- 1School of Energy and Power Engineering, Jiangsu University, Jiangsu, China
- 2School of Advanced Technologies in Medicine (SATiM), Tehran University of Medical Science (TUMS), Tehran, Iran
- 3Department of Environmental Engineering, School of Architecture and Civil Engineering, Chengdu University, Chengdu, China
- 4Botany and Microbiology Department, Faculty of Science, Tanta University, Tanta, Egypt
Microalgae are known as the most efficient biological sequesters of carbon dioxide (CO2). Recently, they have been exploited to enhance air quality by decreasing CO2 levels and increasing oxygen (O2) concentrations. However, in public places, there are sources of toxic chemicals such as tobacco smoke that may affect the growth of microalgae. For the first time, the current study explores the influence of tobacco smoke on the cell growth, biodiesel characteristics, and biochemical composition of two strains of model microalgae. Chlamydomonas strains were treated with tobacco smoke in a sealed box for 7 days, wherein every 1 h, one cigarette was burnt. Upon exposure of microalgae to tobacco smoke, the specific growth rate (μmax) was unaffected in CHL-2220 and remained around 0.500 days−1, whereas in CHL-2221, it decreased notably from 0.445 days−1 to 0.376 days−1. In the CHL-2221, the lipid level considerably reduced from 15.55 %DW to 13.37 %DW upon exposure to tobacco smoke. In both strains, palmitic acid was the main constituent of saturated fatty acids (SFAs) that displayed significant (p < 0.05) decreases in response to tobacco smoke exposure. In CHL-2221, oleic acid (C18:1) presented a substantial increase from 7.64 to 17.09% in response to tobacco smoke. Moreover, exposure of CHL-2220 to tobacco smoke decreased the cetane number (CN) from 30.34 to 25.28, while in CHL-2221, it increased from 26.07 to 29.73 upon treatment. Both strains demonstrated low-quality biodiesel to be used as feedstock. Conversely, their fatty acid profile revealed their promising use as nutrient food.
Introduction
According to the World Health Organization (WHO), being exposed to low-quality air and polluted air are serious environmental hazards to people (Health Organization, 2016). Recently, indoor air pollution has become more prominent as people pass more than 80% of their time in places that are carefully sealed to minimize energy losses of heating and cooling systems (González-Martín et al., 2021). In indoor air, particulate matter (smoke, biomass, and dust), bioaerosols (microorganisms, spores, and molds), and gaseous chemicals [carbon monoxide (CO), ozone (O3), nitrogen dioxide (NO2), sulfur dioxide (SO2), volatile organic compounds, and formaldehyde] are the most common forms of indoor pollutants that are resulted from building and construction waste, human activities, and penetration of unfiltered outdoor air (Deng and Deng, 2018). Moreover, studies have revealed that low-quality indoor air as a result of increased CO2 levels is the main factor in health problems globally, which leads to substantial money losses (Azuma et al., 2018; Du et al., 2020). Microalgae are efficient photosynthetic microorganisms that can be utilized for air purification to generate O2 and decrease CO2, toxic elements including SOx and NOx (Cheng et al., 2019). Therefore, microalgal air purifiers may be a possible solution for reducing indoor pollution and CO2 levels in public places, including schools, offices, or malls (Megahed and Ghoneim, 2020). For this reason, the utilization of microalgae as a natural air purifier and (CO2 sequester and O2 producer) has gained attention worldwide (Durán et al., 2018). Throughout the microalgae growth, CO2 is transformed to O2, and biomass can be utilized to generate different biofuels (Enamala et al., 2018). Also, microalgae are extraordinary organisms that are able to store lipids ranging from 30 to 70% of the cellular dry weight (DW) (Bellou et al., 2014). The lipid extract from microalgae can be exploited for biodiesel generation over a chemical transformation method known as transesterification (Gerotto et al., 2020; Wang et al., 2021). In comparison to other biodiesel feedstocks, microalgae are more attractive as in their cultivation, there is no competition with human food, and are able to cultivate on non-arable and marginal land (Veillette et al., 2018). Furthermore, it is recognized that microalgae can store high-value lipid subunits, polyunsaturated fatty acids (PUFAs), which are key constituents playing an important part in the health and development of both humans and animals (Promoters et al., 2014).
Flue gas mainly consists of CO2, NO, NO2, SO3, and CO, heavy metals (Van Den Hende et al., 2012). The application of microalgae for sequestration of CO2 from flue gas emitted from power plants and cement factories has been reported (Zhao et al., 2021). For example, Tetraselmis suecica was cultivated in an industrial-scale airlift PBR under an unfiltered coal-fired power plant containing N2 (62%), O2 (5%), CO2 (11%), H2O (22%), SO2 (220 ppm), NOx (150 ppm), and dust (∼25 ppm), while promising organic biomass productivity (178.9 ± 30 mg L−1 day−1) and CO2 sequestration (89.15 ± 20 mg “C” L−1) were obtained (Moheimani, 2016). Also, mixed consortia of microalgae were grown under 10, 30, and 50% flue gas from a 4-MW (megawatt) coal-fired boiler; commonly, the mixtures demonstrated a decrease in the lipid content as the flue gas level increased, and treatment by 10% flue gas presented better results comparatively (Aslam et al., 2018a). On the other hand, several patents have been approved regarding the applicability of microalgae as indoor air purifiers (Lu et al., 2019). However, the impacts of indoor air pollutants such as tobacco smoke on their performance, such as physiological behavior and biodiesel parameters, have never been reported. Notwithstanding the existence of specific smoking rooms in some public areas, leakage of tobacco smoke has been reported (Pion and Givel, 2004). Thus, if microalgal air purifiers in public places that even have a smoking room, such as some airports, hotels, and train stations, are used, it is essential to assess the effect of tobacco smokes on microalgae. As described earlier, in indoor places, the existence of tobacco smoke and the accumulation of its compounds into the microalgae medium can inhibit microalgae growth. In fact, tobacco smoke contains several toxicologically well-known chemicals such as quinoline, 1,1-dimethylhydrazine, acrylonitrile, benzene, acetaldehyde, and 2-nitropropane (Talhout et al., 2011), and there is no study investigating the effect of these compounds individually or whole tobacco smoke on microalgae. Among microalgae species, Chlamydomonas sp. is deliberated as an outstanding model microorganism that is studied due to its various potential uses (Scranton et al., 2015). Hence, this study aimed to investigate the influence of tobacco smoke on biochemical compositions, growth, and biodiesel parameters of Chlamydomonas sp., which can provide valuable insights for the research community and inventors into the possible effect of tobacco smoke on the performance and applicability of produced biomass.
