- 1Department of Environmental Sciences, Government College University Faisalabad, Faisalabad, Pakistan
- 2Soil and Environment Biotechnology Division, National Institute for Biotechnology and Genetic Engineering (NIBGE), Faisalabad, Pakistan
- 3CAS-Key Laboratory of Crust-Mantle Materials and the Environments, School of Earth and Space Sciences, University of Science and Technology of China, Hefei, China
- 4Department of Land, Air and Water Resources, University of California, Davis, Davis, CA, United States
- 5Botany and Microbiology Department, College of Science, King Saud University, Riyadh, Saudi Arabia
Crude oil is causing widespread pollution in both aquatic and terrestrial environments. Phytoremediation, which is an emerging technology, involves the efficient use of plant species to remove, detoxify, and/or immobilize contaminants in the soil through natural processes. For this study, Para grass (Brachiaria mutica) inoculated with a previously isolated and characterized bacterial consortium was grown in a pot containing crude oil-contaminated soil. The effects of different concentrations (0.01, 0.1, and 1% of 10% detergent solution in ultrapure water) of nonionic surfactant (Triton X-100) on the degradation of crude oil in contaminated soil were observed. After 4-month experimentation, the maximum growth of the plant root length (28.57 cm), shoot length (65.73 cm), and dry biomass of root and shoot (92.42 g) in the pot having an application of surfactants of 0.1% augmented with the bacterial consortium (T7) was observed. Increasing the concentration of Triton X-100 from 0.01 to 1% augmented with a bacterial consortium led to the maximum removal of total petroleum hydrocarbons ranging between 6 and 40%. This is an indication of the inhibiting effect of Triton X-100 above 0.1% on the growth of plants. Furthermore, the hydrocarbon degradation was confirmed by the FTIR study that can be attributed to the adopted plant species' ability to degrade crude oil contamination, and it is evident through the FTIR results after 120 days of experimentation that the different functional groups are responsible for petroleum hydrocarbons present in soil samples. The current study concludes that the application of integrated treatment of crude oil-contaminated soil by using Triton X-100 and augmented with microbes can help to restore polluted soils for agricultural use. Future strategies such as bioaugmentation of contaminated soil with PGPR and the use of genetically modified (GMO) plants may result in amplifying plant tolerance and ultimately lower the level of soil pollutants for better soil health and boost plant yield.
Introduction
Soil contamination from unrefined petroleum despoiled soil is an elementary worldwide problem. This is normally the aftereffect of improper storing and removal, irresponsible chronicle releases, incidental breaks, fuel tank spills, and oil drain off (Wang et al., 2011). Crude oil is the world’s most powerful energy source. However, conventional fossil oil mining and drilling activities have significant environmental consequences. Crude oil contains several compounds that are cytotoxic, carcinogenic, and mutagenic and threatens the environment and human health significantly (Dickinson, 2017). Reducing petroleum hydrocarbon compounds in the toxic atmosphere has become a major challenge in the petrochemical technologies used to treat soil contaminated by petroleum hydrocarbons. They are, however, usually expensive, inefficient, and rarely remain neutral (Ezeji et al., 2007). Therefore, it is important to create remediation advances that are financially and environmentally achievable, harmless to the ecosystem, quick, and relevant in a wide scope of actual conditions (Farag et al., 2018). Phytoremediation is a promising technology being used to clean polluted and contaminated sites. This technology has shown its economic sustainability and benefits for long-term uses (Kanwal et al., 2019; Abiodun, 2010).
This technology involves the efficient use of plant species to remove or detoxify contaminants in a growth matrix (soil, water, or sediments) through natural processes (Kumaria et al., 2021). For this purpose, local plants or grasses may grow on the shoreline of water pounds (Cronk and Fennessy, 2001) with fast-growing traits. The current study aimed to study the local plant, e.g., Brachiaria mutica, to access it as a potential candidate for remediation of soil contaminated with crude oil. Phytoremediation with Brachiaria mutica (Para grass), which was used in this study, has several notable characteristics. These are fast-growing perennial plants (Kassaye et al., 2017). It has developed an extensive taproot system, forming a wide range of habitats for rhizospheric microbes (Kirk et al., 2005). It is linked to symbiotic rhizobia bacteria that enable nitrogen fixation (Kanwal et al., 2021; Kirk et al., 2005). They are found all over the world and are well suited to a variety of climates. These plants have been commonly used in phytoremediation over the last decade. These species have phytoremediation of petroleum hydrocarbons into polycyclic aromatic hydrocarbons (PAHs) (Wiltse et al., 1998). Moreover, the process of remediation may be enhanced by using a surfactant known as soil washing. They have been proven to be a key mechanism to remove a mixture of contaminants, for instance, petroleum and volatile organic chemicals, heavy metals, herbicides, pesticides, and other hazardous and non-biodegradable compounds from contaminated sites (water or soils) (Rosen, 2004; Mahmood et al., 2021). A few recent studies have also indicated that the use of surfactants may enhance the biodegradation of contaminants from soils (Kirk et al., 2002). They have hydrophobic as well as hydrophilic regions that can efficiently alter the oil–water interface character (Liu et al., 2018) and play a key function in situ remediating polluted sites. Besides chemical and physical techniques, phytoremediation is environmentally friendly, economical, and easy to implement with relatively simple and single operation processes (Al-Doury, 2019).
During the last few years, extensive production and use of biodegradable surfactants have completely reduced the adverse signs of surfactants in the soil environment (Qin et al., 2019). Although surfactant-enhanced bioremediation (SEB) has successfully applied biological application treatment of oily waste using microbial consortia (Hamme and Ward, 2001; Ward et al., 2003), there are still many challenges that limit its use in oil spill bioremediation conditions. Controlled lab-scale studies reveal a variety of important interactions and outcomes that may adversely affect oil repair when surfactants are available. This study highlights various challenges to be considered for the effective use of SEB, focusing on the use of synthetic surfactants for SEB. Surfactants can improve the bioavailability of hydrophobic mixtures, which is a property used to upgrade the phytoremediation impact of surfactants. The chief mechanism behind the phytoremediation technique is the microbial degradation of contaminants, particularly petroleum compounds. Degradation via soil microbes mainly requires optimum moisture conditions and is, therefore, limited by a mass transfer mechanism (Kim and Weber, 2003). Such type of degradation process may be enhanced by the treatment of surfactants, therefore enhancing phytoremediation efficacy (Tang et al., 1998). Other reports have suggested that the presence of surfactants prevents degradation and can reduce the adhesion of bacteria in hydrophobic environments (Stelmack et al., 1999). This machine may be useful for the destruction of almost non-soluble pollutants, so the use of water-repellent materials may be counterproductive. One report suggested that the special use of surfactants by PAH contaminants causes the apparent inhibition of hydrocarbon biodegradation (Deschênes et al., 1996).
Other reports have suggested that the presence of surfactants promotes environmental degradation. The most important effect of surfactants on the interaction between soil and air pollutants is the promotion of the transport of large amounts of pollutants from the soil to the aquatic phase (Salanitro et al., 1997). The addition of surfactants improved hydrocarbon (HOC) desorption and, as a result, facilitated their movement to the associated water phase from the solid phase. The extent of successful microbial biodegradation and, ultimately, bioremediation depends on multiple factors such as availability of nutrients, particularly N and P (needed for microbial cell growth), soil pH, moisture content, and temperature. The bacterial consortium showed a significantly higher degradation of crude oil (Mukherjee and Bordoloi, 2011). The process of bioremediation is a multi-stage phenomenon. It has been observed that a single bacterial strain is not effective to degrade any contaminant (chemical) to less-toxic or non-hazardous forms. If we use different strains for the same process, it helps in the transformation of the contaminant into different forms of its degraded products, thereby achieving complete biodegradation (Patowary et al., 2016). It has also been reported that microbial interactions (e.g., co-metabolism) also play a critical role in effective biodegradation. The selection of different microbial strains for the consortium is very crucial. The addition of a mixed consortium of bacteria helps in the degradation of hydrocarbons.
