- 1School of Mechanical and Electric Engineering, Guangzhou University, Guangzhou, China
- 2Guangzhou Institute of Energy Conversion, Chinese Academy of Sciences, Guangzhou, China
- 3Dalian National Laboratory for Clean Energy, Dalian, China
- 4Department of Energy and Mechanical Engineering, Hunan Institute of Humanities, Science and Technology, Loudi, China
- 5University of Chinese Academy of Sciences, Beijing, China
The combustion characteristics of laminar non-premixed CH4 jet flame in an O2/CO2 coflows with different oxygen mole fractions were studied experimentally. The flame heights at different oxygen concentrations and fuel jet velocity were obtained. The experimental observation shows that the luminosity of the CH4 jet flame in O2/CO2 coflow is different from that of the flame in air stream. A two-dimensional numerical study of a laminar non-premixed CH4 jet flame in the O2/CO2 coflow with the O2 mole fraction of 0.35 was conducted to analyze the effects of CO2 dilution on the flame. The distribution of OH radicals in the flame was measured experimentally using planar laser-induced fluorescence (PLIF) to validate the computational method adopted in this work, and the computational and experimental results of the OH distributions showed good consistency at various fuel flow velocities. Three artificial species were created in the numerical experiment to analyze the effects of the chemical reactions, third-body collisions, and transport properties of CO2 on the height, width, and temperature distribution of the flame. The results showed that CO2 participation in chemical reactions exerts significant effects on the flame. However, the influences of the third-body effects and transport properties of CO2 on the jet flame are unremarkable. The global reaction pathways and distributions of important species in the laminar non-premixed CH4 jet flame were analyzed in detail to investigate the influence mechanisms of CO2 on the flame height and temperature. The entire flame can be divided into two oxidation parts, which separated by the boundary of the HCCO. The H, O, and OH concentrations and distributions in different parts of the flame were influenced by CO2 dilution, resulting in different flame heights and temperature distributions.
Introduction
The reduction of CO2 emissions during fossil fuel utilization is an important task for human society. The injection of purified CO2 from flue gas into underground reservoirs is believed to help achieve this goal (Buhre et al., 2005; Wall, 2007). However, CO2-rich flue gas is required to increase the efficiency and economy during carbon capture and storage. Several new advanced combustion technologies that use O2/CO2 as an oxidizer, such as oxy-fuel combustion technology (Buhre et al., 2005; Wall, 2007; Hjärtstam et al., 2009; Rathnam et al., 2009; Scheffknecht et al., 2011; Taniguchi et al., 2011; Chen et al., 2012; Dhaneswar and Pisupati, 2012; Luo et al., 2015; Moroń and Rybak, 2015; Liu et al., 2016; Ge et al., 2017; Seddighi, 2017; Menage et al., 2018; Zhang et al., 2018), moderate or intense low-oxygen dilution (MILD) oxy-combustion (Li et al., 2013; Tu et al., 2015; Mardani and FazlollahiGhomshi, 2016; Mao et al., 2017; Gładysz et al., 2018), gaseous fuel-fired oxy-fuel combustion technology (Yin et al., 2011; Seepana and Jayanti, 2012a; Seepana and Jayanti, 2012b; Oh et al., 2013a; Oh et al., 2013b; Oh and Noh, 2013; Oh and Noh, 2014; Giménez-López et al., 2015; Oh and Noh, 2015; Oh and Hong, 2016; Bürkle et al., 2018), and high-temperature oxygen combustion technology (Li et al., 2014; Li et al., 2015; Li et al., 2016), have been developed to meet this requirement. However, many other challenges must be solved before these combustion technologies can be widely adopted in the industry, since the differences in the physical properties and chemical characteristics of CO2 and N2 can lead to important distinctions in flame structure. More information on the combustion characteristics of different fuels in various O2/CO2 environments must be obtained for the development of new combustion approaches.
Experimental and numerical studies on the fundamental combustion characteristics of O2/CO2-fired flames have been conducted previously. The effect of CO2 on the free-propagation speed of laminar premixed CH4/O2/CO2 flames was investigated numerically (Liu et al., 2003). The speeds of laminar CH4/O2/CO2 flames at different equivalence ratios and pressures were measured and computed (Xie et al., 2013). The CH4 oxidation under CO2-rich conditions has also been investigated using an atmospheric pressure flow reactor (Glarborg and Bentzen, 2008). The effects of environmental pressure (Maruta et al., 2007) and oxidizer temperature (Li et al., 2014) on the stretch extinction characteristics of CH4/CO2 versus O2/CO2 counterflow non-premixed flames have been studied through experiments and numerical computations. The ignition temperature of a stoichiometric CH4/O2/CO2 mixture was examined by using a micro flow reactor with a controlled temperature profile (Li et al., 2015). The fundamental studies (Liu et al., 2003; Maruta et al., 2007; Glarborg and Bentzen, 2008; Xie et al., 2013; Li et al., 2014; Li et al., 2015; Li et al., 2016) show that CO2 exerts an inhibitory effect on flames. Consequently, a higher oxygen concentration is recommended for the O2/CO2-fired flames to achieve a comparable combustion characteristics as the air flames.
The previous fundamental studies have provided an essential understanding of flames in O2/CO2 environments (Liu et al., 2003; Maruta et al., 2007; Glarborg and Bentzen, 2008; Xie et al., 2013; Li et al., 2014; Li et al., 2015; Li et al., 2016). However, the three-dimensional structures of flames in O2/CO2, which are important for the development of industrial burner, deserves more attention. The combustion characteristics of turbulent premixed CH4/air/CO2 and CO/H2/CO2/O2 flames was investigated experimentally (Kobayashi et al., 2007; Kobayashi et al., 2009; Wang et al., 2013). And it was observed that local wrinkled structures become sharp and propagate deeply into the burned mixture with addition of CO2. It was also proposed that CO2 addition is effective for restraining combustion oscillation (Kobayashi et al., 2007). Experimental studies of the swirl-stabilized turbulent CH4/air and CH4/O2/CO2 flames show that the average length of air flames is longer than that of oxy-flames. Moreover, it was found that one-dimensional laminar flame properties could not be used to explain the intense burning of turbulent oxy-flames (Watanabe et al., 2016).
Recently, the influences of CO2 on the combustion characteristics of multidimensional flames has been studied in detail. The physical and chemical effects of CO2 dilution on a CH4/H2 jet flame in the MILD oxy-combustion regime have been investigated using two-dimensional numerical computations with a detailed kinetic mechanism (Tu et al., 2016; Tu et al., 2017). It was found that the chemical effects of CO2 play a comparable role in the suppression of temperature rise to the physical effects (Tu et al., 2016). The influences of CO2 dilution on the shape and structure of laminar CO/H2 diffusion flames in an O2/N2/CO2 coflow was examined (Xu et al., 2017), and the chemical effects of the thermal and transport properties of CO2 on the flames were discussed in detail. The previous numerical studies provide important information on the effects of CO2 under MILD (Tu et al., 2016; Tu et al., 2017) and syngas (Xu et al., 2017) combustion regimes. However, the effects of CO2 on the flame shape and temperature distribution of gaseous-fuel-fired flames in an O2/CO2 environment with a high O2 concentration needs special attention and was not clarified.