Materials and Methods
Experimental Design and Culture Conditions
Two local Chlamydomonas strains were obtained from the Freshwater Algae Culture Collection at the Institute of Hydrobiology (FACHB) with the record ID of FACHB-2221 and FACHB-2220 and in the current experiment denoted as CHL-2221 and CHL-2220, respectively. The stock cultures were grown in the fresh medium of Tris–acetate–phosphate (TAP) and grown at ambient temperature (25 ± 1.5°C) under light illumination of 50 µmol photons m−2 s−1 prior to the treatment. The 20% inoculum size culture was prepared for the experiment by inoculating 100 ml of stock culture with an optical density (OD) of 0.2 at 750 ʎ (OD750 = 0.2) into 400 ml fresh TAP medium in a 1-L conical flask. The inoculated cultures were grown at 25 ± 1.5°C and illuminated in a 12:12-h light–dark cycle with white fluorescent lamps with 50 µmol photons m−2 s−1 light intensity. An air pump fixed in a container was used to provide the aeration. To examine the influence of tobacco smoke on microalgae, every 1 h, one cigarette was burnt and kept in the container to provide the smoke to microalgae cultures, while for the control, cultures only aerated with atmospheric air. The schematic of the experimental rig used in the study is presented in Supplementary Figure S1. The maximum specific growth rate was calculated throughout the exponential growth phase based on the natural logarithm of optical density (OD750) against time (day) following Eq. 1 (Barati et al., 2018):
where De is OD750 at te, Di is OD750 at ti, and te and ti are times in the exponential phase.
Analysis of Protein, Lipid, Carbohydrate, and Pigment Contents
Total carbohydrate concentration was measured based on the widely accepted protocol offered by Borowitzka and Moheimani (2013) (Borowitzka and Moheimani, 2013) with minor modifications. In brief, 50 ml of each replicate was centrifuged, and the pallet was ground in HCl (2M) with a hand homogenizer. The ground samples were sonicated and incubated for 1 h at 80°C in an ultrasonicator. Next, the samples were centrifuged, and aliquots of samples were taken into glass tubes, followed by phenol, water, and gentle addition of H2SO4 (95.5% v/v). Then, the samples were incubated for 30 min, and the OD was determined at 485 nm (OD485). Finally, the concentration of carbohydrates was calculated from the standard curve by considering glucose as a reference sugar. Lipids were recovered by means of methanol/chloroform/water (2:1:0.8 v/v) and calculated via the gravimetric technique by Bligh and Dyer (Bligh and Dyer, 1959). The solution in the glass vial was air-dried with nitrogen gas, and then the dried extract was retained for 24 h in a desiccator before determining the weight. The difference in weights of glass vials was considered as the lipid weight. Proteins were recovered with NaOH 0.5 N at 80°C for 30 min, and the protein concentration was calculated via the dye-binding technique by Bradford using the standard curve produced by bovine serum albumin as standard (Borowitzka and Moheimani, 2013). Additionally, carotenoid and chlorophyll extractions were done by filtration of 20 ml culture on the glass-fiber filter (Whatman GF/C, 0.45 μM), and subsequent homogenization in 10 ml of absolute methanol. After overnight incubation, the samples were centrifuged for 5 min at 1,409 × g to attain the clear supernatant prior to spectrophotometric quantification. Chlorophyll concentrations (mg/L) were calculated by analyzing the cell extracts’ absorbance at 645 and 665 nm using a UV–vis spectrophotometer following Eq. 2 (Lichtenthaler, 1987):
Likewise, carotenoids (mg/L) were quantified from the same solution by determining the absorbance at 470 nm following Eq. 3 (Lichtenthaler, 1987):
Analysis of Fatty Acid Profile
Lipid transesterification was performed by mixing 1 ml of 1% H2SO4–MeOH with the lipid extract and incubating for 60 min at 90°C by a test tube heater. Subsequently, the methylated fatty acids (FAMEs) were recovered by hexane, and then hexane was evaporated using the stream of nitrogen gas. Then, the samples were preserved at −20°C. The recovered FAMEs were analyzed via gas chromatography–mass spectrometry (GC/MS, Thermo Fisher Scientific, United States) equipped with a non-polar DB 35 column where helium gas was used as the carrier. From each sample, 1 µl of FAME was injected to the GC/MS controlled under systematic temperature settings of the initial oven temperature set at 40°C for 5 min, then elevated at a rate of 4°C min−1 to 260°C, and held for 1 min.
Analysis of Biodiesel Parameters
To examine the biomass quality as a feedstock for biodiesel production, the physicochemical characteristics of FAMEs were calculated according to widely accepted formulas. The cetane number (CN), percentage of unsaturation degree (DU, %), long-chain saturation factor (LCSF, wt%), iodine value (IV, g I2/100 g oil), cold filter plugging point (CFPP,°C), and saponification value (SV, mg KOH g−1) were calculated according to Francisco et al. (2010) and Ashour et al. (2019), as presented in Eqs. 4–9:
where PUFAs and MUFAs signify the proportion of polyunsaturated and monounsaturated fatty acids.
where N% signifies the ratio of each individual fatty acid, M signifies the molecular weight of each fatty acid, and D denotes the quantity of carbon–carbon double bonds.
where C24:0, C22:0, C20:0, C18:0, and C16:0 signify the weight ratio of each fatty acid. In addition, the higher heating value (HHV) is estimated via Equation 10 (Demirbas, 2016):
Data Analysis
The influence of tobacco smoke on the specific growth rate, lipids, proteins, fatty acids, and carbohydrates was evaluated using one-way ANOVA. All calculations were done in SPSS 16.0 (SPSS Inc, United States), and the variances were assumed significant when p < 0.05.
Result and Discussion
Influence of Tobacco Smoke on Microalgae Growth
Growth was analyzed by measuring the OD of the cultures cultivated under ambient atmospheric air and tobacco smoke for the duration of 7 days. The growth patterns were varied for the two studied microalgal strains upon exposure to tobacco smoke (Figure 1). It can be seen that by the increase in the exposure duration, the differences were intensified in CHL-2221, and by the end of the experiment, the cell concentration of smoke-treated CHL-2221 compared to the control was considerably lower. In CHL-2220, lower growth was observed till day 3; however, it was able to tolerate the tobacco smoke and recover its proliferation ability by the end of the experiment, which is possibly owing to the presence and activation of tolerance mechanisms under tobacco smoke, although their activation required time. The biomass productivity and specific growth rate (μmax) of the investigated strains are shown in Figure 1. In ambient aeration, μmax in CHL-2221 and CHL-2220 was, 0.445 days−1 and 0.506 days−1, individually, displaying a considerably faster growth in CHL-2220. The μmax was not influenced in CHL-2220 (p > 0.05) by exposure to tobacco smoke, and it was almost 0.500 days−1, whereas in CHL-2221, μmax reduced meaningfully (p < 0.05) from 0.445 days−1 to 0.376 days−1. The difference in growth response of studied strains is due to their capability to tolerate various contaminations in tobacco smoke. For example, heavy metals can cause this growth inhibition. It was shown that inorganic chemicals As, Co, Cd, Cu, Cr, Hg, Ni, Mn, Pb, Se, Sb, Sn, Zn, and V were added to the microalgal growth medium of Nannochloropsis salina, which resulted in significant suppression of growth (Hess et al., 2017). Additionally, the presence of heavy metals in tobacco smoke can be considered a key growth inhibitor. Quinoline, acrylonitrile, and dimethylhydrazine are the three main components of tobacco smoke, and they are probably the main factors affecting microalgae growth. Quinoline is known as a common N-containing six-membered ring compound that is present in algal bio-oil and in organic chemical wastewater [31]. It is reported that it is biodegradable and organisms can use it as a carbon source [32]. Acrylonitrile is a resin derivative and is widely used in various industrial manufacturing fields [33]; its biodegradation by bacteria is reported [34]. The toxicity effect of 1,1-dimethylhydrazine can be mitigated by antioxidant molecules in microalgae [35]. The availability of mechanisms for the degradation of these compounds in bacteria suggests that our studied microalgal strains may comprise those mechanisms. Therefore, future research is required to confirm it.