The basic objective behind the current study was to enhance the degradation rate of petroleum hydrocarbons through the addition of surfactant TritonX-100, a bacterial consortium combined with phytoremediation, as a stimulating agent for the degradation of crude oil contamination with varying experimental conditions. Various plants such as ryegrass, alfalfa, birdsfoot, Bermuda grass, nodding beggar, and maize (L. multiflorum; M. sativa; L. corniculatus; C. dactylon; B. cernua; and Z. mays are their scientific names, respectively) have been used to prepare hydrocarbon-contaminated soil (Khan et al., 2013). However, the addition of Brachiaria mutica grasses containing indigenes has not been reported to improve the soil contaminated with crude oil. In addition, the effect of synthetic surfactants in the treatment of bacterial consortium augmented phytoremediation has not been fully investigated.
Materials and Methods
Growth Conditions
Soil samples from a contaminated site at a depth of 60 cm were collected from an “oil production and exploration company” situated in the district of Chakwal, Pakistan (32.988682°N, 72.925756°E). These soil samples were ground using a mortar and pestle, air-dried, sieved via a 2 mm mesh, homogenized, and analyzed for various physiochemical parameters (Table 1) as described by Mohanty and Patra (2011). Plant root and shoot samples were also collected from contaminated soil pots for the measurement of growth parameters such as root and shoot length and fresh and dry weight of root and shoot after 60–120 days.
Experimental Setup
For the experiment, locally available perennial species of Para grass (Brachiaria mutica) plants were used as a phytoremediation entity (Dietz and Schnoor, 2001; Collins, 2007). The standard method of propagating Brachiaria mutica is by planting enrooted cuttings as they are easy to handle and economical too (Schaff et al., 2002). The cuttings of these plants were collected from the natural fields in nearby areas and soaked in clean water for 10 days to increase their survival and growth rate. As soon as an initial root emerged from soaked cuttings, they were planted in a pot according to the treatment plan. Different concentrations of solubilized Triton X-100 were used as a surfactant. All the treatments were used in triplicates. The detailed treatment plan is described as follows:
C1: soil + plant
T1: soil + crude oil + plant
T2: soil + crude oil + plant + bacteria
T3: soil + crude oil + plant + surfactant (0.01%)
T4: soil + crude oil + plant + surfactant (0.1%)
T5: soil + crude oil + plant + surfactant (1.0%)
T6: soil + crude oil + plant + surfactant (0.01%) + bacteria
T7: soil + crude oil + plant + surfactant (0.1%) + bacteria
T8: soil + crude oil + plant + surfactant (1.0%) + bacteria
The physical investigation of the soil revealed that it belonged to the taxonomic category of loamy sand. The soil was slightly acidic, with mean pH values of 6.5 and 4.5. The pH of the soil plays an important role in the adsorption of heavy metals and regulates the hydrolysis and solubility of metal carbonate, phosphate, and hydroxide (Afzal et al., 2011). Although EC has no direct effect on plant growth, it can be used to determine the number of nutrients available for plant uptake and the salinity level of soil that can inhibit microbial growth (Anwar ul Haq et al., 2018).
Total Microbial Count
The total microbial count was determined by using the pure plate technique after 60 days of overtime zero according to Samuel et al. (2018) with minor modifications. From each pot, 1 g of soil was taken. Colonies that developed after incubation were counted, and then, the total bacteria count was measured and expressed as CFU (colony-forming units) per gram of each soil sample.
Measurement of Plant Parameters
The simplest and most obvious agronomic parameters such as shoot and root length (cm) and shoot and root, both dry and fresh, weight (g) were measured by using a meter rod and analytical balance, respectively, at the time of harvest after the 4-month experiment.
Fourier-Transform Infrared Spectroscopy
The most applicable technique to get quality and functional group-related information about petroleum-related materials is the Fourier-transform infrared (FTIR) analysis technique. The technique uses IR (infrared) light to scan and observe the chemical properties of test samples. Samples were analyzed by FTIR spectroscopy to check the different functional groups of crude oil-contaminated soil (COCS). Samples were ground and mixed with 1 mg of contaminated soil with 200 mg of KBr (IR grade). With a hydraulic press system, the samples were transformed into a pellet. The pellet sample was analyzed by using an FTIR spectrophotometer (Thermo Fisher Scientific, United States). An absorbance spectrum was collected in the range of 400–4,000 cm−1 (Aziz et al., 2015).
Data and Statistical Analysis
The parameters for plant growth and the amount of TPH are presented as mean ± SE of three replicates. The statistical significance of differences among treatments at p < 0.05 was analyzed.
Results and Discussion
Plant Tolerance
Removal of crude oil is due to the activities of naturally occurring soil microorganisms in non-vegetated soil, and this soil microbial activity increases if plants are present in the soil that aid the breakdown of the pollutants (Juck et al., 2000) The roots play an important role because they provide a large surface area for the degradation of crude oil and provide nutrients which are essential for the growth of microorganisms (Ma et al., 2016). Hyperaccumulator plants such as Pterisvittata are very effective and potentially useful for the removal of crude oil-contaminated soil. Soil pH is affected by a variety of factors, including mineral concentration and texture of the soil (Njoku et al., 2008). The soil which is used in the experiment was alkaline. It has been studied that crude oil contamination increases soil acidity and makes soil toxic. Reduced pH has been studied as phytoextraction, an effective technique for the removal of heavy metals that are present in contaminated soil. Naturally, the plant absorbs ammonium which decreases the pH and has been shown to lower the rhizosphere (Villegas and Fortin, 2001). During the research, the moisture content of the soil changed across a wider range due to variables such as wind, watering frequency, humidity, and plant transpiration rate.
All studied growth parameters showed significantly reduced growth under contaminated soil environments. Shoot length as a function of experimental time is presented in Figure 1). The value of shoot length after 60 days showed (62–86 cm, 43.43 g) no non-significant increase compared to that measured at the harvesting time (65.73 cm, 53.94 g). This confirmed a stunted growth behavior under crude oil-contaminated environments. Similarly, throughout the growth period of the plant, root growth was inhibited. However, plants augmented with a mixed consortium of bacteria help in growth along with different levels of chemical surfactant (Triton X-100). The maximum growth of the plant (root and shoot length and dry biomass) was observed in the pot having an application of surfactants (0.1%) augmented with the bacterial consortium (Figures 1, 2). After 60–120 days, plant dry biomass was observed and found in the form of root and shoot dry weight, and it was not increased significantly as affected in the co-contaminated soil and showed premature death. Growth rates continuously increased with time but by a lesser amount as compared to initial harvesting at 60 days (Figure 2).