The target of the present work is to study on the combustion characteristics of laminar non-premixed methane jet flames in oxygen/carbon dioxide coflows. The laminar flame heights at different oxygen concentrations were measured by experiments. The OH distributions of typical laminar methane jet flames in a O2/CO2 coflow were measured by OH-PLIF technique. Two-dimensional numerical computations with a detailed kinetic mechanism were conducted, and three artificial species modified from CO2 were employed in the numerical experiment to clarify the effects of CO2. The influences through the chemical reactions, third-body collisions, and transport properties of CO2 on the combustion characteristics of a laminar non-premixed CH4 jet flame in an O2/CO2 environment with a high O2 concentration was distinguished in detail.
Experiment
Experimental Setup and Method
Figure 1 shows a schematic diagram of the experimental system. The jet-flame experimental apparatus includes three parts, namely, a quartz tube, a fuel tube, and a stainless-steel chamber. The length and height of the rectangular quartz tube are 10 and 60 cm, respectively, and the wall thickness is 4 mm. The fuel stream was supplied through a stainless-steel tube with an inner diameter (d) of 1 mm and a length of 15 cm. The fuel tube was installed at the center of a rectangular quartz tube. The rectangular quartz tube and the stainless-steel chamber were connected together, and a ceramic honeycomb (diameter, 12 cm; height, 10 cm) and ceramic beads (diameter, 2 mm) were arranged in the stainless-steel chamber to achieve a uniform coflow velocity. A well-mixed O2/CO2 stream was fed into the rectangular quartz tube from the bottom of the chamber. Three MKS digital mass flow controllers were employed to control the volumetric flow rates of gases, and a wet gas meter (Shinagawa, W-NK-2) was used to calibrate the digital mass flow controllers. The rectangular quartz tube was adopted in the experiment because it is convenient for optical measurements. In addition, the similarity of non-premixed laminar CH4 jet flames in a circular tube with an inner diameter of 9.2 cm to those in a rectangular quartz tube has been confirmed by preliminary experiment. The laminar CH4 jet flames in O2/CO2 colfows with different oxygen mole fractions (XO2 = 0.30, 0.35 and 0.40) were tested by the present experimental system.
A single-lens reflex digital camera (Nikon D-610, f/4) was fixed in front of the flame to record the flame images. The ISO and exposure time were set as 1,250 and 1/40 s, respectively. The qualitative distribution of OH radicals in laminar non-premixed CH4 jet flames in an O2/CO2 coflow was measured using the OH-PLIF technique. The experimental apparatus was fixed on a lifter with a scale which can adjust the position of the flame. Consequently, the OH distribution of the different parts of the flame can be measured. The distribution of OH radicals at the center plane of the flame was measured by the OH-PLIF system. A beam with a wavelength of 355 nm was provided by a Nd:YAG laser (Quanta-Ray Pro-230). This beam was then transformed into a new laser beam by a dye laser (Sirah PSCAN-G-30) to excite the OH radicals in the flame. The wavelength of the dye laser beam was around 283.565 nm for the present OH-PLIF system. And the height of the laser sheet was about 40 mm and the thickness was less than 100 μm at the location of the flame. An ICCD camera (LAVISION VC-IRO and VC-Imager Pro X 4M) with an OH filter was used to obtain the OH image of the flame. The finest pixel resolution of the ICCD camera at the flame position was approximately 50 µm. A detailed description of the OH-PLIF system and the selected wavelength is provided elsewhere (Li et al., 2017). Raw OH-PLIF images were directly used for qualitative comparison with the numerical results because the measured OH intensity is proportional to the computational OH molar concentration within a 10% error (Yamamoto et al., 2009). The OH-PLIF results at each fuel flow velocity were averaged from every 100 OH-PLIF images. As the length of the laser sheet at the location of the flame was approximately 40 mm, which cannot cover the overall scope of the flame, the OH distributions in different parts of the flame were measured by adjusting the height of the experimental apparatus. The overall OH distribution of a jet flame was assembled from the OH distributions at different parts of the flame.
Experimental Results
The flame height and width which are determined by oxygen concentration and fuel flow rate are important parameter for the design of the industrial burner. Consequently, the laminar CH4 jet flames in O2/CO2 coflows with oxygen mole fractions (XO2) of 0.30, 0.35 and 0.40 were studied experimentally. The coflow velocity was kept at 0.1 m/s for different cases. The laminar CH4 jet flames in the air coflow were also tested for the comparison. The images of CH4 laminar jet flames in the O2/CO2 coflow (XO2 = 0.35) with different fuel jet velocities are shown in Figure 2. It can be seen that the flame height of the laminar CH4 jet flame increase linearly with the fuel velocity when the O2/CO2 is used as the oxidizer.
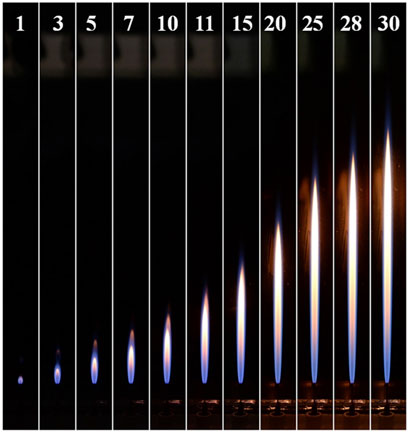
FIGURE 2. The images of CH4-jet-flames in O2/CO2 coflows with an oxygen mole fraction of 0.35. (The number on the top denotes the corresponding fuel flow velocity in the unit of m/s).
The experimental results in Figures 2, 3 show that the luminosity of the flame in the O2/CO2 coflow is different from that of the laminar CH4 jet flame in air. Both the laminar CH4 jet flames in the air and O2/CO2 coflows can be divided into two sections, bottom and top partitions, based on the flame luminosity. The bottom section of the flame is dominated by the blue color luminosity, while the top partition of flame shows orange and yellow colors for the flame in O2/CO2 and air respectively. It can be seen that the bottom section of the flame with O2/CO2 coflow show dark blue color, however, the flame in air is more bright. The luminosities of the laminar CH4 jet flames in O2/CO2 and air coflows suggests that the effect of CO2 on the flame structure is remarkable, which needs further investigation.