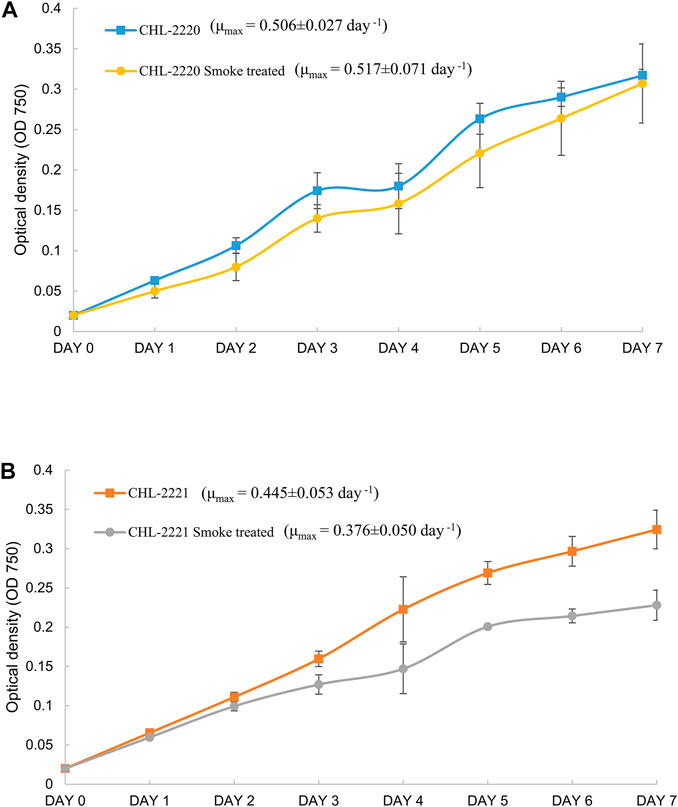
FIGURE 1. Comparison of growth of CHL-2220 (A) and CHL-2221 (B) grown under tobacco exposure and ambient conditions. Data signify the mean value of triplicates and error bars are standard deviations.
Influence of Tobacco Smoke Exposure on Biochemical and Pigment Composition
Overall, the lipid concentration of the studied strains was reduced in response to tobacco smoke. CHL-2220 accumulated lipid by 15.16 %DW under control condition, while upon smoke exposure, it decreased to 13.82 %DW (p > 0.05) (Figure 2A). Likewise, a similar pattern was seen in CHL-2221, when its lipid level of 15.55 %DW under ambient conditions (p > 0.05) reduced considerably to 13.37 %DW, which indicates lipid biosynthesis inhibition upon smoke exposure in both strains. This is not in agreement with some studies, as microalgae usually accumulate lipid bodies under stress conditions (Wang et al., 2009). However, the reduction of the lipid content was similarly observed in microalgae consortia when the culture was exposed to unfiltered flue gas, and it was suggested that the suppression of fatty acid synthesis was due to the presence of impurities and acidifications (Aslam et al., 2018a). In addition, the increase in carbohydrates in microalgae often occurs during environmental stresses. In addition, microalgae are shown to accumulate starch as the leading energy and carbon storage molecule (Zhu et al., 2014). The influence of smoke on levels of carbohydrate varied among the studied strains (Figure 2B). In CHL-2221, the carbohydrate level amplified meaningfully (p < 0.05) when it was grown under tobacco smoke conditions, while in CHL-2220, it did not vary significantly. In CHL-2221, by considering its growth inhibition and carbohydrate level increases, it could be proposed that this strain stored carbohydrates as a stress response to tobacco smoke because carbohydrate metabolism regulation is vital in keeping the balance between proliferation and photosynthesis. Moreover, starch is the central storage compound in Chlamydomonas sp. (Puzanskiy et al., 2018), which is produced predominantly and more compared to lipids in moderate stress conditions, and also it is altered quicker subsequent to recovering to the ambient conditions (Siaut et al., 2011). Conversely, a different pattern was seen in the protein content. In CHL-2221 and CHL-2220, protein content increased from 35.48% and 30.91% to 36.55% and 34.56%, individually upon exposure to tobacco smoke (Figure 2C). A noteworthy shift in protein level was detected in CHL-2220, whereas it was not significant in CHL-2221. In fact, this trend was predictable as CHL-2220 presented tolerance to tobacco smoke exposure; thus, it might be due to the synthesis of proteins that confer tolerance. For instance, heat shock proteins (HSPs) are the commonly known proteins that are generally overexpressed in stress conditions; nevertheless, more advanced molecular analyses are essential to recognize the proteins that are differentially expressed (Barati et al., 2019). Alterations induced by different stressors are primarily noticeable in changes of photosynthetic pigments compared to the evidence of any variations in the cell growth (Haire et al., 2018). In CHL-2220, the carotenoid level was not influenced (p > 0.05), and it marginally fluctuated between 0.32 and 0.34 (Figure 2D), suggesting that this strain did not experience significant stress by the smoke exposure, while considerable changes (p > 0.05) were seen in CHL-2221 when the Car/Chl ratio was higher (0.43) upon treatment than under normal conditions (0.28). The elevated Car/Chl ratio proposes the occurrence of stress upon tobacco smoke. The increase in carotenoid levels is confirmed in Chlamydomonas sp. as a response to diverse stressors (Almarashi et al., 2020), including exposure to heavy metals stress (Nowicka et al., 2016). This proposes the conceivable influence of toxic chemicals present in tobacco smoke.
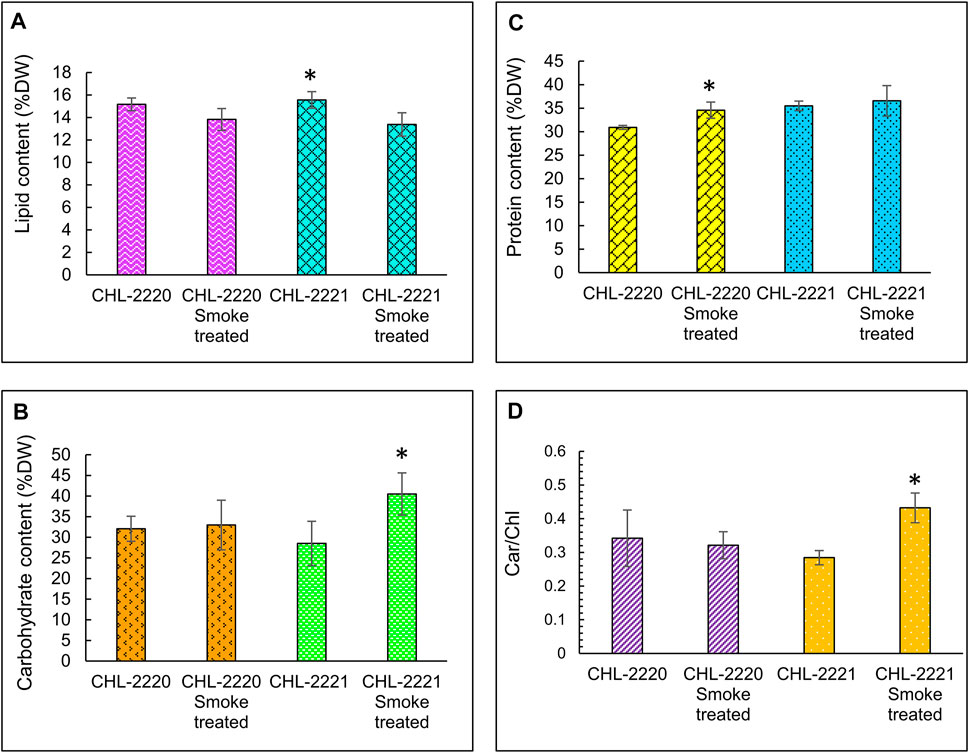
FIGURE 2. Biochemical and pigment compositions of CHL-2221 and CHL-2220 upon 7-day tobacco smoke exposure and ambient air with Percentage of lipid (A), carbohydrate (B), protein (C) and Chl/Caro ratio (D) of CHL-2221 and CHL-2220 upon 7-day tobacco smoke exposure and ambient air.