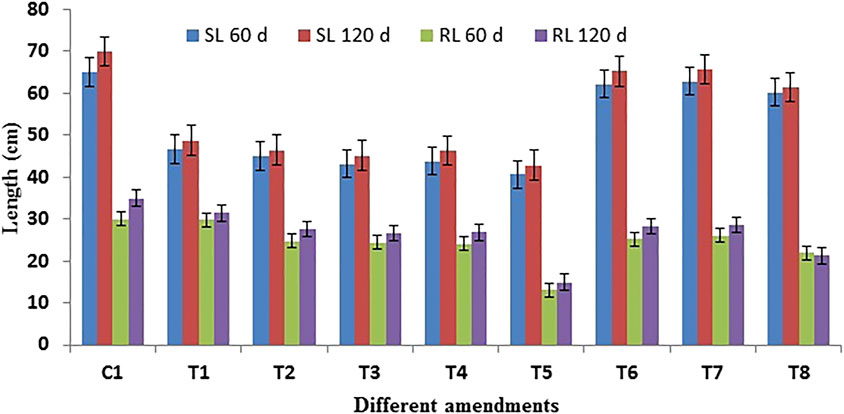
FIGURE 1. Growth response of Para grass under different amendments representing the average length (SL: shoot length; RL: root length). Control: C1, P: T1, PB: T2, PS1: T3, PS2: T4, PS3: T5, PS1B: T6, PS2B: T7, and PS3B: T8 carried on with crude oil-contaminated soil. Values are expressed as mean ± standard errors of triplicate measurements.
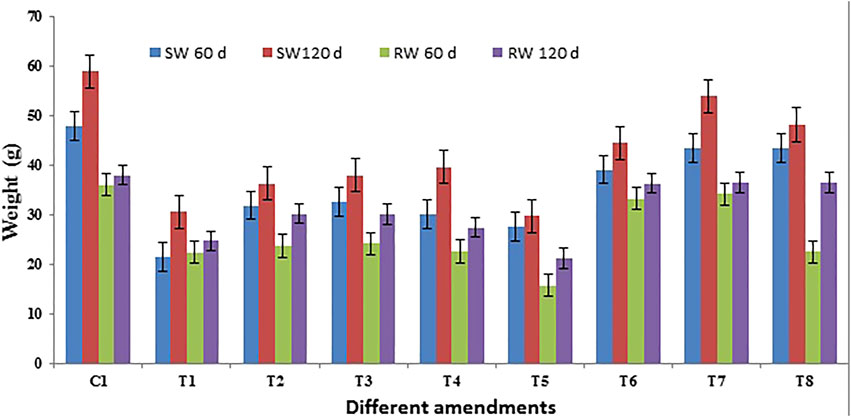
FIGURE 2. Biomass of Pare grass under different treatments representing the average weight (SW: shoot weight; RW: root weight). Control: C1, P: T1, PB: T2, PS1: T3, PS2: T4, PS3: T5, PS1B: T6, PS2B: T7, and PS3B: T8 carried on with crude oil-contaminated soil. Values are expressed as mean ± standard errors of triplicate measurements.
The contaminated soil (crude oil) showed injurious effects on plant growth, thus leading to adverse effects on all studied growth traits, particularly the germination process. The process of germination, being sensitive, may be influenced by the presence of soil contaminants. Within the rhizosphere, heavy metals inhibit the water uptake by young seedlings (Kranner and Colville, 2011; Ko et al., 2012). Oil coating on the soil surface may reduce the diffusion of O2 and water uptake by growing plants (Baker, 1970), and consequently, it lowers the rate of plant growth in undercontaminated soil environments (PeraltaVidea et al., 2002; Mehdi et al., 2021). Furthermore, total biomass was significantly reduced under a polluted soil medium (Monalisa and Patra, 2012). The present findings were consistent with the findings of Peralta-Videa et al. (2002), who found that the simultaneous presence of hydrocarbons significantly affects plant growth. The length of both root and shoot along with the dry biomass of the plant was hampered due to stress exerted by both types of contaminants in the growth medium. Concerning plant sensitivity to petroleum hydrocarbons, seedling growth was stunted under higher levels of total petroleum hydrocarbons (TPHs) than previously reported (Kirk et al., 2005; Aslam et al., 2021).
The concurrent existence of heavy metals along with oil-based hydrocarbon pollutants may boost toxicity in plants. The agronomic parameters are one of the indicators while investigating the overall health of a plant. Our data regarding root and shoot biomass showed that plant roots were more sensitive to the toxic effects of pollutants in the soil as compared to shoots. The direct contact between soil pollutants and the surface of roots may be the major factor in root sensitivity (Kummerová et al., 2013) overshoots. Both direct as well as indirect phytotoxicity mechanisms of heavy metals and petroleum hydrocarbons were evident in affecting the physiology of plants by changing the physical, chemical, and biological aspects of the soil (Baker, 1970; Kabata-Pendias, 2011). Maximum above-ground yield is a prerequisite for an efficient phytoextraction process; on the other hand, the development of a dense plant root network generates a productive habitat for rhizosphere-based microbes involving rhizodegradation.
Bioavailability of Hydrocarbons in Contaminated Soil Influenced by Surfactants
For different concentrations of Triton X-100, the fractions of oil decreased with the low concentration of Triton X-100 (Figure 3). The fractions of degraded oil ranged from 6 to 40 percent. The maximum removal of oil fractions from the soil after 30 days of treatment was as follows: 26% at 0.01% of Triton X-100, < 27% at 0.1% of Triton X-100, and > 25.4% at 1% of Triton X-100. The maximum removal of oil fractions from the soil after 60 days of treatment was as follows: 32% at 0.01% of Triton X-100, < 33% at 0.1% of Triton X-100, and > 32.4% at 1% of Triton X-100. The removal of oil fractions from the soil after 90 days of treatment was as follows: 34% at 0.01% of Triton X-100, < 36.2% at 0.1% of Triton X-100, and < 37% at 1% of Triton X-100. The removal of oil fractions from the soil after 120 days of treatment was observed as follows: 38.98% at 0.01% of Triton X-100, < 40.33% at 0.1% of Triton X-100, and > 38% at 1% of Triton X-100. However, the increase in the concentration of Triton X-100 from 0.01 to 1% led to the increased removal of total petroleum hydrocarbons at moderate concentration. This is indicative of the inhibiting effect of Triton X-100 above 0.1% on the growth of plants. In the current study, the population of microorganisms was increased in the samples with both crude oil and Triton X-100. This suggests that Triton X-100 could be used as a carbon source and was not harmful to the microbes. Some reports have shown that both nonionic and anionic surfactants increase the dissolution of hydrocarbons by forming micelles. The surfactants begin to combine into micelles at critical micelle concentration (CMC), and the interior of the micelles provides a hydrophobic environment to dissolve soluble compounds, such as hydrocarbons (Roy et al., 1994). The higher reduction of TPH was observed with a higher concentration of Triton X-100 due to the nonionic character of surfactants that increases the solubility of hydrocarbons.
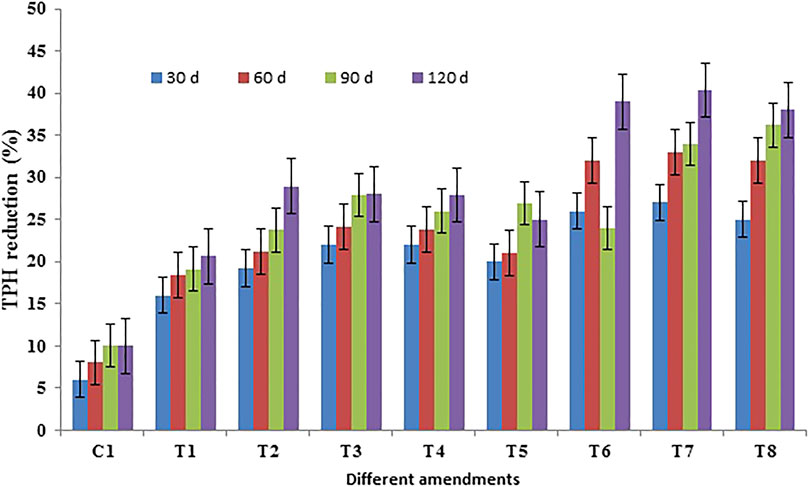
FIGURE 3. Reduction of total petroleum hydrocarbon (TPH) during the phytoremediation of crude oil-contaminated soil (control: C1, P: T1, PB: T2, PS1: T3, PS2: T4, PS3: T5, PS1B: T6, PS2B: T7, and PS3B: T8 carried on with crude oil-contaminated soil).