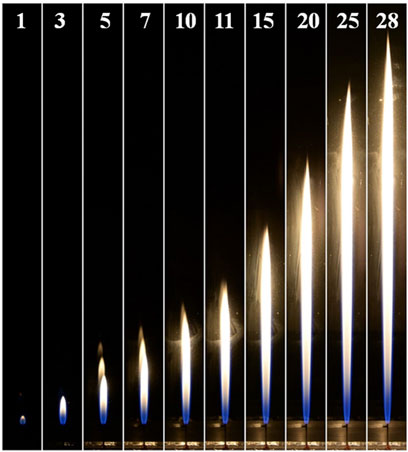
FIGURE 3. The images of CH4-jet-flames in air coflow. (The number on the top denotes the corresponding fuel flow velocity in the unit of m/s).
The flame height of laminar CH4 jet flames in O2/CO2 (XO2 = 0.30, 0.35 and 0.40) and air coflows were measured, as shown in Figure 4. It can be seen that the flame height is smoothly decreased as the increase of oxygen mole fraction. The flame in air is taller than those of the flames in O2/CO2 coflows. The theoretical study on the flame length of non-premixed laminar jet flame was conducted in the early studies (Roper, 1977; Roper et al., 1977), and the formula of the flame length for circular port burner was obtained and shown as follows,
Where T0, TF and Tf are the ambient temperature, the fuel flow temperature and the average temperature of the flame respectively. Q is the volumetric flow rate of fuel stream, S is the stoichiometric molar ratio of oxidizer to fuel. D0 is the diffusion coefficient at T0. The increase of oxygen concertation leads a decrease of the stoichiometric molar ratio of oxidizer to fuel and an increase of the flame temperature. Consequently the laminar flame height in the O2/CO2 coflow with a higher oxygen concentration is lower.
Numerical Computation and Analysis
Physical Model and Computation Method
Numerical computation with a detailed reaction mechanism was conducted to clarify the effects of CO2 on the combustion characteristics of the laminar CH4 jet flame in O2/CO2 colfow. Figure 5 illustrates the physical model and boundary conditions. A CH4 stream was injected into the O2/CO2 coflow through a tube with an inner diameter (d) of 1 mm and a length (L) of 15 cm. The length (L) and width (D/2) of the computation domain are 50 and 4.6 cm, respectively. An O2/CO2 mixture with an O2 mole fraction (XO) of 0.35 was used in the study, since the previous work has shown that the counterflow non-premixed CH4 flame in an O2/CO2 coflow with XO = 0.35 has a combustion intensity comparable with that of an air flame (Maruta et al., 2007; Li et al., 2014). The pressure in the system was 1 atm, and the inlet temperature of the oxidizer and fuel streams was 300 K. Laminar non-premixed CH4 jet flames with two different fuel jet velocities (VF = 5 and 10 m/s, Re = 293 and 586) were investigated. The inlet flow velocity of the oxidizer stream was fixed at 0.1 m/s. The laminar non-premixed jet flame was simplified to a two-dimensional axisymmetric swirl model. All walls were considered to be in a no-slip state. A convective heat transfer boundary (ambient temperature, 300 K; convective heat transfer coefficient, 6 W/m2·K) based on the experimental conditions was used for the wall of the computational domain, and an adiabatic wall condition was applied to the fuel tube wall. While the velocity inlet boundary was used at the fuel and oxidizer inlets, the pressure outlet boundary was applied at the outlet.
The governing equations included the mass, momentum, energy, and species-conservation equations, as well as the ideal gas equation of state. A detailed description of the governing equations can be found elsewhere (Li et al., 2017). The governing equations were discretized based on the finite-volume method, and the open-source framework, OpenFOAM (OpenFOAM, 2016) was employed to conduct the numerical study. The SIMPLE algorithm was used. The finite rate chemistry model and the GRI-Mech 3.0 mechanism (Smith et al., 2020) were also used to compute the chemical reactions. Previous studies on CO2-diluted flames (Liu et al., 2003; Maruta et al., 2007; Xie et al., 2013; Li et al., 2014; Li et al., 2015) have shown satisfactory performance of the mechanism. The diffusion coefficient was calculated using the Maxwell–Stefan equations. The thermal diffusion effect was also included in the calculations. Mesh independence was confirmed by preliminary numerical computations. Grids with 136320 cells were used, and the finest grid size was 45 µm. The values of 1.0 × 10–6 and 1.0 × 10–8 were used as convergence criteria for the mass and energy conservation equations and chemical reactions, respectively. Radiation heat transfer from the gases was not included in the computation, since the present work primarily focuses on comparisons of the effects of the chemical reactions, third-body collisions, and transport properties of CO2. A discussion on the radiation of CO2 on non-premixed flames can be found elsewhere (Maruta et al., 2007).
Additional computations using three artificial species modified from CO2 were conducted to analyze the effects of the chemical reactions, third-body reactions, and transport properties of CO2 on the combustion characteristics of the laminar non-premixed jet flame. The properties of the artificial species are listed in Table 1. The first artificial species is KCO2, which does not participate in chemical reactions but participates in third-body collisions and has thermal and transport properties identical to those of CO2. The second artificial species is XCO2, which has the same thermal and transport properties as CO2 but does not participate in chemical reactions and third-body collisions. The third artificial species is DCO2, which has the same chemical reactions, third-body effects, and thermal properties as CO2 but transport properties (i.e., thermal conductivities, viscosities, diffusion coefficients, and thermal diffusion coefficients) identical to those of N2. The participation or not of CO2 in the chemical reactions can lead to differences in the computational results between the CO2 and KCO2 conditions. Differences in the combustion characteristics between KCO2 and XCO2 can be attributed to the third-body effects of CO2. The effects of the transport properties of CO2 on the jet flame can be attributed to differences in the computational results between the DCO2 and CO2 cases.
Validation of the Computational Method
The computational OH distributions of laminar non-premixed CH4 jet flames at VF = 5 and 10 m/s were compared with the experimental results, as shown in Figure 6. The total OH distributions in the flames at fuel jet velocities of 5 and 10 m/s consist of three and five parts, respectively. It can be seen that the shape and height determined from the computational OH distributions are consistent with the results obtained from the experiment. The small difference between the computational and experimental OH distributions may be attributed to two reasons: the laser sheet is not perfectly set at the center of the flame and is slightly inclined and the computational method assumes a two-dimensional physical model and neglects radiation heat loss. Despite minor differences can be observed between the measured and computational OH distributions, the comparison of the overall results indicates that the present computational method and kinetic mechanism are reasonable for the study of the effects of CO2 on the combustion characteristics of laminar non-premixed CH4 jet flames in an O2/CO2 coflow with a high O2 concentration.