Influence of Tobacco Smoke Exposure on Composition of Fatty Acids
The fatty acid composition of CHL-2221 and CHL-2220 grown under tobacco smoke exposure and ambient conditions is displayed in Table 1. The ratio of saturated fatty acids (SFAs) in both strains significantly varied. The SFA level of CHL-2220 upon exposure to tobacco smoke declined significantly (p < 0.05) from 29.15 to 24.14%, whereas no substantial variations were detected in the SFA level of CHL-2221, and it remained at about 20%. Among SFAs, palmitic acid was the main constituent and displayed significant (p < 0.05) decreases in response to tobacco smoke exposure. Palmitic acid (C16) is the most frequently found constituent of the membrane cell wall and is also one of the main SFAs in microalgae (Osorio et al., 2019). Also, its substantial alterations in response to diverse stressors, including salinity stress conditions, have been previously reported (Yang et al., 2018), indicating its probable participation in acclimation under environmental stress conditions. Mutually, both studied strains presented reduced palmitic acid in response to tobacco smoke as it declined from 10.68 to 7.31% in CHL-2221 and from 20.18 to 11.22% in CHL-2220. Both CHL-2220 and CHL-2221 MUFAs’ content demonstrated a significant increase in response to the tobacco smoke by demonstrating an increase in MUFA from 11.59 to 15.97% and from 13.10 to 20.73%, respectively. Palmitelaidic acid (C16:1) and oleic acid (C18:1) were the dominant MUFAs in both strains. In CHL-2220, oleic acid (C18:1) content has no significant (p > 0.05) alterations, while its content in CHL-2221 significantly (p < 0.05) increased from 7.64 to 17.09% in response to tobacco smoke. Oleic acid is the major constituent of triacylglycerols (TAGs); therefore, the increase in its content can be considered as TAG accumulation (de Jaeger et al., 2017). Moreover, according to the previous study on green microalgae (Srivastava et al., 2017), the acclimation process in the current study could be considered the CHL-2221 response to tobacco smoke.
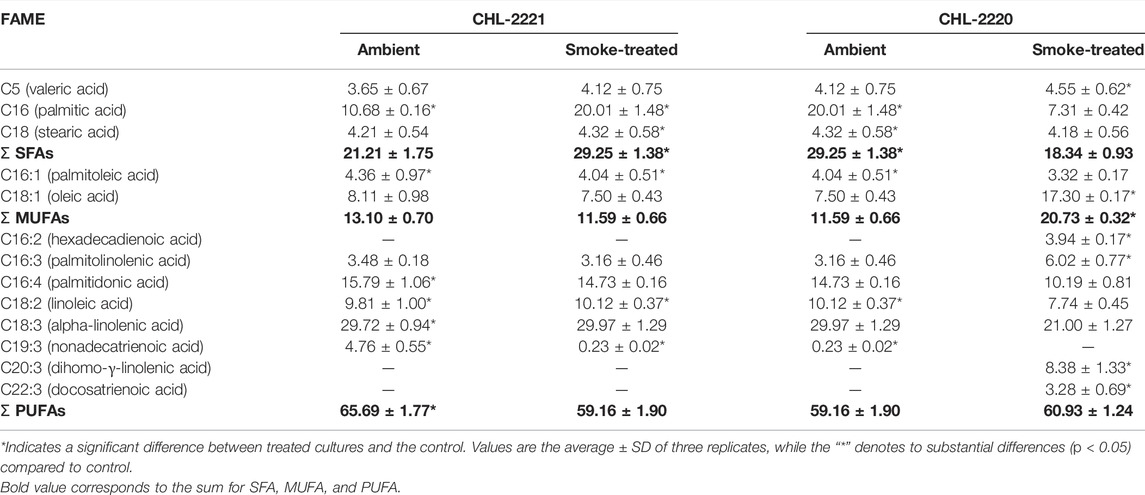
TABLE 1. FAME composition of CHL-2221 and CHL-2220 under tobacco smoke exposure and ambient air conditions.
The main FAME components obtained from both tobacco smoke-treated strains and control strains in this experiment were the PUFAs. Its content has no significant alteration in control and tobacco smoke-treated CHL-2220 strains and was around 59%, while a significant decline was observed in CHL-2221 treated by the tobacco smoke (declined from 65.69 to 60.93%). Alpha-linolenic acid (C18:3) was the main PUFA in mutually both treated and control strains, which is a common dominant unsaturated fatty acid in many microalgae species (Moon et al., 2013). The CHL-2220 control strain and CHL-2221 control strain have the alpha-linolenic acid (C18:3) content of 29.97 and 29.72%, respectively. However, treating the CHL-2221 strain with tobacco smoke dramatically decreased the alpha-linolenic acid content (from 29.72 to 21%). On the other hand, the alteration of the alpha-linolenic acid content of CHL-2220 strains treated with tobacco smoke remained unchanged, while phosphorous (Qari and Oves, 2020) and nitrogen (Sun et al., 2018) stress in the Chlamydomonas strains have caused alpha-linolenic acid accumulation. Meanwhile, hexadecadienoic acid (C16:2) was another evident PUFAs in this study. It was not detected in both control strains under ambient conditions, while the exposure to tobacco smoke led to an increase of C16:2 in CHL-2221 and CHL-2220 to 3.81 and 3.88%, respectively. Dihomo-γ-linolenic acid (C20:3) has demonstrated the same pattern as hexadecadienoic acid (C16:2). Although C20:3 was not found in the studied strains grown in ambient conditions, exposure to tobacco smoke led to its accumulation by 3.42 and 3.94% in the CHL-2220 and CHL-2221, respectively. These results suggest that dihomo-γ-linolenic acid might have the acclimatization role in response to the tobacco smoke ambient. Moreover, the synthesis of omega-3 and omega-6 fatty acids has been provoked in both strains in response to tobacco smoke. This can be considered as a positive impact. Due to the essential role of fatty acids such as omega-3 and omega-6 in human well-being, additional analysis is necessary to identify the active compounds triggering their synthesis. However, molecular studies and toxicological screening should be considered prior to any decision to use them as a food supplement.