Microbial Population
For microbial consortium treatment, a variety of methods are used, including seed coverings, soil injections, rhizosphere injections, and leaf injections. However, the effects of such injection methods on the effectiveness of phytoremediation have not been evaluated. In this study, the production of most biomass of plants, the reduction of toxicity of hydrocarbon degradation, and the bacterial colony were identified as soil based compared with other methods (Mahmood et al., 2021; Afzal et al., 2012). However, other previous studies have reported that seed coverage with a microbial consortium works better compared to other vaccines (Müller and Berg, 2008; Müller et al., 2009). In addition, inoculation is a well-established method and is often used to detect seed germination quickly and consistently. Seed extraction has been used successfully in the phytoremediation of organic pollutants such as hydrocarbons (Afzal et al., 2011). If you add a beneficial microbial consortium to contaminated soil, the inoculated bacteria must be able to adapt quickly to the new environment and survive. In addition, the survival and persistence of the inoculated beneficial bacterial consortium are essential for the growth and health of plants in an effective repair system where plants and microorganisms interact with each other (Afzal et al., 2011; Ahmad et al., 2012).
The colony-forming unit (CFU) g−1 of crude oil-contaminated soil in the presence of surfactant as amendment ranged between 6.7 × 107 at the end of 2 months and 2.2 × 104 at the end of the fourth month of applying 0.01% of surfactant Triton X-100. The crude oil-polluted soil amended with 0.1% of surfactant Triton X-100 ranged from 5.8 × 107 at the end of 2 months and 6.4 × 107 at the end of the fourth month of treatment, while that of crude oil-polluted soil amended with 1.0% of surfactant Triton X-100 ranged from 6.2 × 107 at the end of 2 months and 8.2 × 107 at the end of the fourth month of treatment, respectively (Table 2). By applying Triton X-100 combined with crude oil, the total colony count showed an increased bacterial population. On the contrary, under control conditions (without oil contaminants), the bacterial population was slightly lower than that in other treatment samples. The samples with Triton X-100 showed non-significant differences regarding colony count. During the 120 days of the growth period, the control sample did not show an increasing trend.
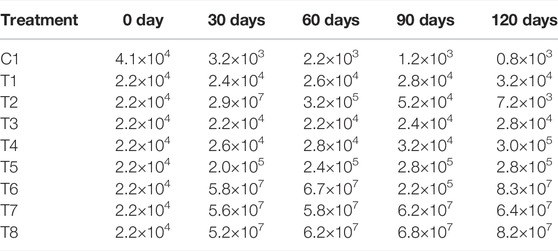
TABLE 2. Total microbial counts in soil under different treatments during phytoremediation experiment.
The application of biosurfactants has been published in improving the bioavailability of hydrocarbons within the soil and, as a result, increased microbial-based pollutant degradation (Marchant and Banat, 2012). This may be due to the ability of rhamnolipids to enhance both types of interactions (hydrophilic and hydrophobic) that lead to blocking the formation of H-bonds, lowering surface tension, and ultimately improving mobilization of oil contents, thus producing oil micelles in water (Marchant and Banat, 2012). Degradation of crude oil in soil enhances nutrient supplementation in contaminated soils. Such a type of microbial degradation was performed by various catabolic pathways. In the case of aerobic oxidation of hydrocarbons, the hydroxylase enzyme performs the terminal or sub-terminal O2 integration, while under anaerobic metabolism, nitrate or sulfate ions act as electron donors, and the addition of the C group promotes alkane activation (Callaghan et al., 2009).
Before the introduction of the mixture of bacteria, the number of indigenous bacteria in the crude oil refinery was determined to be 6.7 × 107CFU/g dry soil. A combination of the Triton x-100 and the bacterial consortium was also made to test the feasibility of using this integrated method in soil preparation. Table 3 shows the efficiency of crude oil during the refining process. In the control group (i.e., unprocessed soil), only 6% of crude oil is degraded which may be due to short-chain bonds and oxidation in the air. The efficacy of T2 group degradation (i.e., soil modified by mixed bacterial consortium inoculation) (29%) was significantly higher than that of the T1 group during all remedial tests, showing that the mixed bacterial consortium was able to adapt quickly to the new contaminated environment of oil and has shown a high degree of degradation of crude oil. The conclusion can also be confirmed by further short-term bioremediation tests. In Figure 3, the number of bacteria increased from 6.7 × 107 to 8.2 × 107 CFU/ g dry soil during the 120-day bioremediation. Mixed bacteria can grow well and, in turn, facilitate the deterioration of crude oil depending on their strength. In the case of the T6 group (i.e., soil modified with a combination of bacterial consortium inoculation and Triton x-100), the degradation efficacy was further improved compared to that of T1 and T2 and up to 40% after 4 days of treatment. Alarcón et al. (2008) found that crude oil depletion was significantly higher (39%) after 180 days of treatment, which was significantly lower than our experimental results. The introduction of the Triton x-100 was used to improve the efficiency of the destruction of crude oil by indigenous microbes (Figure 4). The reason could be that the Triton x-100 could be used as a carbon source by native bacteria and promotes the growth of native bacteria, which was consistent with the results obtained. During the first 60 days of repair, the efficiency of the deterioration increased rapidly. From the beginning, after incubation, there was a high concentration of carbon dioxide in the contaminated soil, and the microorganism was unable to reduce long-chain chains or alkene directly into the petroleum hydrocarbon priority (Chang et al., 2009). From the 60th day to the 120th day of repair, the efficiency of the deterioration tends to be flat. Possible causes may be as follows: 1) crude oil that was easily destroyed is already completely consumed by a mixture of bacteria (Zahed et al., 2010); 2) toxic medium metabolites can be produced and collected (Zhu and Aitken 2010); and 3) with the use of a carbon source, the activity of the bacterial consortium has decreased significantly (Yang et al., 2010).
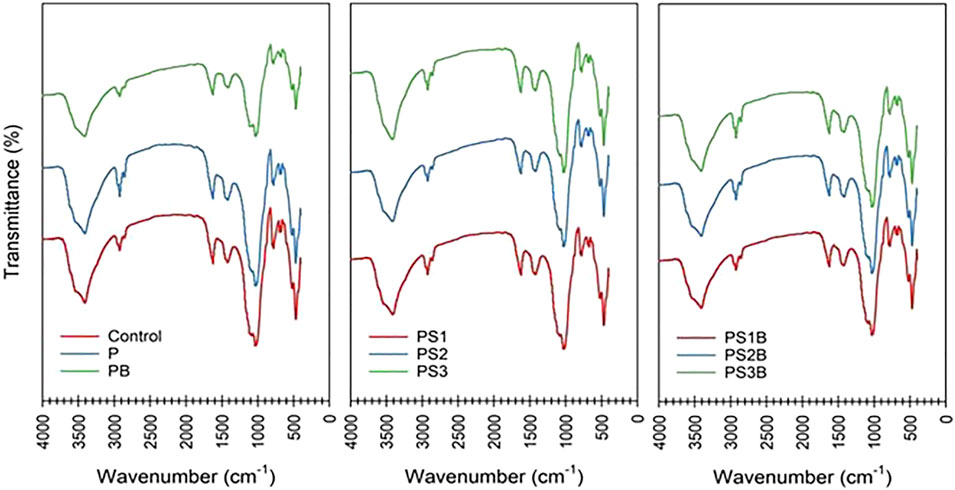
FIGURE 4. FTIR spectrum of the different amendments. Control: C1, P: T1, PB: T2, PS1: T3, PS2: T4, PS3: T5, PS1B: T6, PS2B: T7, and PS3B: T8 carried on with crude oil-contaminated soil.