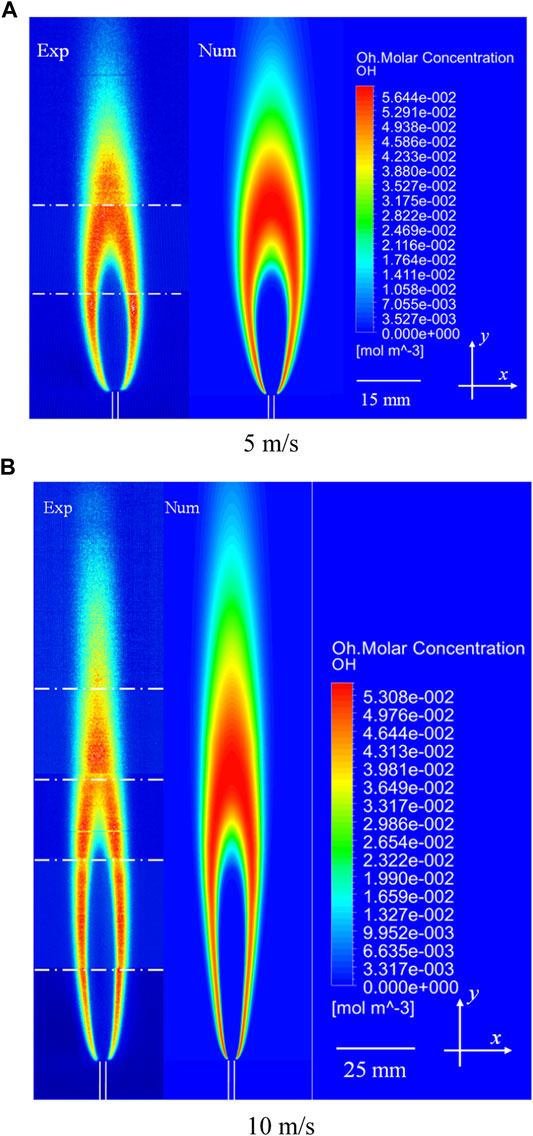
FIGURE 6. Measured and computated OH distributions of laminar non-premixed CH4 jet flames in an O2/CO2 coflow at fuel jet velocities of 5 and 10 m/s. (A) 5 m/s. (B) 10 m/s.
Effects of CO2 on Flame Temperature and Shape
The computational results of jet flames in O2/CO2, O2/KCO2, O2/XCO2, and O2/DCO2 coflows were obtained and compared. Figures 7, 8 respectively show the temperature and OH distributions in laminar non-premixed jet flames at VF = 10 m/s. The flames in the O2/CO2 and O2/DCO2 coflows are nearly identical, and the flames in O2/KCO2 and O2/XCO2 coflows are almost the same. Comparison of flames in the O2/KCO2 and O2/XCO2 coflows indicates that the contribution of the third-body effects of CO2 on the shape and temperature distribution of the laminar jet flame is insignificant. The results of flames in the O2/CO2 and O2/DCO2 coflows reveal that the difference in transport properties between CO2 and N2 also does not lead to a significant change in the flames. However, the difference between the flames in the O2/CO2 and O2/KCO2 coflows suggests that the flame shape and temperature distribution of laminar non-premixed jet flames are significantly changed by the chemical effects of CO2. Specifically, the flame height is decreased, whereas the maximum flame width is increased, when the chemical effects of CO2 are excluded from consideration. The decrease in the maximum flame temperature by the chemical effect of CO2 is approximately 230 K.
The detailed computational results of laminar non-premixed CH4 jet flames in O2/CO2, O2/KCO2, O2/XCO2, and O2/DCO2 coflows at a fuel flow velocities of 5 and 10 m/s were analyzed. The flame heights and widths based on the OH distribution in flames in different oxidizers at VF = 5 and 10 m/s are illustrated in Figures 9A,B, respectively. The flame boundaries are judged by 99% decrease of the maximum OH molar concentration. The variations in the flame height and width of laminar non-premixed CH4 jet flames at VF = 5 m/s in different oxidizer coflows are similar to those of flames at VF = 10 m/s. Although not shown here, the numerical results indicate that the maximum flame temperatures at VF = 5 m/s in O2/CO2 and O2/DCO2 are nearly identical, i.e., 2470 K, and the maximum flame temperatures in O2/KCO2 and O2/XCO2 are similar, i.e., approximately 2670 K. The computational OH distributions show that flame height decreases, whereas flame width increases, when the chemical effect of CO2 is suspended.
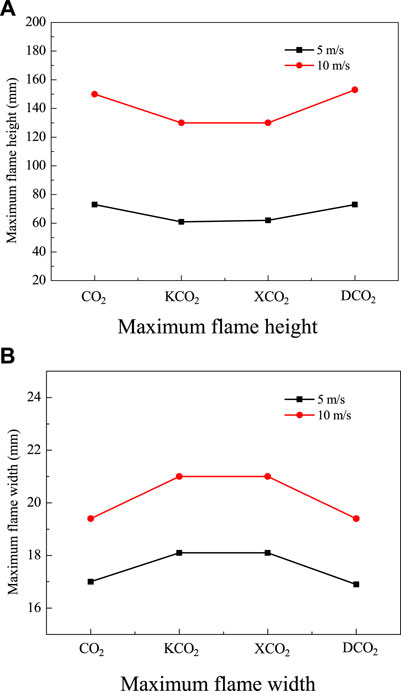
FIGURE 9. Computational flame height and width based on OH distributions in laminar non-premixed CH4 jet flames in different oxidizers. (A) Maximum flame height. (B) Maximum flame width.
Mechanism of CO2 on the Laminar Non-Premixed CH4 Jet Flames
The reaction pathways and flame structures in O2/CO2, O2/KCO2, O2/XCO2 and O2/DCO2 were analyzed to interpret the mechanism of the chemical effect of CO2 on laminar non-premixed CH4 jet flames. The primary reaction pathways of flames in the O2/KCO2, O2/XCO2, and O2/DCO2 coflows are nearly identical to those of flames in the O2/CO2 coflow. Figure 10 shows the global reaction pathways of laminar non-premixed flames in the O2/CO2 coflow at VF = 10 m/s. CH4 is transformed into CH3 through H abstraction by H, O, and OH. Most of the CH3 radicals are transformed into C2H5, CH2(S), and C2H6, and only a small amount of the CH3 radicals react with O atoms to produce CH2O. Most of the C2H6 generated is transformed into C2H5 through third-body reactions. C2H5 radicals decompose rapidly into C2H4, and H abstraction by H and OH, which are important sources of C2H4 consumption, produces C2H3 radicals. The reaction C2H3(+M) = C2H2 + H (+M) consumes C2H3 radicals to produce C2H2. Most of the C2H2 is consumed by reaction with O to produce HCCO. The HCCO radicals generate CH and CO via the third-body reaction HCCO(+M) = CH + CO(+M). Finally, CO is oxidized to CO2 by the reaction OH + CO = H + CO2.