Influence of Tobacco Smoke Exposure on Characteristics of Biodiesel
In order to study the impact of tobacco smoke exposure on biodiesel features, the key biofuel parameters such as SV, IV, CN, CFFP, DU, HHV, and LCSF were investigated in the selected strains (Table 2). Also, we compared these parameters with other Chlamydomonas sp. grown under nitrogen-rich (N+) conditions that previously showed a promising result (Maneechote et al., 2021). The DU indicates the total mass ratio of MUFAs and PUFAs; therefore, the higher the DU, the higher the unsaturation of fatty acids. Although the treated and control samples of CHL-2221 showed no significant alteration in the DU value, the tobacco smoke-treated CHL-2220 displayed a slight increase in the DU value (from 129.91 wt% to 135.74 wt%). However, a significantly lower DU value of 58.20 was observed in other Chlamydomonas sp. grown under enriched N conditions. CN is another critical biofuel property that contributes to the ignition quality of the biofuels. The CN value strictly depends on the DU value. Surprisingly, by CHL-2220 exposure to tobacco smoke, the CN value decreased from 30.34 to 25.28, while CHL-2221 treatment with tobacco smoke increased the CN value from 26.07 to 29.73. However, a relatively higher CN value of 57.92 was observed in Chlamydomonas sp. grown under elevated nitrogen concentration. It shows the relatively lower quality of CN value of the studied strains in both ambient and treatment conditions of the current study than that of other Chlamydomonas sp.
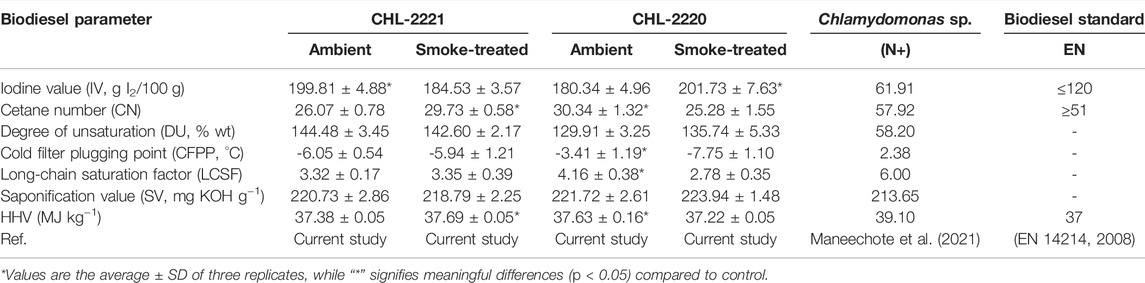
TABLE 2. Biodiesel parameters of CHL-2221 and CHL-2220 in response to ambient air and tobacco smoke exposure.
Another key factor in investigating fatty acid unsaturation is the iodine value (IV), which measured the grams of iodine reacting with 100 g of the FAME sample (Ramos et al., 2009). The IV represents the double bonds available in the unsaturated fatty acid chain structure (EN 14214, 2008). The tobacco smoke exposure increased the IV of the CHL-2220 from 180.34 g I2 100 g−1 to 201.73 g I2 100 g−1, while declining the IV of CHL-2221 from 199.81 g I2 100 g−1 to 184.53 I2 100 g−1. By comparison, Chlamydomonas sp. was reported to have almost three times lower IV value (61.91 g I2 100 g−1), which was in the acceptable range suggested by EN. As it has been determined by the European Standard organization [48], biodiesel with higher IV than 120 g I2 100 g−1 will polymerize and form glycerides. This phenomenon at elevated temperatures leads to deposit buildup or increase in lubricating oil corrosivity (Shaltout et al., 2019). The milligrams of potassium hydroxide (KOH) required to saponify 1 g of FAMEs is described as the saponification value (SV). The SV has an opposite correlation with the chain length of fatty acids. The SV of tobacco smoke-treated CHL-2220 culture was not altered significantly (slightly increased from 221 mg KOH g−1 to 223 mg KOH g−1) in comparison to the control. Similarly, the tobacco smoke-treated CHL-2221 strain SV alteration was not significant compared to the control strain (declined from 220.73 mg KOH g−1 mg KOH g−1 to 218.79 mg KOH g−1). The SV value of Chlamydomonas sp. was 213 mg KOH g−1, which was comparatively lower. The high SV of both strains means a low yield of biodiesel, while extra methanol is required during the biodiesel production process and increases with the production of glycerol (Bart et al., 2010).
Another key factor that should be considered is cold filter plugging point (CFPP) which indicates the minimum temperature that the biodiesel can run an engine without any problem (Vidyashankar et al., 2015). The increase in the SFA concentration increases the CFPP value. In the current study, different CFPP responses were observed from the Chlamydomonas strains exposed to tobacco smoke. The CFPP of tobacco smoke-treated CHL-2220 dropped considerably from −3.41°C to −7.75°C, while this value has increased in the tobacco smoke-treated CHL-2221 strain from −6.05°C to −5.94°C. Therefore, it can be suggested that tobacco smoke has positive impacts on CHL-2220 and enhance the properties of biodiesel extracted from tobacco smoke-treated CHL-2220, while the Chlamydomonas sp. presented a poor CFPP value of 2.23°C, which limits the applicability of the biodiesel to warm climates. This is one of the drawbacks of biodiesel with a high CN value that usually exhibits poor CFFP, due to the high SFA level. LCSF is another key property that describes CN and IV. The higher LCSF represents more favorable physicochemical features of the generated biodiesel. In this study, tobacco smoke exposure led to the decline of the LCSF value in both strains. In CHL-2220, it caused a 33% decline, while the LCSF value of CHL-2221 was not significantly altered. Higher heating value (HHV) is the volume of heat energy produced through the combustion of 1 g of fuel and reports through units of energy per unit mass or volume of substance (Demirbas, 2016). It has been suggested that the fuel with higher HHV is more efficient for small-size engines (Mondal et al., 2019). The HHV values of both strains in the current study were 37, which were perfectly comparable with the suggested acceptable range defined by EN (Brithish Standard EN 14214, 2008). European standards (EN14214) and Australian standards have indicated the optimum CN value of 51 (Kemp and Kemp, 2003; Brithish Standard EN 14214, 2008), while the CN values of the studied strain did not meet it due to the high DU value. Therefore, it can be suggested that these tobacco smoke-treated strains can be used for biodiesel production if the DU could be decreased. Therefore, a proper balance of MUFAs, including oleic acid, and lesser PUFAs and SFAs, would serve this purpose. Blending microalga biomass with other feedstock is a suggested solution to overcome this matter and enhance the biodiesel quality (Abomohra et al., 2020).
Practical Implications, Limitation, and Future Works
This study contributes to developing more sustainable biological air purifiers. The main implication of microalgal air purifiers is that the CO2 of indoor environments such as offices, shopping malls, train stations, and airports can be reduced and instead O2 produced. This is of great significance as the indoor places are usually sealed to control temperature, while CO2 produced by crowd is accumulated and decreases the air quality for people. In microalgal air purifiers, O2 is produced as the culture grows, and CO2 is transformed into the cell building block (e.g., lipids and carbohydrates). Therefore, a circular economy platform can be achieved by converting biomass to high-value products. For example, biodiesel can be generated from microalgae as a more environmentally friendly method to fuel production, or pigments can be recovered to be used as natural colorants. Several patents were published describing reactor design for air purification purposes in recent years, but the potential inhibitory of smoke was not considered. Thus, this study provides valuable information on the possibility of reactor failure due to the accumulation of toxins of tobacco smoke over time and consequently warns the researchers to consider the influence of air pollutants on microalgae.