Under control treatment, the value of log CFU/g of contaminated soil showed lower values than the pots amended with surfactant and ranged between 2.2 × 104 at the end of the second month and 2.8 × 104 at the end of the fourth month of application, respectively. The growth of indigenous oil-degrading microbiota may be activated by surfactant application (Abioye et al., 2010). Similar results were reported when the organic amendment was applied to contaminated soils (Al-Kindi and Abed, 2016). The population of indigenous oil-degrading microbiota rapidly increases in response to increased hydrocarbon availability(Song and Bartha., 1990). The key reason behind the increased log CFU for bacteria under surfactant as an organic amendment for the decontamination of crude oil-based contaminated soil is the availability of more hydrocarbon as a basic nutrient for microbial activities. The addition of surfactant as an amendment improved petroleum hydrocarbon biodegradation by enhancing their availability and solubility to natural soil microbiota.
Surface Chemistry of Contaminated Soil
The most applicable technique to get quality and functional group-related information on petroleum-related materials is the Fourier-transform infrared (FTIR) analysis technique. The technique uses IR (infrared) light to scan and observe the chemical properties of test samples. Samples were analyzed by FTIR spectroscopy to check the different functional groups of crude oil-contaminated soil (COCS). Results regarding the surface chemistry of contaminated oil particles showed similarities amongst surfactants and spectral areas in COCS, although distinct differences also exist in absorption bands, thus pointing out the kinds of functional groups involving bonding interaction. A comprehensive investigation of band shifting and their association with peak area as well as height along with elemental data observed that the curve resolving spectra of COCS showed wide bands at about 3,730–3,001 cm−1 (Sung et al., 2013). Such types of bands are linked with O–H stretching vibrations (Table 4) and are chiefly recognized as belonging to the O–H functional group corresponding to low concentrations of alcoholic, phenolic, and carboxylic acid groups of compounds within COCS. Data suggest that asymmetric stretching of both methyl and methylene functional groups is caused by two larger wavenumber bands in the range of 2,947–2,397 cm−1. In the studied samples, a band that appeared in the range of 1785–1,575 cm−1 was linked with the C=O diaryl ketone stretching phenomenon. All other remaining functional groups that are accountable for IR absorption are as follows: 1,507–1,387 (cis = C–H bending), 1,307–1,097 (–C–O stretch) (Gaskin and Bentham, 2010), 1,067–886 (–C–O stretch), 886–706 cm−1 (–C–Cl stretch), 656–503(–C–Br stretch), and 504–440 (–O– stretch).
Figure 4 presents the FTIR spectra of soil samples with and without any amendments with their functional groups. The results present vital information about the examined soils concerning their surface functional groups as well as traits of chemical bonding. These results were used to classify the functional groups within oil-based contaminants in control soil without any amendments and along with eight different amendments. Without any amendment, it was recognized that the results of FTIR spectra showed numerous functional groups in the contaminated soil sample.
The FTIR spectra result showed that (O–H) functional groups exist within 2,997–3,726 cm−1 wavenumber, (C–H) functional groups from 2,880 to 2,997 cm−1, (Si–H)-based groups from 2397 to 2880cm−1, (C=C) functional groups from 1,344 to 1,504 cm−1, (C–O)-based groups from 1,067 to 1,105 cm−1, (–O–C) functional groups from 838 to 1,067 cm−1, and (Si-O-Si) functional groups from 465 to 594 cm−1. Hydrocarbons group alkanes were confirmed by the presence of O–H and C–H single-bounded bonds. After remediating the contaminated soil with the integrated application of bacterial and surfactant, the result specifies a variety of functional groups in a different soil sample. With the highest spectra ranging from 2,999 to 3,743 cm−1, wavenumber indicated the (O–H) functional group, (C–H) functional groups from 2,880 to 2,947 cm−1, (Si–H)-based compounds from 2,366 to 2,879 cm−1, (C=C)-based groups between 1,343 and 1,560 cm−1, (C–O)-based groups ranging from 1,067 to 1,338 cm−1, (–O–C) groups from 824 to 1,070 cm−1, and (Si-O-Si) functional groups ranging from 434 to 656 cm−1.
These findings confirm that some specific functional groups are accountable for the existence of petroleum hydrocarbons within tested soil samples (Aziz et al., 2015). Functional groups, particularly alcohols, methyl, phenols, aliphatic hydrocarbons, and amides, are responsible for the occurrence of petroleum-based hydrocarbons. Moreover, similar characteristic peaks showed a lower strength under higher oil content concentrations in soil samples. Overall, our findings based on FTIR results may suggest that plant species may help to degrade oil-based soil contamination after 120 days of experimentation. Such alterations strongly suggest that crude oil-based soil contamination was effectively remediated by the process of phytoremediation. Figure 4 shows fluctuations in the FTIR spectrum of COCS and COCS + surfactants at different concentrations. Wavenumbers range from 400 to 4,000 (right to left), whereas absorbance is given on the left side. It can be observed from the aforementioned graph that from 2,000 to 3,000, the asymmetric stretching of methylene is very low and the highest peak of absorbance is shown at 1,000 wavenumber, where CO stretches about 1,031.
Practical Implications of This Study
It has been proven that surfactants used in the remediation of petroleum hydrocarbon-contaminated soil have great application potential. The effects of surfactant (Triton X-100) on the leaching of petroleum hydrocarbons from soil were investigated through various experiments, and petroleum hydrocarbon components were analyzed by FTIR. The effects of surfactants on the degradation of petroleum hydrocarbons were analyzed by the changes in microbial growth. The techniques developed through this study could be applied to field areas contaminated with crude oil.
Conclusion
The present study was conducted by using growing Para grass (Brachiaria mutica) inoculated with a previously isolated and characterized bacterial consortium in the pot containing crude oil-contaminated soil to enhance the degradation rate of petroleum hydrocarbons through the addition of surfactant (Triton X-100) and a bacterial consortium combined with the phytoremediation process. The present study results suggest that the occurrence of petroleum hydrocarbon-based phytotoxicity is perhaps over the threshold level for restricting the survival and growth Brachiaria mutica. While it looks like the existence of pollutants is a vital factor affecting plant growth and yield, the nutrient status of the soil was enough to provide essential nutrients, and thus, no nutrient deficiency symptoms were observed during the experiment. Results regarding the degradation of hydrocarbons suggested that the use of plants is not successful for an efficient degradation process. Regardless of this, the accumulation of contaminants in the roots of plants might lead to the phytostabilization of hydrocarbons. Furthermore, the initial boost in the numbers of hydrocarbon-degrading microbial populations within the rhizosphere of Brachiaria mutica resulted in potential improvement in hydrocarbon degradation. Brachiaria mutica showed improved tolerance toward oil contaminants under lower surfactant (TritonX-100) concentration. Future strategies such as bioaugmentation of contaminated soil with PGPR and the use of genetically modified (GMO) plants may result in amplifying plant tolerance and ultimately lower the level of soil pollutants for better soil health and boost plant yield.
Data Availability Statement
The original contributions presented in the study are included in the article/Supplementary Material, further inquiries can be directed to the corresponding author.
Author Contributions
Conceptualization, MAH and MI. Data curation, MAH, AAA-H and BY. Formal analysis, MAH, AAA-H and HMA. Funding acquisition, MI and AAA-H. Methodology, MAH and MI. Project administration, MAH, AAA-H, BY and MI. Software, MAH and AAA-H. Validation, AAA-H and HMA. Writing—original draft, MAH, MI and AAA-H. Writing—review and editing, BY, MI and HMA. All authors have read and agreed to the published version of the manuscript.