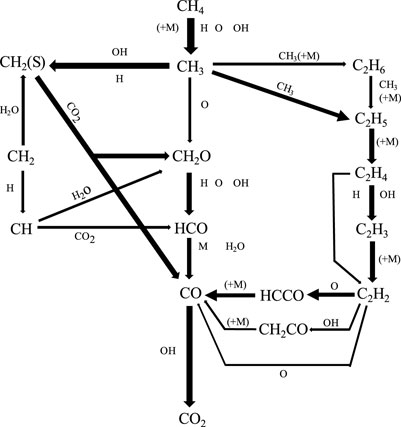
FIGURE 10. Integral reaction flow in a laminar non-premixed CH4 jet flame in an O2/CO2 coflow at VF = 10 m/s (thick lines show the major reactions).
Figure 11 shows the distribution of important species in a laminar non-premixed jet flame in an O2/CO2 environment at VF = 10 m/s. The CH4-rich region can be observed near the exit of the tube, and the CH3-rich region can be observed around the region with a high CH4 concentration. The C2H2-rich concentration region is located at nearly the same area as the CH3-rich region. The HCCO is found on the margins of the C2H2-rich region, and theHCO only exists at the two sides of the CH3-rich region. The molar concentration of the HCCO is higher than that of the HCO. The CO-rich region is located downstream of the HCCO-rich region.
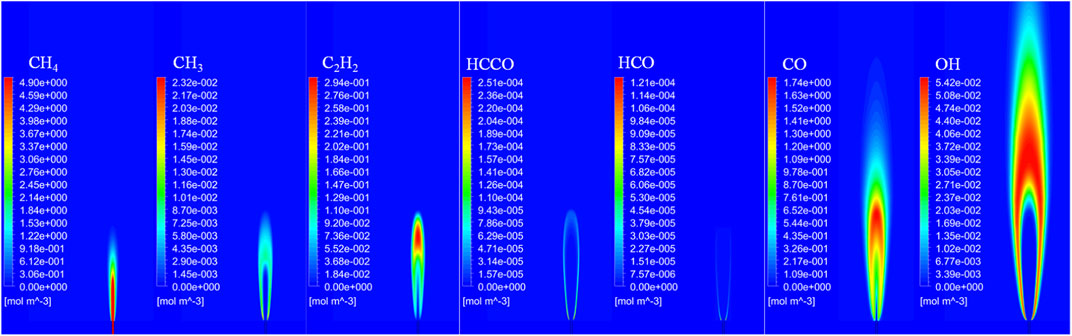
FIGURE 11. Molar concentrations of important species in a laminar non-premixed CH4 jet flame in an O2/CO2 coflow (VF = 10 m/s).
The analysis of the computational results of flames in the O2/KCO2, O2/XCO2, and O2/DCO2 coflows shows that the distributions of important species are similar to those of flames in O2/CO2. Moreover, laminar non-premixed CH4 jet flames in different oxidizers can be divided into two parts based on the global oxidation process. The results of flames in the O2/CO2 coflow at VF = 10 m/s are illustrated in Figure 12; here, the white dotted line indicates the boundary between the first and second parts of the flame. The first part of the flame includes the oxidation process from CH4 to CO through the reaction pathway CH4 → CH3 → C2H6 → C2H5 → C2H4 → C2H3 → C2H2 → HCCO → CO, while the primary reaction in the second part is the oxidation of CO to CO2. Consequently, the top of the HCCO region is used as the boundary separating the first and second parts of the flame. Generation of H2 and H2O is mainly completed in the first part of the flame. The distribution of the heats of reaction indicates that the first and second parts of the flame make remarkable contributions to heat generation. A distinct endothermic region is located in the first part of the flame. The effects of CO2 on the first and second typical parts of non-premixed laminar jet flames result in different combustion characteristics.
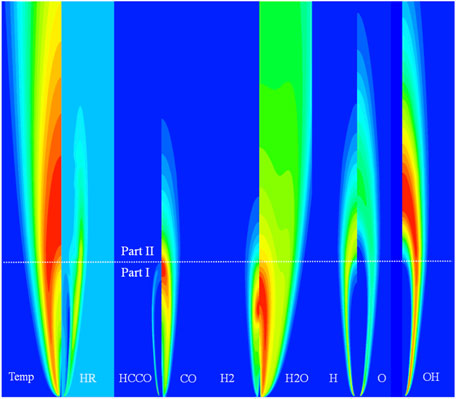
FIGURE 12. The structure of the laminar non-premixed CH4 jet flame (VF = 10 m/s) in an O2/CO2 coflow. (Temp: temperature; HR: heat of reaction).
The distributions of important species of flames in the O2/CO2 and O2/KCO2 coflows were compared to enable interpretation of the chemical effect of CO2 on the laminar non-premixed CH4 jet flame, since the effect of third-body collisions with CO2 on the laminar non-premixed jet flame has been confirmed to be negligible. The distributions of the molar concentrations of HCCO, CO, O, and H in the flames in O2/CO2 and O2/KCO2 coflows are shown in Figures 13A–D. The heights of the HCCO-, CO-, O-, and H-rich regions in the flame in the O2/KCO2 coflow are shorter than those in the flame in the O2/CO2 coflow. Interestingly, the difference between the heights of the HCCO-rich region in the flames in the O2/CO2 and O2/KCO2 coflows is nearly identical to that between the heights of the OH-rich regions (as shown in Figure 8). The difference between the flame heights based on the OH distribution is related to differences in HCCO distribution in the first part of the flame. The analysis of the reaction pathways shows that HCCO is primarily produced by the reaction O+ C2H2 = H + HCCO. As O atoms are mainly generated by the reaction H + O2 = O+ OH, the flame height essentially depends on the H distribution. The contours of the H molar concentration shown in Figure 13D indicate that the concentration of H atoms of the flame in O2/KCO2 is significantly higher than that of the flame in O2/CO2. Pathway analysis of the entire flame region shows that the reaction OH + CO = H + CO2 is an important source of H atoms. This finding suggests that the chemical effect of CO2 on H formation and distribution ultimately leads to a difference in flame heights.
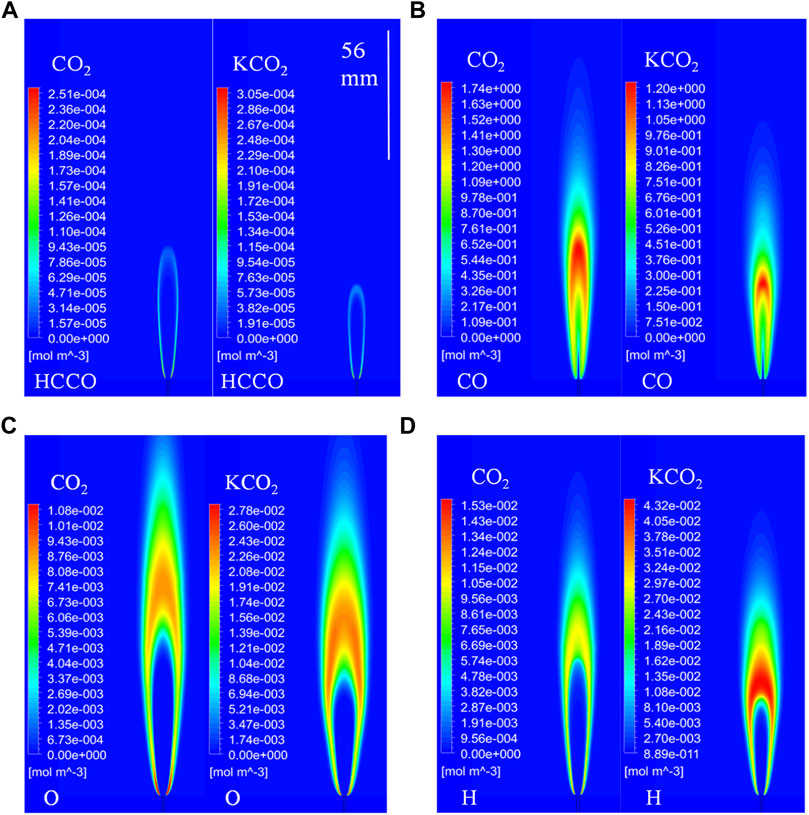
FIGURE 13. Distributions of the molar concentration of important species in flames in O2/CO2 and O2/KCO2: (A) HCCO, (B) CO, (C) O, and (D) H.