Tobacco smoke is composed of various compounds that are released in the form of gas during aeration; studying the composition of inlet and outlet gas can provide important information on the absorbance capability of microalgae. However, this was not studied in this study due to the limitation of experimental tools such as gas sensors. Therefore, we only studied the effect of the entire tobacco smoke composition on the performance of microalgae. Many studies have already discussed the effect of heavy metals, SOX, NOX, and pharmaceutical pollutants on microalgae growth. Nevertheless, the effect of important chemicals such as acrylonitrile and benzene has not been studied so far, suggesting an interesting potential research gap for future studies. In the current study, both strains’ lipid extracts presented poor biodiesel characteristics, whereas their substantial PUFA ratio recommended its applicability for pharmaceuticals, nutrients, and animal feed. Therefore, it is recommended to investigate the toxicity of FAME extracts after smoke exposure. Moreover, the tolerance of microalgae can be enhanced to achieve higher microalgae performance using adaptive laboratory evolution (ALE). ALE is a process of selecting the most suitable mutations to particular stresses or conditions that naturally occur in the cell population (Arora et al., 2020). It was found that it was a successful approach to enhance the tolerance of microalgae to unfiltered flue gas (Aslam et al., 2017) and chemical contaminants such as phenol (Wang et al., 2016). Therefore, it can be considered as a method to enhance the tolerance of microalgae to air pollutants in future studies.
Conclusion
The multiplicity of complex chemical compounds in the smoke of tobacco and information scarcity regarding its impact on the microalgae performance was the main motive of this study. Therefore, the growth and biochemical composition and biodiesel parameters of two Chlamydomonas strains (CHL-2221 and CHL-2220) upon tobacco smoke exposure were studied for the first time. According to the findings of this study, the impact of smoke was a strain-dependent phenomenon, and therefore, strain screening is required prior to selecting the candidate microalgae. CHL-2220 tolerated the introduced tobacco smoke and successfully adapted, demonstrating a strong performance and minor changes of biochemical components, while in CHL-2221, the biochemical composition, pigments, and growth was affected severely. Moreover, the biodiesel characteristics of strains treated with tobacco smoke were altered. The degree of unsaturation and the amount of carbon double-bound were increased in the tobacco smoke-treated CHL-2220 strain in comparison to the control. Furthermore, exposure to tobacco smoke has induced the biosynthesis of PUFA (omega-6 and omega-3). Based on all these findings, this study proposes the application of microalgae as a potential natural air purifier to improve the O2 level of the places where tobacco smoke exists with the perspective of harvesting its by-products and biomass.
Data Availability Statement
The raw data supporting the conclusions of this article will be made available by the authors, without undue reservation.
Author Contributions
BB, FF, AA and SW contributed to the conception and design of this study. BB, CH, LQ and FF developed the method; acquired the data; and wrote the first draft of the manuscript. AA and FF ran the analyses. AA and SW provided feedback on the analysis. AA, BB and CH provided revisions and editing. All authors read and approved the submitted version.
Funding
This work was supported by the Natural Science Foundation of Jiangsu Province, China (20190843), and China Postdoctoral Science Foundation (2019M661742).
Conflict of Interest
The authors declare that the research was conducted in the absence of any commercial or financial relationships that could be construed as a potential conflict of interest.
Publisher’s Note
All claims expressed in this article are solely those of the authors and do not necessarily represent those of their affiliated organizations, or those of the publisher, the editors and the reviewers. Any product that may be evaluated in this article, or claim that may be made by its manufacturer, is not guaranteed or endorsed by the publisher.
Supplementary Material
The Supplementary Material for this article can be found online at: https://www.frontiersin.org/articles/10.3389/fenrg.2022.881758/full#supplementary-material
References
Abomohra, A. E.-F., Elsayed, M., Esakkimuthu, S., El-Sheekh, M., and Hanelt, D. (2020). Potential of Fat, Oil and Grease (FOG) for Biodiesel Production: A Critical Review on the Recent Progress and Future Perspectives. Prog. Energ. Combustion Sci. 81, 100868. doi:10.1016/j.pecs.2020.100868
Almarashi, J. Q. M., El-Zohary, S. E., Ellabban, M. A., and Abomohra, A. E.-F. (2020). Enhancement of Lipid Production and Energy Recovery from the green Microalga Chlorella Vulgaris by Inoculum Pretreatment with Low-Dose Cold Atmospheric Pressure Plasma (CAPP). Energ. Convers. Manag. 204, 112314. doi:10.1016/j.enconman.2019.112314
Arora, N., Yen, H.-W., and Philippidis, G. P. (2020). Harnessing the Power of Mutagenesis and Adaptive Laboratory Evolution for High Lipid Production by Oleaginous Microalgae and Yeasts. Sustainability 12, 5125. doi:10.3390/su12125125
A. Shaltout, N., A. Ghazal, M., G. Shams El-Din, N., E. Ali, A., and M. Beltagy, D. (2019). Optimization and Kinetic Studies of Biodiesel Production from the green Alga Ulva Fasciata Delile. Egypt. J. Aquat. Biolo. Fish. 23, 301–322. doi:10.21608/ejabf.2019.54922
Ashour, M., Elshobary, M. E., El-Shenody, R., Kamil, A.-W., and Abomohra, A. E.-F. (2019). Evaluation of a Native Oleaginous marine Microalga Nannochloropsis Oceanica for Dual Use in Biodiesel Production and Aquaculture Feed. Biomass and Bioenergy 120, 439–447. doi:10.1016/j.biombioe.2018.12.009
Aslam, A., Thomas-Hall, S. R., Mughal, T. A., and Schenk, P. M. (2017). Selection and Adaptation of Microalgae to Growth in 100% Unfiltered Coal-Fired Flue Gas. Bioresour. Technol. 233, 271–283. doi:10.1016/j.biortech.2017.02.111
Aslam, A., Thomas-Hall, S. R., Manzoor, M., Jabeen, F., Iqbal, M., uz Zaman, Q., et al. (2018a). Mixed Microalgae Consortia Growth under Higher Concentration of CO2 from Unfiltered Coal Fired Flue Gas: Fatty Acid Profiling and Biodiesel Production. J. Photochem. Photobiol. B: Biol. 179, 126–133. doi:10.1016/j.jphotobiol.2018.01.003
Azuma, K., Kagi, N., Yanagi, U., and Osawa, H. (2018). Effects of Low-Level Inhalation Exposure to Carbon Dioxide in Indoor Environments: A Short Review on Human Health and Psychomotor Performance. Environ. Int. 121, 51–56. doi:10.1016/j.envint.2018.08.059
Barati, B., Gan, S.-Y., Lim, P.-E., Beardall, J., and Phang, S.-M. (2019). Green Algal Molecular Responses to Temperature Stress. Acta Physiol. Plant 41, 26. doi:10.1007/s11738-019-2813-1
Barati, B., Lim, P.-E., Gan, S.-Y., Poong, S.-W., Phang, S.-M., and Beardall, J. (2018). Effect of Elevated Temperature on the Physiological Responses of marine Chlorella Strains from Different Latitudes. J. Appl. Phycol. 30, 1–13. doi:10.1007/s10811-017-1198-z
Bart, J. C. J., Palmeri, N., and Cavallaro, S. (2010). Emerging New Energy Crops for Biodiesel Production. Biodiesel Sci. Technol. 290, 226–284. doi:10.1533/9781845697761.226
Bellou, S., Baeshen, M. N., Elazzazy, A. M., Aggeli, D., Sayegh, F., and Aggelis, G. (2014). Microalgal Lipids Biochemistry and Biotechnological Perspectives. Biotechnol. Adv. 32, 1476–1493. doi:10.1016/j.biotechadv.2014.10.003
Bligh, E. G., and Dyer, W. J. (1959). A Rapid Method of Total Lipid Extraction and Purification. Can. J. Biochem. Physiol. 37, 911–917. doi:10.1139/o59-099
Borowitzka, M. A., and Moheimani, N. R. (2013). “Algae for Biofuels and Energy,” in Algae for Biofuels and Energy. Editors M. A. Borowitzka, and N. R. Moheimani (Dordrecht: Springer Netherlands). doi:10.1007/978-94-007-5479-9
British Standard EN 14214 (2008). Automotive Fuels — Fatty Acid Methyl Esters (FAME) for Diesel Engines — Requirements and Test Methods. Eur. Comm. Stand. 14214.