Conflict of Interest
The authors declare that the research was conducted in the absence of any commercial or financial relationships that could be construed as a potential conflict of interest.
Publisher’s Note
All claims expressed in this article are solely those of the authors and do not necessarily represent those of their affiliated organizations, or those of the publisher, the editors, and the reviewers. Any product that may be evaluated in this article, or claim that may be made by its manufacturer, is not guaranteed or endorsed by the publisher.
Acknowledgments
The author would like to acknowledge the technical help of Dr. M. Afzal and Fahad and their editorial support. The laboratory support of NIBGE is also gratefully acknowledged. The authors acknowledge the financial support by Researchers Supporting Project number (RSP-2021/186) King Saud University, Riyadh, Saudi Arabia..
References
Abiodun, S. (2010). Bioremediation of a Crude Oil Polluted Soil with PleurotusPulmonarius and Glomus Mosseae Using Amaranthus Hybrids as a Test Plant. J. Bioremed. Biodegr. 1, 1–6. doi:10.4172/2155-6199.1000113
Abioye, P. O., Abdul Aziz, A., and Agamuthu, P. (2010). Enhanced Biodegradation of Used Engine Oil in Soil Amended with Organic Wastes. Water Air Soil Pollut. 209, 173–179. doi:10.1007/s11270-009-0189-3
Afzal, M., Yousaf, S., Reichenauer, T. G., Kuffner, M., and Sessitsch, A. (2011). Soil Type Affects Plant Colonization, Activity and Catabolic Gene Expression of Inoculated Bacterial Strains during Phytoremediation of Diesel. J. Hazard. Mater. 186, 1568–1575. doi:10.1016/j.jhazmat.2010.12.040
Afzal, M., Yousaf, S., Reichenauer, T. G., and Sessitsch, A. (2012). The Inoculation Method Affects Colonization and Performance of Bacterial Inoculant Strains in the Phytoremediation of Soil Contaminated with Diesel Oil. Int. J. Phytoremediation 14, 35–47. doi:10.1080/15226514.2011.552928
Afzal, M., Khan, S., Iqbal, S., Mirza, M. S., and Khan, Q. M. (2013). Inoculation Method Affects Colonization and Activity of Burkholderia Phytofirmans PsJN during Phytoremediation of Diesel-Contaminated Soil. Int. Biodeterioration Biodegradation 85, 331–336. doi:10.1016/j.ibiod.2013.08.022
Ahmad, F., Iqbal, S., Anwar, S., Afzal, M., Islam, E., Mustafa, T., et al. (2012). Enhanced Remediation of Chlorpyrifos from Soil Using Ryegrass (Lollium Multiflorum) and Chlorpyrifos-Degrading Bacterium Bacillus Pumilus C2A1. J. Hazard. Mater. 237-238, 110–115. doi:10.1016/j.jhazmat.2012.08.006
Al-Doury, M. M. I. (2019). Treatment of Oily Sludge Produced from Baiji Oil Refineries Using Surfactants. Pet. Sci. Technology 37, 718–726. doi:10.1080/10916466.2019.1566247
Al-Kindi, S., and Abed, R. M. M. (2016). Comparing Oil Degradation Efficiency and Bacterial Communities in Contaminated Soils Subjected to Biostimulation Using Different Organic Wastes. Water Air Soil Pollut. 227, 36. doi:10.1007/s11270-015-2722-x
Alarcón, A., Davies, F. T., Autenrieth, R. L., and Zuberer, D. A. (2008). Arbuscular Mycorrhiza and Petroleum-Degrading Microorganisms Enhance Phytoremediation of Petroleum-Contaminated Soil. Int. J. Phytoremediation 10, 251–263. doi:10.1080/15226510802096002
Anwar-ul-Haq, M., Hashmat, A. J., Afzal, E. M., Mahmood, A., Ibrahim, M., Nawaz, M., et al. (2018). Pilot-Scale Electrochemical Treatment of Textile Effluent and its Toxicological Assessment for Tilapia (Oreochromis niloticus L.) Culture. Pak. J. Zool. 50 (5), 1703–17013. doi:10.17582/journal.pjz/2018.50.4.1703.1708
Aslam, A., Ibrahim, M., Mahmood, A., Mubashir, M., Sipra, H. F. K., Shahid, I., et al. (2021). Mitigation of Particulate Matters and Integrated Approach for Carbon Monoxide Remediation in an Urban Environment. J. Environ. Chem. Eng. 9 (4), 105546. doi:10.1016/j.jece.2021.105546
Aziz, N. S. B. A., Nor, M. A. B. M., Manaf, S. F. B. A., and Hamzah, F. (2015). Suitability of Biochar Produced from Biomass Waste as Soil Amendment. Proced.-Soc. Behav. Sci. 195, 2457–2465. doi:10.1016/j.sbspro.2015.06.288
Baker, J. M. (1970). The Effects of Oils on Plants. Environ. Pollut. (1970) 1, 27–44. doi:10.1016/0013-9327(70)90004-2
Bernier, H. M., Fine, P., and Borisover, M. (2013). Organic Matter Composition in Soils Irrigated with Treated Wastewater: FT-IR Spectroscopic Analysis of Bulk Soil Samples. Geoderma 209–210, 233–240. doi:10.1016/j.geoderma.2013.06.017
Callaghan, C. O., Blazquez, C., Downey, G., O’Donnell, D., and Howard, V. (2009). Prediction of Moisture, Fat and Inorganic Salts in Processed Cheese by Near Infrared Reflectance Spectroscopy and Multivariate Data Analysis. J. Near Infrared Spectroscopy 12 (3), 149–157.
Chang, W.-N., Liu, C.-W., and Liu, H.-S. (2009). Hydrophobic Cell Surface and Bioflocculation Behavior of Rhodococcus Erythropolis. Process Biochem. 44, 955–962. doi:10.1016/j.procbio.2009.04.014
Chauhan, R., Kumar, R., and Sharma, V. (2018). Soil Forensics: A Spectroscopic Examination of Trace Evidence. Microchemical J. 139, 74–84. doi:10.1016/j.microc.2018.02.020
Collins, C. D. (2007). “Implementing Phytoremediation of Petroleum Hydrocarbons,”. Methods in Biotechnology: Phytoremediation: Methods and Reviews, Part I. Editor N. Willey (New Jersey: Humana Press), 99-108. doi:10.1007/978-1-59745-098-0_8
Dickinson, N. (2017). “Phytoremediation,” in Encyclopedia of Applied Plant Sciences. 2nd Edn. (Oxford: Academic Press), 327–331. doi:10.1016/b978-0-12-394807-6.00016-2
Deschênes, M., Bourassa, J., Doan, V. D., and Parent, A. (1996). A Single-Cell Study of the Axonal Projections Arising from the Posterior Intralaminar Thalamic Nuclei in the Rat. Eur. J. Neurosci. 8, 329–343. doi:10.1111/j.1460-9568.1996.tb01217.x
Dietz, A. C., and Schnoor, J. L. (2001). Advances in Phytoremediation. Environ. Health Perspect. 109, 163–168. doi:10.1289/ehp.01109s1163
Ezeji, U. E., Anyadoh, S. O., and Ibekwe, V. I. (2007). Clean up of Crude Oil-Contaminated Soil. Terr. Aquat. Environ. Toxicol. 1 (2), 54–59.