The results shown in Figure 12 indicate that the high-temperature region and the OH-rich region are coincident, this finding can also be observed from the results shown in Figures 7, 8. This phenomena is attributed to the fact that the high-temperature region is located in the second part of the laminar non-premixed flame, where the reaction OH + CO = H + CO2 is the primary exothermic reaction. The results shown in Figure 7 indicate that the maximum temperature of the laminar non-premixed flame in the O2/CO2 coflow is approximately 230 K lower than that of the flame in the O2/KCO2 coflow. Although not shown here, analysis of the computational results shows that the net reaction rates of OH + CO = H + CO2 and H + O2 = O + OH of the flame in the O2/CO2 coflow are lower than those of the flame in the O2/KCO2 coflow. The net reaction rate of OH + CO = H + CO2 in the second part of the flame is significantly reduced by the chemical effect of CO2, which results in a low flame temperature.
Conclusion
The heights of the laminar CH4 jet flames in O2/CO2 coflows with different oxygen mole fractions of 0.30, 0.35 and 0.40 were obtained by experimental study. The height of the flame in O2/CO2 coflow decreases as the increase of oxygen mole fraction. And the height of the laminar CH4 jet flame in O2/CO2 coflow with oxygen mole fraction of 0.30 is shorter than that of the laminar CH4 jet flame in air. Moreover, the luminosity of the CH4 jet flame in O2/CO2 coflow is totally different from that of the flame in air stream.
The chemical reactions, third-body effects, and transport properties of CO2 on the combustion characteristics of a laminar non-premixed CH4 jet flame in an O2/CO2 coflow with a high O2 concentration (XO = 0.35) were investigated using a two-dimensional numerical computational method with a detailed kinetic mechanism. The computational OH distributions showed good consistency with the results obtained from the experiments. Whereas the third-body effect and transport properties of CO2 do not exert remarkable effects on the laminar non-premixed flame, the chemical effect of CO2 on the laminar non-premixed jet flame is significant. The chemical effect of CO2 increases the flame height but decreases the maximum flame temperature. The primary oxidation pathway in the jet flame is CH4 → CH3 → C2H6 → C2H5 → C2H4 → C2H3 → C2H2 → HCCO → CO → CO2. The CH4 non-premixed laminar jet flame can be divided into two parts based on the global oxidization process; here, the top of the HCCO-rich region is the boundary between these parts. The decrease in the concentration of O atoms by the chemical effect of CO2 in the first part of the flame can explain the observed decrease in flame height, and the inhibitory effect of CO2 on the reaction rate of OH + CO = H + CO2 in the second part of the flame induces a decrease in maximum flame temperature.
Data Availability Statement
The original contributions presented in the study are included in the article/supplementary material, further inquiries can be directed to the corresponding author.
Author Contributions
FZ: Laser diagnostic, Data analysis, Reviewing and editing. XL: Conceptualization, Supervision, Methodology, Investigation, Reviewing and editing, Funding acquisition. SX: Data Curation, Reviewing and editing. JW: Data Curation, Reviewing and editing. XW: Reviewing and editing.
Funding
The supports of National Natural Science Foundation of China (No. 52176139) and the DNL Cooperation Fund, CAS (DNL202006) are gratefully acknowledged.
Conflict of Interest
The authors declare that the research was conducted in the absence of any commercial or financial relationships that could be construed as a potential conflict of interest.
Publisher’s Note
All claims expressed in this article are solely those of the authors and do not necessarily represent those of their affiliated organizations, or those of the publisher, the editors, and the reviewers. Any product that may be evaluated in this article, or claim that may be made by its manufacturer, is not guaranteed or endorsed by the publisher.
References
Buhre, B. J. P., Elliott, L. K., Sheng, C. D., Gupta, R. P., and Wall, T. F. (2005). Oxy-fuel Combustion Technology for Coal-Fired Power Generation. Prog. Energ. Combustion Sci. 31, 283–307. doi:10.1016/j.pecs.2005.07.001
Bürkle, S., Dreizler, A., Ebert, V., and Wagner, S. (2018). Experimental Comparison of a 2D Laminar Diffusion Flame under Oxy-Fuel and Air Atmosphere. Fuel 212, 302–308. doi:10.1016/j.fuel.2017.10.067
Chen, L., Yong, S. Z., and Ghoniem, A. F. (2012). Oxy-fuel Combustion of Pulverized Coal: Characterization, Fundamentals, Stabilization and CFD Modeling. Prog. Energ. Combustion Sci. 38, 156–214. doi:10.1016/j.pecs.2011.09.003
Dhaneswar, S. R., and Pisupati, S. V. (2012). Oxy-fuel Combustion: The Effect of Coal Rank and the Role of char-CO2 Reaction. Fuel Process. Technol. 102, 156–165. doi:10.1016/j.fuproc.2012.04.029
Ge, X., Dong, J., Fan, H., Zhang, Z., Shang, X., Hu, X., et al. (2017). Numerical Investigation of Oxy-Fuel Combustion in 700°C-Ultra-Supercritical Boiler. Fuel 207, 602–614. doi:10.1016/j.fuel.2017.06.119
Giménez-López, J., Millera, A., Bilbao, R., and Alzueta, M. U. (2015). Experimental and Kinetic Modeling Study of the Oxy-Fuel Oxidation of Natural Gas, CH4 and C2H6. Fuel 160, 404–412. doi:10.1016/j.fuel.2015.07.087