Cheng, J., Zhu, Y., Zhang, Z., and Yang, W. (2019). Modification and Improvement of Microalgae Strains for Strengthening CO2 Fixation from Coal-Fired Flue Gas in Power Plants. Bioresour. Tech. 291, 121850. doi:10.1016/j.biortech.2019.121850
de Jaeger, L., Springer, J., Wolbert, E. J. H., Martens, D. E., Eggink, G., and Wijffels, R. H. (2017). Gene Silencing of Stearoyl-ACP Desaturase Enhances the Stearic Acid Content in Chlamydomonas Reinhardtii. Bioresour. Tech. 245, 1616–1626. doi:10.1016/j.biortech.2017.06.128
Demirbas, A. (2016). Calculation of Higher Heating Values of Fatty Acids. Energy Sourc. A: Recovery, Utilization, Environ. Effects 38, 2693–2697. doi:10.1080/15567036.2015.1115924
Deng, L., and Deng, Q. (2018). The Basic Roles of Indoor Plants in Human Health and comfort. Environ. Sci. Pollut. Res. 25, 36087–36101. doi:10.1007/s11356-018-3554-1
Du, B., Tandoc, M. C., Mack, M. L., and Siegel, J. A. (2020). Indoor CO 2 Concentrations and Cognitive Function: A Critical Review. Indoor Air 30, 1067–1082. doi:10.1111/ina.12706
Durán, I., Rubiera, F., and Pevida, C. (2018). Microalgae: Potential Precursors of CO2 Adsorbents. J. CO2 Utilization 26, 454–464. doi:10.1016/j.jcou.2018.06.001
Enamala, M. K., Enamala, S., Chavali, M., Donepudi, J., Yadavalli, R., Kolapalli, B., et al. (2018). Production of Biofuels from Microalgae - A Review on Cultivation, Harvesting, Lipid Extraction, and Numerous Applications of Microalgae. Renew. Sust. Energ. Rev. 94, 49–68. doi:10.1016/j.rser.2018.05.012
Francisco, É. C., Neves, D. B., Jacob-Lopes, E., and Franco, T. T. (2010). Microalgae as Feedstock for Biodiesel Production: Carbon Dioxide Sequestration, Lipid Production and Biofuel Quality. J. Chem. Technol. Biotechnol. 85, 395–403. doi:10.1002/jctb.2338
Gerotto, C., Norici, A., and Giordano, M. (2020). Toward Enhanced Fixation of CO2 in Aquatic Biomass: Focus on Microalgae. Front. Energ. Res. 8. doi:10.3389/fenrg.2020.00213
González-Martín, J., Kraakman, N. J. R., Pérez, C., Lebrero, R., and Muñoz, R. (2021). A State-Of-The-Art Review on Indoor Air Pollution and Strategies for Indoor Air Pollution Control. Chemosphere 262, 128376. doi:10.1016/j.chemosphere.2020.128376
Haire, T. C., Bell, C., Cutshaw, K., Swiger, B., Winkelmann, K., and Palmer, A. G. (2018). Robust Microplate-Based Methods for Culturing and In Vivo Phenotypic Screening of Chlamydomonas Reinhardtii. Front. Plant Sci. 9, 1–10. doi:10.3389/fpls.2018.00235
Health Organization, W. (2016). Ambient Air Pollution: a Global Assessment of Exposure and burden of Disease. Clean. Air J. 26, 6. doi:10.17159/2410-972x/2016/v26n2a4
Hess, D., Napan, K., McNeil, B. T., Torres, E. M., Guy, T., McLean, J. E., et al. (2017). Quantification of Effects of Flue Gas Derived Inorganic Contaminants on Microalgae Growth System and End Fate of Contaminants. Algal Res. 25, 68–75. doi:10.1016/j.algal.2017.04.007
Lichtenthaler, H. K. (1987). Chlorophylls and Carotenoids: Pigments of Photosynthetic Biomembranes. Methods Enzymol. 148, 350–382. doi:10.1016/0076-6879(87)48036-1
Lu, Q., Ji, C., Yan, Y., Xiao, Y., Li, J., Leng, L., et al. (2019). Application of a Novel Microalgae‐film Based Air Purifier to Improve Air Quality through Oxygen Production and fine Particulates Removal. J. Chem. Technol. Biotechnol. 94, 1057–1063. doi:10.1002/jctb.5852
Maneechote, W., Cheirsilp, B., Srinuanpan, S., and Pathom-aree, W. (2021). Optimizing Physicochemical Factors for Two-Stage Cultivation of Newly Isolated Oleaginous Microalgae from Local lake as Promising Sources of Pigments, PUFAs and Biodiesel Feedstocks. Bioresour. Tech. Rep. 15, 100738. doi:10.1016/j.biteb.2021.100738
Megahed, N. A., and Ghoneim, E. M. (2021). Indoor Air Quality: Rethinking Rules of Building Design Strategies in post-pandemic Architecture. Environ. Res. 193, 110471. doi:10.1016/j.envres.2020.110471
Moheimani, N. R. (2016). Tetraselmis Suecica Culture for CO2 Bioremediation of Untreated Flue Gas from a Coal-Fired Power Station. J. Appl. Phycol. 28, 2139–2146. doi:10.1007/s10811-015-0782-3
Mondal, M., Khan, A. A., and Halder, G. (2019). Estimation of Biodiesel Properties Based on Fatty Acid Profiles of Chlamydomonas Sp. BTA 9032 and Chlorella Sp. BTA 9031 Obtained under Mixotrophic Cultivation Conditions. Biofuels 12, 1175–1181. doi:10.1080/17597269.2019.1600453
Moon, M., Kim, C. W., Park, W.-K., Yoo, G., Choi, Y.-E., and Yang, J.-W. (2013). Mixotrophic Growth with Acetate or Volatile Fatty Acids Maximizes Growth and Lipid Production in Chlamydomonas Reinhardtii. Algal Res. 2, 352–357. doi:10.1016/j.algal.2013.09.003
Nowicka, B., Pluciński, B., Kuczyńska, P., and Kruk, J. (2016). Physiological Characterization of Chlamydomonas Reinhardtii Acclimated to Chronic Stress Induced by Ag, Cd, Cr, Cu and Hg Ions. Ecotoxicology Environ. Saf. 130, 133–145. doi:10.1016/j.ecoenv.2016.04.010
Osorio, H., Jara, C., Fuenzalida, K., Rey-Jurado, E., and Vásquez, M. (2019). High-efficiency Nuclear Transformation of the Microalgae Nannochloropsis Oceanica Using Tn5 Transposome for the Generation of Altered Lipid Accumulation Phenotypes. Biotechnol. Biofuels 12, 134. doi:10.1186/s13068-019-1475-y
Pion, M., and Givel, M. S. (2004). Airport Smoking Rooms Don't Work. Tob. Control. 13, 37i–40. doi:10.1136/tc.2003.005447
Promoters, O., Fermentation, O., Puri, M., Thyagarajan, T., Gupta, A., and Barrow, C. J. (2014). Omega-3 Fat. Available at://publication/uuid/6658F884-5B99-472D-9E6D-6C49A51541F9.