Farag, S., Soliman, N. A., and Abdel-Fattah, Y. R. (2018). Statistical Optimization of Crude Oil Bio-Degradation by a Local marine Bacterium Isolate Pseudomonas Sp. Sp48. J. Genet. Eng. Biotechnol. 16, 409–420. doi:10.1016/j.jgeb.2018.01.001
Gaskin, S. E., and Bentham, R. H. (2010). Rhizoremediation of Hydrocarbon Contaminated Soil using Australian Native Grasses. Sci. Total Environ. 408, 3683–3688. doi:10.1016/j.scitotenv.2010.05.004
Juck, D., Charles, T., Whyte, L. G., and Greer, C. W. (2000). Polyphasic Microbial Community Analysis of Petroleum Hydrocarbon-Contaminated Soils from Two Northern Canadian Communities. Femsmicrobiol. Ecol. 33, 241–249. doi:10.1111/j.1574-6941.2000.tb00746.x
Kabata-Pendias, A. (2011). Trace Elements in Soils and Plants. 4th ed. Boca Raton, Florida: CRC Press, LLC.
Kanwal, U., Ibrahim, M., Abbas, F., Yamin, M., Jabeen, F., Shahzadi, A., Farooque, A. A., Imtiaz, M., Ditta, A., and Ali, S. (2021). Phytoextraction of Lead Using a Hedge Plant [Alternanthera Bettzickiana (Regel) G. Nicholson]: Physiological and Biochemical Alterations through Bioresource Management. Sustainability 13 (9), 5074. doi:10.3390/su13095074
Kanwal, U., Ibrahim, M., Ali, S., Adrees, M., Mahmood, A., Rizwan, M., and Muhammad, T. A. D. (2019). Potential of Alternanthera Bettzickiana (Regel) G. Nicholson for Remediation of Cadmium-Contaminated Soil Using Citric Acid. Pakjas 56, 753–759. doi:10.21162/pakjas/19.8181
Kassaye, G., Gabbiye, N., and Alemu, A. (2017). Phytoremediation of Chromium from Tannery Wastewater Using Local Plant Species. Water Pract. Technol. 12, 894–901. doi:10.2166/wpt.2017.094
Khan, S., Afzal, M., Iqbal, S., and Khan, Q. M. (2013). Plant-bacteria Partnerships for the Remediation of Hydrocarbon Contaminated Soils. Chemosphere 90, 1317–1332. doi:10.1016/j.chemosphere.2012.09.045
Kim, H. S., and Weber, W. J. (2003). Preferential Surfactant Utilization by a PAH-Degrading Strain: Effects on Micellar Solubilization Phenomena. Environ. Sci. Technol. 37 (3), 3574–3580. doi:10.1021/es0210493
Kirk, J. L., Klirnomos, J. N., Lee, H., and Trevors, J. T. (2002). Phytotoxicity Assay to Assess Plant Species for Phytoremediation of Petroleum-Contaminated Soil. Bioremediation J. 6, 57–63. doi:10.1080/10889860290777477
Kirk, J. L., Klironomos, J. N., Lee, H., and Trevors, J. T. (2005). The Effects of Perennial Ryegrass and Alfalfa on Microbial Abundance and Diversity in Petroleum Contaminated Soil. Environ. Pollut. 133, 455–465. doi:10.1016/j.envpol.2004.06.002
Ko, K.-S., Lee, P.-K., and Kong, I. C. (2012). Evaluation of the Toxic Effects of Arsenite, Chromate, Cadmium, and Copper Using a Battery of Four Bioassays. Appl. Microbiol. Biotechnol. 95, 1343–1350. doi:10.1007/s00253-011-3724-2
Kranner, I., and Colville, L. (2011). Metals and Seeds: Biochemical and Molecular Implications and Their Significance for Seed Germination. Environ. Exp. Bot. 72, 93–105. doi:10.1016/j.envexpbot.2010.05.005
Kumari, K., Tiwari, J., Sharma, P., and Bauddh, K. (2021). “Selection of Plant Species for Phytoremediation of Oil Drilling Sites: An Overview,” in Phytorestoration of Abandoned Mining and Oil Drilling Sites (New York: Elsevier), 455–472. doi:10.1016/b978-0-12-821200-4.00006-6
Kummerová, M., Zezulka, Š., Babula, P., and Váňová, L. (2013). Root Response in Pisumsativum and Zea mays under Fluoranthene Stress: Morphological and Anatomical Traits. Chemosphere 90, 665–673. doi:10.1016/j.chemosphere.2012.09.047
Liu, G., Zhong, H., Yang, X., Liu, Y., Shao, B., and Liu, Z. (2018). Advances in Applications of Rhamnolipids Biosurfactant in Environmental Remediation: A Review. Biotechnol. Bioeng. 115, 796–814. doi:10.1002/bit.26517
Ma, Y., Oliveira, R. S., Freitas, H., and Zhang, C. (2016). Biochemical and Molecular Mechanisms of Plant-Microbe-Metal Interactions: Relevance for Phytoremediation. Front. Plant Sci. 7, 918. doi:10.3389/fpls.2016.00918
Mahmood, A., Shahzad, T., Hussain, S., Ali, Q., Ali, H. M., Yasin, S., et al. (2021). Evaluation of Symbiotic Association between Various Rhizobia, Capable of Producing Plant-Growth-Promoting Biomolecules, and Mung Bean for Sustainable Production. Sustainability 13 (24), 13832. doi:10.3390/su132413832
Marchant, R., and Banat, I. M. (2012). Biosurfactants: a Sustainable Replacement for Chemical Surfactants? Biotechnol. Lett. 34 (9), 1597–1605. doi:10.1007/s10529-012-0956-x
Mehdi, S. E. H., Amen, R., Ali, A., Anjum, H., Mahmood, A., Mubashir, M., Mukhtar, A., Ullah, S., Al-Sehemi, A. G., Ibrahim, M., Khan, M. S., Qyyum, M. A., and Show, P. L. (2021). Sources, Chemistry, Bioremediation and Social Aspects of Arsenic-Contaminated Waters: a Review. Environ. Chem. Lett. 19, 3859–3886. doi:10.1007/s10311-021-01254-3
Mohanty, M., and Patra, H. K. (2011). Attenuation of Chromium Toxicity by Bioremediation Technology. Rev. Environ. Contam. Toxicol. 210, 1–34. doi:10.1007/978-1-4419-7615-4_1
Monalisa, M., and Patra, H. K. (2012). Phytoremediation Potential of Paragrass - an In Situ Approach for Chromium Contaminated Soil. Int. J. Phytoremediation 14, 796–805. doi:10.1080/15226514.2011.619595
Mukherjee, A. K., and Bordoloi, N. K. (2011). Bioremediation and Reclamation of Soil Contaminated with Petroleum Oil Hydrocarbons by Exogenously Seeded Bacterial Consortium: a Pilot-Scale Study. Environ. Sci. Pollut. Res. 18, 471–478. doi:10.1007/s11356-010-0391-2
Müller, H., Westendorf, C., Leitner, E., Chernin, L., Riedel, K., Schmidt, S., et al. (2009). Quorum-sensing Effects in the Antagonistic Rhizosphere Bacterium Serratia Plymuthica HRO-C48. Fems Microbiol. Ecol. 67, 468–478. doi:10.1111/j.1574-6941.2008.00635.x
Müller, H., and Berg, G. (2008). Impact of Formulation Procedures on the Effect of the Biocontrol Agent Serratia Plymuthica HRO-C48 on Verticillium Wilt in Oilseed Rape. BioControl 53, 905e916.
Njoku, K. L., Akinola, M. O., and Oboh, B. O. (2008). Does Crude Oil Affect the pH, Moisture Andorganic Content of Soils? Ecol. Environ. Conservation Paper 14 (04), 731–736.
