Gigartinales), a Commercially Important Seaweed in Malaysia. J. Appl. Phycol. 27 (3), 1271–1275. doi:10.1007/s10811-014-0398-z
Yoo, C., Jun, S.-Y., Lee, J.-Y., Ahn, C.-Y., and Oh, H.-M. (2010). Selection of Microalgae for Lipid Production under High Levels Carbon Dioxide. Bioresour. Technol. 101 (1Suppl. ment), S71–S74. doi:10.1016/j.biortech.2009.03.030
Zhang, H., Bhattacharya, D., and Lin, S. (2005). Phylogeny of Dinoflagellates Based on Mitochondrial Cytochrome B and Nuclear Small Subunit Rdna Sequence Comparisons1. J. Phycol. 41 (2), 411–420. doi:10.1111/j.1529-8817.2005.04168.x
Zhao, P., Yu, X., Li, J., Tang, X., and Huang, Z. (2014). Enhancing Lipid Productivity by Co-cultivation of Chlorella Sp. U4341 and Monoraphidium Sp. FXY-10. J. Biosci. Bioeng. 118 (1), 72–77. doi:10.1016/j.jbiosc.2013.12.014
Keywords: biodiesel feedstock, biomass productivity, freshwater algae, marine algae, mass cultivation
Citation: Andrew AR, Yong WTL, Misson M, Anton A and Chin GJWL (2022) Selection of Tropical Microalgae Species for Mass Production Based on Lipid and Fatty Acid Profiles. Front. Energy Res. 10:912904. doi: 10.3389/fenrg.2022.912904
Received: 05 April 2022; Accepted: 03 June 2022;
Published: 28 June 2022.
Edited by:
Marcin Debowski, University of Warmia and Mazury in Olsztyn, PolandReviewed by:
Tonmoy Ghosh, Indian Institute of Technology Indore, IndiaCopyright © 2022 Andrew, Yong, Misson, Anton and Chin. This is an open-access article distributed under the terms of the Creative Commons Attribution License (CC BY). The use, distribution or reproduction in other forums is permitted, provided the original author(s) and the copyright owner(s) are credited and that the original publication in this journal is cited, in accordance with accepted academic practice. No use, distribution or reproduction is permitted which does not comply with these terms.
*Correspondence: Grace Joy Wei Lie Chin, Z3JhY2Vqb3lAdW1zLmVkdS5teQ==
Disclaimer: All claims expressed in this article are solely those of the authors and do not necessarily represent those of their affiliated organizations, or those of the publisher, the editors and the reviewers. Any product that may be evaluated in this article or claim that may be made by its manufacturer is not guaranteed or endorsed by the publisher.
Research integrity at Frontiers
Learn more about the work of our research integrity team to safeguard the quality of each article we publish.