Puzanskiy, R., Tarakhovskaya, E., Shavarda, A., and Shishova, M. (2018). Metabolomic and Physiological Changes of Chlamydomonas Reinhardtii (Chlorophyceae, Chlorophyta) during Batch Culture Development. J. Appl. Phycol. 30, 803–818. doi:10.1007/s10811-017-1326-9
Qari, H. A., and Oves, M. (2020). Fatty Acid Synthesis by Chlamydomonas Reinhardtii in Phosphorus Limitation. J. Bioenerg. Biomembr. 52, 27–38. doi:10.1007/s10863-019-09813-8
Ramos, M. J., Fernández, C. M., Casas, A., Rodríguez, L., and Pérez, Á. (2009). Influence of Fatty Acid Composition of Raw Materials on Biodiesel Properties. Bioresour. Tech. 100, 261–268. doi:10.1016/j.biortech.2008.06.039
Scranton, M. A., Ostrand, J. T., Fields, F. J., and Mayfield, S. P. (2015). Chlamydomonas as a Model for Biofuels and Bio‐products Production. Plant J. 82, 523–531. doi:10.1111/tpj.12780
Siaut, M., Cuiné, S., Cagnon, C., Fessler, B., Nguyen, M., Carrier, P., et al. (2011). Oil Accumulation in the Model green Alga Chlamydomonas Reinhardtii: Characterization, Variability between Common Laboratory Strains and Relationship with Starch Reserves. BMC Biotechnol. 11, 7. doi:10.1186/1472-6750-11-7
Srivastava, G., Nishchal, , and Goud, V. V. (2017). Salinity Induced Lipid Production in Microalgae and Cluster Analysis (ICCB 16-BR_047). Bioresour. Tech. 242, 244–252. doi:10.1016/j.biortech.2017.03.175
Sun, H., Mao, X., Wu, T., Ren, Y., Chen, F., and Liu, B. (2018). Novel Insight of Carotenoid and Lipid Biosynthesis and Their Roles in Storage Carbon Metabolism in Chlamydomonas Reinhardtii. Bioresour. Tech. 263, 450–457. doi:10.1016/j.biortech.2018.05.035
Talhout, R., Schulz, T., Florek, E., van Benthem, J., Wester, P., and Opperhuizen, A. (2011). Hazardous Compounds in Tobacco Smoke. Ijerph 8, 613–628. doi:10.3390/ijerph8020613
Van Den Hende, S., Vervaeren, H., and Boon, N. (2012). Flue Gas Compounds and Microalgae: (Bio-)chemical Interactions Leading to Biotechnological Opportunities. Biotechnol. Adv. 30, 1405–1424. doi:10.1016/j.biotechadv.2012.02.015
Veillette, M., Giroir-Fendler, A., Faucheux, N., and Heitz, M. (2018). Biodiesel from Microalgae Lipids: from Inorganic Carbon to Energy Production. Biofuels 9, 175–202. doi:10.1080/17597269.2017.1289667
Vidyashankar, S., VenuGopal, K. S., Swarnalatha, G. V., Kavitha, M. D., Chauhan, V. S., Ravi, R., et al. (2015). Characterization of Fatty Acids and Hydrocarbons of Chlorophycean Microalgae towards Their Use as Biofuel Source. Biomass and Bioenergy 77, 75–91. doi:10.1016/j.biombioe.2015.03.001
Wang, K., Khoo, K. S., Chew, K. W., Selvarajoo, A., Chen, W.-H., Chang, J.-S., et al. (2021). Microalgae: The Future Supply House of Biohydrogen and Biogas. Front. Energ. Res. 9, 1–9. doi:10.3389/fenrg.2021.660399
Wang, L., Xue, C., Wang, L., Zhao, Q., Wei, W., and Sun, Y. (2016). Strain Improvement of Chlorella Sp. For Phenol Biodegradation by Adaptive Laboratory Evolution. Bioresour. Tech. 205, 264–268. doi:10.1016/j.biortech.2016.01.022
Wang, Z. T., Ullrich, N., Joo, S., Waffenschmidt, S., and Goodenough, U. (2009). Algal Lipid Bodies: Stress Induction, Purification, and Biochemical Characterization in Wild-type and Starchless Chlamydomonas Reinhardtii. Eukaryot. Cel 8, 1856–1868. doi:10.1128/EC.00272-09
Yang, L., Chen, J., Qin, S., Zeng, M., Jiang, Y., Hu, L., et al. (2018). Growth and Lipid Accumulation by Different Nutrients in the Microalga Chlamydomonas Reinhardtii. Biotechnol. Biofuels 11, 1–12. doi:10.1186/s13068-018-1041-z
Zhao, Q., Jin, G., Liu, Q., Pan, K., Zhu, B., and Li, Y. (2021). Tolerance Comparison Among Selected Spirulina Strains Cultured under High Carbon Dioxide and Coal Power Plant Flue Gas Supplements. J. Ocean Univ. China 20, 1567–1577. doi:10.1007/s11802-021-4783-3
Keywords: fatty acids, smoke effect, growth, Chlamydomonas, biodiesel
Citation: Barati B, Fazeli Zafar F, Amani Babadi A, Hao C, Qian L, Wang S and El-Fatah Abomohra A (2022) Microalgae as a Natural CO2 Sequester: A Study on Effect of Tobacco Smoke on Two Microalgae Biochemical Responses. Front. Energy Res. 10:881758. doi: 10.3389/fenrg.2022.881758
Received: 23 February 2022; Accepted: 07 March 2022;
Published: 31 March 2022.
Edited by:
Mohammad Rehan, King Abdulaziz University, Saudi ArabiaReviewed by:
Muhammad Abdul Qyyum, Sultan Qaboos University, OmanAbdul-Sattar Nizami, Government College University, Lahore, Pakistan
Copyright © 2022 Barati, Fazeli Zafar, Amani Babadi, Hao, Qian, Wang and El-Fatah Abomohra. This is an open-access article distributed under the terms of the Creative Commons Attribution License (CC BY). The use, distribution or reproduction in other forums is permitted, provided the original author(s) and the copyright owner(s) are credited and that the original publication in this journal is cited, in accordance with accepted academic practice. No use, distribution or reproduction is permitted which does not comply with these terms.
*Correspondence: Shuang Wang, YWxleGp1dmVuQHVqcy5lZHUuY24=