Patowary, K., Patowary, R., Kalita, M. C., and Deka, S. (2016). Development of an Efficient Bacterial Consortium for the Potential Remediation of Hydrocarbons from Contaminated Sites. Front. Microbiol. 7, 1092. doi:10.3389/fmicb.2016.01092
Peralta-Videa, J. R., Gardea-Torresdey, J. L., Gomez, E., Tiemann, K. J., Parsons, J. G., De la Rosa, G., et al. (2002). Potential of Alfalfa Plant to Phytoremediate Individually Contaminated Montmorillonite-Soils with Cadmium(II), Chromium(VI), Copper (II), Nickel(II), and Zinc(II). Bull. Environ. Contam. Toxicol. 69 (1), 74–81. doi:10.1007/s00128-002-0012-y
Qin, X., Liu, Y., Huang, Q., Liu, Y., Zhao, L., and Xu, Y. (2019). In-situ Remediation of Cadmium and Atrazine Contaminated Acid Red Soil of South China Using Sepiolite and Biochar. Bull. Environ. Contam. Toxicol. 102, 128–133. doi:10.1007/s00128-018-2494-2
Roy, D., Liu, M., and Wang, G. t. (1994). Modeling of Anthracene Removal from Soil Columns by Surfactant. J. Environ. Sci. Health . A: Environ. Sci. Eng. Toxicol. 29, 197–213. doi:10.1080/10934529409376029
Salanitro, J. P., Dorn, P. B., Huesemann, M. H., Moore, K. O., Rhodes, I. A., Rice Jackson, L. M., et al. (1997). Crude Oil Hydrocarbon Bioremediation and Soil Ecotoxicity Assessment. Environ. Sci. Technol. 31, 1769–1776. doi:10.1021/es960793i
Samuel, O., Christian, S., and Frederick, O. (2018). Effect of Pig Manure on the Microbial Remediation of Crude Oil-Polluted Soil. Am. J. Life Sci. Res. 6 (2), 76–90.
Schaff, S. D., Pezeshki, S. R., and Shields, F. D. (2002). Effects of Pre-planting Soaking on Growth and Survival of Black Willow Cuttings. Restor Ecol. 10, 267–274. doi:10.1046/j.1526-100x.2002.02035.x
Song, H.-G., and Bartha, R. (1990). Effects of Jet Fuel Spills on the Microbial Community of Soil. Appl. Environ. Microbiol. 56, 646–651. doi:10.1128/aem.56.3.646-651.1990
Stelmack, J., Szlyk, J., Stelmack, T., Babcock-Parziale, J., Demers-Turco, P., Williams, R. T., and Massof, R. W. (1999). Use of Rasch Person-Item Map in Exploratory Data Analysis: A Clinical Perspective. J. Rehabil. Res. Dev. 41, 233–242. doi:10.1682/jrrd.2004.02.0233
Sun, B. P., Zhao, B., Han, C., Wang, J., Mo, D., and Zhang, S. (2018). Lab Study on the Effect of Cation Exchange Capacity on Slurry Performance in Slurry Shields. Hindawi Adv. Civil Eng. 2018, 9. doi:10.1155/2018/2942576
Sung, K., Kim, K. S., and Park, S. (2013). Enhancing Degradation of Total Petroleum Hydrocarbons and Uptake of Heavy Metals in a Wetland Microcosm Planted withPhragmites Communisby Humic Acids Addition. Int. J. Phytoremediation 15, 536–549. doi:10.1080/15226514.2012.723057
Tang, W.-C., White, J. C., and Alexander, M. (1998). Utilization of Sorbed Compounds by Microorganisms Specifically Isolated for that Purpose. Appl. Microbiol. Biotechnol. 49, 117–121. doi:10.1007/s002530051147
Van Hamme, J. D., and Ward, O. P. (2001). Physical and Metabolic Interactions of Pseudomonas Sp. Strain JA5-B45 and Rhodococcus Sp. Strain F9-D79 during Growth on Crude Oil and Effect of a Chemical Surfactant on Them. Appl. Environ. Microbiol. 67 (10), 4874–4879. doi:10.1128/aem.67.10.4874-4879.2001
Villegas, J., and Fortin, J. A. (2001). Phosphorus Solubilization and pH Changes as a Result of the Interactions between Soil Bacteria and Arbuscular Mycorrhizal Fungi on a Medium Containing NH4+as Nitrogen Source. Can. J. Bot. 79, 865–870. doi:10.1139/b01-069
Wang, Q., Zhang, S., Li, Y., and Klassen, W. (2011). Potential Approaches to Improving Biodegradation of Hydrocarbons for Bioremediation of Crude Oil Pollution. Jep 02, 47–55. doi:10.4236/jep.2011.21005
Ward, O., Singh, A., and Van Hamme, J. (2003). Accelerated Biodegradation of Petroleum Hydrocarbon Waste. J. Ind. Microbiol. Biotechnol. 30 (5), 260–270. doi:10.1007/s10295-003-0042-4
Wiltse, C. C., Rooney, W. L., Chen, Z., Schwab, A. P., and Banks, M. K. (1998). Greenhouse Evaluation of Agronomic and Crude Oil‐Phytoremediation Potential Among Alfalfa Genotypes. J. Environ. Qual. 27, 169–173. doi:10.2134/jeq1998.00472425002700010024x
Xing, Z., Du, C., Tian, K., Ma, F., Shen, Y., and Zhou, J. (2016). Application of FTIR-PAS and Raman Spectroscopies for the Determination of Organic Matter in Farmland Soils. Talanta 1580, 262–269. doi:10.1016/j.talanta.2016.05.076
Yang, J., Zhang, L., Fukuzaki, Y., Hira, D., and Furukawa, K. (2010). High-rate Nitrogen Removal by the Anammox Process with a Sufficient Inorganic Carbon Source. Bioresour. Technology 101, 9471–9478. doi:10.1016/j.biortech.2010.07.087
Zahed, M. A., Aziz, H. A., Isa, M. H., and Mohajeri, L. (2010). Enhancement Biodegradation of N-Alkanes from Crude Oil Contaminated Seawater. Int. J. Environ. Res. 4, 655–664.
Keywords: Triton X-100, phytoremediation, crude oil-contaminated soil, bacterial consortium, Brachiaria mutica, FTIR
Citation: Anwar-ul-Haq M, Ibrahim M, Yousaf B, Al-Huqail AA and Ali HM (2022) Role of Bacterial Consortium and Synthetic Surfactants in Promoting the Phytoremediation of Crude Oil-Contaminated Soil Using Brachiaria mutica. Front. Energy Res. 10:874492. doi: 10.3389/fenrg.2022.874492
Received: 12 February 2022; Accepted: 24 March 2022;
Published: 27 April 2022.
Edited by:
Abdul-Sattar Nizami, Government College University, Lahore, PakistanReviewed by:
Farhat Abbas, University of Prince Edward Island, CanadaMuhammad Imtiaz, Huazhong Agricultural University, China
Ahmad Mukhtar, Washington State University, United States
Abdul Jabbar Chaudhary, Brunel University London, United Kingdom
Copyright © 2022 Anwar-ul-Haq, Ibrahim, Yousaf, Al-Huqail and Ali. This is an open-access article distributed under the terms of the Creative Commons Attribution License (CC BY). The use, distribution or reproduction in other forums is permitted, provided the original author(s) and the copyright owner(s) are credited and that the original publication in this journal is cited, in accordance with accepted academic practice. No use, distribution or reproduction is permitted which does not comply with these terms.
*Correspondence: Muhammad Ibrahim, ZWJyYWhlbS5tQGdtYWlsLmNvbQ==