Gładysz, P., Stanek, W., Czarnowska, L., Sładek, S., and Szlęk, A. (2018). Thermo-ecological Evaluation of an Integrated MILD Oxy-Fuel Combustion Power Plant with CO2 Capture, Utilisation, and Storage – A Case Study in Poland. Energy 144, 379–392. doi:10.1016/j.energy.2017.11.133
Glarborg, P., and Bentzen, L. L. B. (2008). Chemical Effects of a High CO2 Concentration in Oxy-Fuel Combustion of Methane. Energy Fuels 22, 291–296. doi:10.1021/ef7005854
Hjärtstam, S., Andersson, K., Johnsson, F., and Leckner, B. (2009). Combustion Characteristics of lignite-fired Oxy-Fuel Flames. Fuel 88, 2216–2224. doi:10.1016/j.fuel.2009.05.011
Kobayashi, H., Hagiwara, H., Kaneko, H., and Ogami, Y. (2007). Effects of CO2 Dilution on Turbulent Premixed Flames at High Pressure and High Temperature. Proc. Combust. Inst. 31, 1451–1458. doi:10.1016/j.proci.2006.07.159
Kobayashi, H., Yata, S., Ichikawa, Y., and Ogami, Y. (2009). Dilution Effects of Superheated Water Vapor on Turbulent Premixed Flames at High Pressure and High Temperature. Proc. Combust. Inst. 32, 2607–2614. doi:10.1016/j.proci.2008.05.078
Li, P., Dally, B. B., Mi, J., and Wang, F. (2013). MILD Oxy-Combustion of Gaseous Fuels in a Laboratory-Scale Furnace. Combust. Flame 160, 933–946. doi:10.1016/j.combustflame.2013.01.024
Li, X., Jia, L., Nakamura, H., Tezuka, T., Hasegawa, S., and Maruta, K. (2015). Study on Flame Responses and Ignition Characteristics of CH4/O2/CO2 Mixture in a Micro Flow Reactor with a Controlled Temperature Profile. Appl. Therm. Eng. 84, 360–367. doi:10.1016/j.applthermaleng.2015.03.022
Li, X., Jia, L., Onishi, T., Grajetzki, P., Nakamura, H., Tezuka, T., et al. (2014). Study on Stretch Extinction Limits of CH4/CO2 versus High Temperature O2/CO2 Counterflow Non-premixed Flames. Combust. Flame 161, 1526–1536. doi:10.1016/j.combustflame.2013.12.004
Li, X., Yang, H., Jiang, L., Wang, X., and Zhao, D. (2016). Stretch Extinction Characteristics of CH4/CO2 versus O2/H2O/CO2 and O2/H2O Counterflow Non-premixed Flames at Different Oxidizer Temperatures. Fuel 186, 648–655. doi:10.1016/j.fuel.2016.09.017
Li, X., Zhang, J., Yang, H., Jiang, L., Wang, X., and Zhao, D. (2017). Combustion Characteristics of Non-premixed Methane Micro-jet Flame in Coflow Air and thermal Interaction between Flame and Micro Tube. Appl. Therm. Eng. 112, 296–303. doi:10.1016/j.applthermaleng.2016.10.082
Liu, D., Wall, T., and Stanger, R. (2016). CO2 Quality Control in Oxy-Fuel Technology for CCS: SO2 Removal by the Caustic Scrubber in Callide Oxy-Fuel Project. Int. J. Greenhouse Gas Control. 51, 207–217. doi:10.1016/j.ijggc.2016.05.026
Liu, F., Guo, H., and Smallwood, G. J. (2003). The Chemical Effect of CO2 Replacement of N2 in Air on the Burning Velocity of CH4 and H2 Premixed Flames. Combust. Flame 133, 495–497. doi:10.1016/s0010-2180(03)00019-1
Luo, W., Wang, Q., Huang, X., Liu, Z., and Zheng, C. (2015). Dynamic Simulation and Transient Analysis of a 3MWth Oxy-Fuel Combustion System. Int. J. Greenhouse Gas Control. 35, 138–149. doi:10.1016/j.ijggc.2015.02.003
Mao, Z., Zhang, L., Zhu, X., and Zheng, C. (2017). Experiment Investigation of Coal MILD-Oxy Combustion Integrated with Flue Gas Recirculation at a 0.3MWth Furnace. Fuel Process. Technol. 162, 126–134. doi:10.1016/j.fuproc.2017.04.002
Mardani, A., and Fazlollahi Ghomshi, A. (2016). Numerical Study of Oxy-Fuel MILD (Moderate or Intense Low-Oxygen Dilution Combustion) Combustion for CH4–H2 Fuel. Energy 99, 136–151. doi:10.1016/j.energy.2016.01.016
Maruta, K., Abe, K., Hasegawa, S., Maruyama, S., and Sato, J. (2007). Extinction Characteristics of CH4/CO2 versus O2/CO2 Counterflow Non-premixed Flames at Elevated Pressures up to 0.7MPa. Proc. Combust. Inst. 31, 1223–1230. doi:10.1016/j.proci.2006.08.013
Menage, D., Lemaire, R., and Seers, P. (2018). Experimental Study and Chemical Reactor Network Modeling of the High Heating Rate Devolatilization and Oxidation of Pulverized Bituminous Coals under Air, Oxygen-Enriched Combustion (OEC) and Oxy-Fuel Combustion (OFC). Fuel Process. Technol. 177, 179–193. doi:10.1016/j.fuproc.2018.04.025
Moroń, W., and Rybak, W. (2015). Ignition Behaviour and Flame Stability of Different Ranks Coals in Oxy Fuel Atmosphere. Fuel 161, 174–181. doi:10.1016/j.fuel.2015.08.065
Oh, J., and Hong, S. (2016). Oxygen Temperature Variation of a Non-premixed Oxy-Methane Flame in a Lab-Scale Slot Burner. Appl. Therm. Eng. 104, 804–817. doi:10.1016/j.applthermaleng.2016.05.112
Oh, J., and Noh, D. (2015). Flame Characteristics of a Non-premixed Oxy-Fuel Jet in a Lab-Scale Furnace. Energy 81, 328–343. doi:10.1016/j.energy.2014.12.046
Oh, J., Noh, D., and Ko, C. (2013). The Effect of Hydrogen Addition on the Flame Behavior of a Non-premixed Oxy-Methane Jet in a Lab-Scale Furnace. Energy 62, 362–369. doi:10.1016/j.energy.2013.09.049
Oh, J., Noh, D., and Lee, E. (2013). The Effect of CO Addition on the Flame Behavior of a Non-premixed Oxy-Methane Jet in a Lab-Scale Furnace. Appl. Energ. 112, 350–357. doi:10.1016/j.apenergy.2013.06.033
Oh, J., and Noh, D. (2013). Lifted Flame Behavior of a Non-premixed Oxy-Methane Jet in a Lab-Scale Slot Burner. Fuel 103, 862–868. doi:10.1016/j.fuel.2012.09.055
Oh, J., and Noh, D. (2014). The Effect of CO2 Addition on the Flame Behavior of a Non-premixed Oxy-Methane Jet in a Lab-Scale Furnace. Fuel 117, 79–86. doi:10.1016/j.fuel.2013.08.065
OpenFOAM The Open Source CFD Toolbox. 2016 Available at: http://www.openfoam.org.
Rathnam, R. K., Elliott, L. K., Wall, T. F., Liu, Y., and Moghtaderi, B. (2009). Differences in Reactivity of Pulverised Coal in Air (O2/N2) and Oxy-Fuel (O2/CO2) Conditions. Fuel Process. Technol. 90, 797–802. doi:10.1016/j.fuproc.2009.02.009
Roper, F. G., Smith, C., and Cunningham, A. C. (1977). The Prediction of Laminar Jet Diffusion Flame Sizes: Part II. Experimental Verification. Combust. Flame 29, 227–234. doi:10.1016/0010-2180(77)90113-4
Roper, F. G. (1977). The Prediction of Laminar Jet Diffusion Flame Sizes: Part I.Theoretical Model. Combust. Flame. 29, 219–226. doi:10.1016/0010-2180(77)90112-2
Scheffknecht, G., Al-Makhadmeh, L., Schnell, U., and Maier, J. (2011). Oxy-fuel Coal Combustion-A Review of the Current State-Of-The-Art. Int. J. Greenhouse Gas Control. 5, S16–S35. doi:10.1016/j.ijggc.2011.05.020
Seddighi, S. (2017). Design of Large Scale Oxy-Fuel Fluidized Bed Boilers: Constant thermal Power and Constant Furnace Size Scenarios. Energy 118, 1286–1294. doi:10.1016/j.energy.2016.11.004
Seepana, S., and Jayanti, S. (2012). Experimental Studies of Flame Extinction in a Swirl-Stabilized Oxy-Fuel Burner. Fuel 93, 75–81. doi:10.1016/j.fuel.2011.10.065
Seepana, S., and Jayanti, S. (2012). Flame Structure Investigations of Oxy-Fuel Combustion. Fuel 93, 52–58. doi:10.1016/j.fuel.2011.07.033
Smith, G. P., Golden, D. M., Frenklach, M., Moriarty, N. W., Eiteneer, B., Goldenberg, M., et al. 2020 Berkeley Mechanical Engineering. Available at: http://www.me.berkeley.edu/gri_mech/.
Taniguchi, M., Yamamoto, K., Okazaki, T., Rehfeldt, S., and Kuhr, C. (2011). Application of Lean Flammability Limit Study and Large Eddy Simulation to Burner Development for an Oxy-Fuel Combustion System. Int. J. Greenhouse Gas Control. 5, S111–S119. doi:10.1016/j.ijggc.2011.05.008
Tu, Y., Liu, H., Chen, S., Liu, Z., Zhao, H., and Zheng, C. (2015). Numerical Study of Combustion Characteristics for Pulverized Coal under Oxy-MILD Operation. Fuel Process. Technol. 135, 80–90. doi:10.1016/j.fuproc.2014.10.025
Tu, Y., Liu, H., and Yang, W. (2017). Flame Characteristics of CH4/H2 on a Jet-In-Hot-Coflow Burner Diluted by N2, CO2, and H2O. Energy Fuels 31, 3270–3280. doi:10.1021/acs.energyfuels.6b03246
Tu, Y., Su, K., Liu, H., Chen, S., Liu, Z., and Zheng, C. (2016). Physical and Chemical Effects of CO2 Addition on CH4/H2 Flames on a Jet in Hot Coflow (JHC) Burner. Energy Fuels 30, 1390–1399. doi:10.1021/acs.energyfuels.5b02499
Wall, T. F. (2007). Combustion Processes for Carbon Capture. Proc. Combustion Inst. 31, 31–47. doi:10.1016/j.proci.2006.08.123
Wang, J., Matsuno, F., Okuyama, M., Ogami, Y., Kobayashi, H., and Huang, Z. (2013). Flame Front Characteristics of Turbulent Premixed Flames Diluted with CO2 and H2O at High Pressure and High Temperature. Proc. Combust. Inst. 34, 1429–1436. doi:10.1016/j.proci.2012.06.154
Watanabe, H., Shanbhogue, S. J., Taamallah, S., Chakroun, N. W., and Ghoniem, A. F. (2016). The Structure of Swirl-Stabilized Turbulent Premixed CH4/air and CH4/O2/CO2 Flames and Mechanisms of Intense Burning of Oxy-Flames. Combust. Flame. 174, 111–119. doi:10.1016/j.combustflame.2016.09.015
Xie, Y., Wang, J., Zhang, M., Gong, J., Jin, W., and Huang, Z. (2013). Experimental and Numerical Study on Laminar Flame Characteristics of Methane Oxy-Fuel Mixtures Highly Diluted with CO2. Energy Fuels 27, 6231–6237. doi:10.1021/ef401220h
Xu, H., Liu, F., Sun, S., Zhao, Y., Meng, S., and Tang, W. (2017). Effects of H2O and CO2 Diluted Oxidizer on the Structure and Shape of Laminar Coflow Syngas Diffusion Flames. Combust. Flame 177, 67–78. doi:10.1016/j.combustflame.2016.12.001
Yamamoto, K., Ozeki, M., Hayashi, N., and Yamashita, H. (2009). Burning Velocity and OH Concentration in Premixed Combustion. Proc. Combust. Inst. 32, 1227–1235. doi:10.1016/j.proci.2008.06.077
Yin, C., Rosendahl, L. A., and Kær, S. K. (2011). Chemistry and Radiation in Oxy-Fuel Combustion: A Computational Fluid Dynamics Modeling Study. Fuel 90, 2519–2529. doi:10.1016/j.fuel.2011.03.023
Keywords: non-premixed laminar jet flame, O2/CO2 coflow, chemical effect, third-body effect, transport property
Citation: Zhang F, Li X, Xie S, Wang J and Wang X (2022) Experimental and Numerical Study on the Combustion Characteristics of a Laminar Non-Premixed Methane Jet Flame in Oxygen/Carbon Dioxide Coflow. Front. Energy Res. 10:847947. doi: 10.3389/fenrg.2022.847947
Received: 03 January 2022; Accepted: 24 January 2022;
Published: 17 February 2022.
Edited by:
Zhihua Wang, Zhejiang University, ChinaReviewed by:
Yu Wang, Wuhan University of Technology, ChinaLei Zhou, Harbin Institute of Technology, Shenzhen, China
Jinhua Wang, Xi’an Jiaotong University, China
Copyright © 2022 Zhang, Li, Xie, Wang and Wang. This is an open-access article distributed under the terms of the Creative Commons Attribution License (CC BY). The use, distribution or reproduction in other forums is permitted, provided the original author(s) and the copyright owner(s) are credited and that the original publication in this journal is cited, in accordance with accepted academic practice. No use, distribution or reproduction is permitted which does not comply with these terms.
*Correspondence: Xing Li, lixing@ms.giec.ac.cn, inspirationlee@hotmail.com